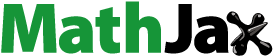
Abstract
Sediment with varying sizes is transported through runoff, finding ways into sewerage pipes and deposited at low-velocity sections. As wastewater is high in organic content, biofilm formed on the deposited sediment, cementing the deposition and resulting in difficulties in the removal from pipes. High concentration of oil and grease in domestic wastewater promotes strengthening the cementing process of the deposited sediment. The presence of silt in the sediment distribution promotes physical cohesion, whereas biofilm through Extracellular Polymeric Substances (EPS) provides microbial cohesion. Thus, this study attempts to investigate the incipient sediment motion of sediment mixture with a different fraction of fine sand-silt, presence of biofilm through variation of EPS concentration, 0–0.1% and percentage of oil and grease (O&G) at 0–10%. The analysis showed that physical cohesion is more critical than microbial cohesion, with the presence of O&G posing the least significant on the changes of incipient sediment motion.
1. Introduction
Sewerage pipes collect and convey domestic wastewater from residential areas to a Sewage Treatment Plant for a mandatory treatment process before discharge into waterways. The fundamental design of the sewage network involves not only the pipe diameter and materials but also the settlement of solids. The system is designed with a minimum velocity, also known as “self-cleansing velocity,” to prevent the deposition of transported materials, including sediment. Accumulation of deposited sediment in the sewage lines may be up to 10 mm in depth [Citation1,Citation2] and comprises non-homogenous sediment, with varying distribution from fine sand to gravel [Citation3]. Deposited sediment in the sewer pipes reduced effective area consequentially and the production of poisonous or hazardous gaseous substances [Citation4].
The naturally high organic content in sewage promotes and accelerates the biofilm formation on the wall of pipes and the deposited sediment, shown in Figure . Extracellular Polymeric Substances (EPS) secreted by the bacterial community (within the biofilm) strengthen the physicochemical interactions, particularly for fine-size sediment, increasing the sediment stability [Citation5]. Biostabilisation from the formation of biofilm on sediment protects the sediment bed from being entrained (and thus eroded) [Citation6], requiring high self-cleansing velocity than what was calculated based on a non-stabilised sediment bed. Biological activity must be considered for a long-term assessment of sediment behaviour [Citation7]. According to Vignaga et al. [Citation8], microorganism attachment to river sediments via EPS may result in the creation of an elastic organic biofilm layer on the sediment bed, increasing sediment erosion resistance. Their results showed that the film generated on the sediment surface by biological activity was the most important component influencing sediment incipient motion, and they created a model for assessing sediment incipient motion. In another study, Malarkey et al. [Citation7] demonstrated that the biologically cohesive EPS have a greater impact on incipient sediment motion than the physically cohesive EPS.
Figure 1. The formation of biofilm on deposited sediment and the presence of oil and grease in the wastewater sewer pipes. The EPS secreted by microbes on the fine sand provides stability and influences the transport and cleaning processes.

Most of the existing sediment transport behaviour was described mostly based on non-cohesive sediment, whereas studies on the influence of physical cohesion have also been picked up [Citation9,Citation10]. Additional binding of biological cohesion (through EPS) significantly changes the bedform development compared to the abiotic-influenced systems and suggests a reformulation of cohesive biological effects on the sediment transport models [Citation7,Citation11,Citation12]. Higher hydrodynamic forces are needed to initiate erosion of the bio-sedimentary beds, particularly for beds aged more than 5 days [Citation13,Citation14]. Hypothetically, shear stress is needed to break the protective biofilm layer before being able to move (and transport) sediment, commonly resulting in a higher stress of up to two orders of magnitude. Biofilm consists of mat-like biofilm and filamentous elements penetrating the sediment bed, adding adhesive forces and enhancing sediment stabilization [Citation15]. Not only that, the biofilm coating the sediment surface changes the turbulence characteristics at the interfacial sublayer [Citation16]. Bed shear stress imposed on the biofilm-coated sediment bed is much smaller compared to those without biofilm [Citation17].
Unmaintained grease traps, high consumption of fast foods and booming food service establishments promote a high concentration of Fat, Oil and Grease (FOG) in the wastewater [Citation18,Citation19]. The formation of FOG deposits is promoted in conditions of low pH, concrete pipes and in the presence of unsaturated Free Fatty Acids (FFA) [Citation20]. The deposition of FOG on the sewer liners has long been reported as the leading cause of sewer blockages, resulting in numerous cases of Sanitary Sewer Overflows (SSO) [Citation21,Citation22].
Sediment movements are influenced by hydrodynamic and electrochemical forces and also biological processes [Citation23,Citation24]. The concept of initiation sediment motion was first introduced by Shields [Citation25] and described using homogeneous non-cohesive sediment. Movement of sediment is when the hydrodynamic forces impinging on the particle grain exceed the resistance from the submerged weight [Citation26]. Although the description based on the well-established Shields diagram (or the derived empirical equation) may represent an approximation for a mixture of sediment, the influence of physical and biological cohesions proved to be higher than what was predicted by Mantz [Citation27]. A considerable increase in erosion threshold was observed for sand mixture with more than 5% fractions of mud [Citation28]. The presence of clay at > 5% changes the structural framework from previously sandy-type to the clay mineral framework giving more of a cohesive sediment behaviour [Citation28,Citation29]. Furthermore, a high concentration of FOG not only accelerates the pipe clogging but also is expected to have significant impacts by further cementing the existing deposited sediment bed.
The threshold criteria of sediment motion are commonly described using the critical Shields parameter :
(1)
(1)
where
is the critical shear velocity, the symbol
is
, which
is the sediment density,
is the density of water,
is the gravitational acceleration, and
is the median grain size of sediment size.
The concept of incipient sediment motion is critical in sediment transport models, in particular characterizing bed load and suspended load movement and rates of erosion and deposition. In this research, we present an experimental approach to explore the incipient sediment motion with organic content and oil and grease. For this aim, different sand-silt mixtures as 100/0, 90/10, 80/20, 70/30 and 60/40, four different EPS concentrations as 0%, 0.01%, 0.07%, and 0.1% and two percentages of oil and grease at 5% and 10% are examined. The effect of each additional material as sand-silt, EPS and oil-grease percentages on incipient sediment motion is statistically investigated. To the best of our knowledge, the influence of a mixture of EPS and FOG on incipient sediment motion for different sand-silt percentages is yet to be discussed in detail, giving robust laboratory data to enhance the understanding of mixed sediment transport models.
2. Materials and methods
2.1. Experimental setup
The erosion chamber used in this study, shown in Figure , helps in determining the relationship between the dimensionless shear stress produced on the surface of the sediment sample and the corresponding revolutions per minute of the propeller. The method allows for a variation in propeller speed (and hence the velocity) systematically through the dial. In this test, a single-size bed was created and used by putting together many sand samples of the same size. The stirrer motor was then switched on, whereby the speed of the propeller (and hence, the angular velocity) was increased stepwise (using the dial) until the particles were observed to start moving continuously. In this study, the definition described by Kramer [Citation30] of general movement was used. Once the state of incipient motion was established, the reading of the propeller was recorded (as rpm) using a tachometer. At this point, the velocity is deemed as critical velocity, whereby the rotation of the propeller (in rpm) is made analogous to the critical velocity calculated based on van Rijn’s [Citation31] description. The procedure was conducted using 8 sizes of homogenous sediment from fine to coarse sediment, i.e. 106, 300, 450, 600, 850, 1180, 2360, and 4750 µm. As such, we obtained a representation of propeller speed (measured as rotation per minute, rpm) and critical velocity as
. Using this equation, the associated critical stress (and hence critical velocity, too) may be obtained based on the tachometer readings. This approach is a more efficient experimental setup for incipient sediment motion, reducing the requirement of the high volume of water (as needed in a pipe/flume), and allows for a quicker repetition of measurement.
Three experimental procedures were carried out to achieve the objectives of the study. Preparation of the sediment mixture is first started by homogenously mixing the fine sand and silt before adding the EPS to emulate the cohesivity imposed by the biofilm. The last step involves the addition of oil and grease at 5% and 10%, whereby the percentage of O&G applied here falls within the typical range of O&G in wastewater [Citation32]. The sand-silt mixture with different percentages of fine sand and kaolinite is investigated, and their physical properties are presented in Table , giving a total of 60 samples. Xanthan gum (C35H49O29), a commonly used food additive, is adapted as EPS as previously conducted in the earlier laboratory tests [Citation5,Citation33]. This easily accessible and safe food additive is selected as it provides not only similar characteristics to the natural biofilm colonization [Citation34] but is also able to control the level of biostabilisation. The utilization of extracted EPS reduces both preparation and experimental times, which often takes up to 5–30 days [Citation15].
Table 1. Sediment physical properties.
The sediment mixture consisting of sand with a size of 300 and 28 µm size of kaolin was prepared through a wet mixing procedure at a temperature of 25°C, using tap water pH 7 with 25% v/v (to achieve the plastic limit). Five mixtures of sand-silt were prepared with varying percentages of sand/silt as 100/0, 90/10, 80/20, 70/30 and 60/40 for different concentrations of EPS. The sand is put first in a bucket before mixing it with the kaolin to ensure a homogeneous dry mixture. Water is added in stages and thoroughly mixed using a spatula. The bucket is covered to minimize contamination and left for 18 h. For sediment mixture with EPS, the xanthan gum is thoroughly mixed using 1% of water (giving 24% of water needs to be added to the mixture) before being added to the sand-silt mixture. The mixture is carefully transferred into the erosion chamber bottom container and levelled with a scraper, giving a consistent level of the bed. The bottom container is then installed at its place in the erosion chamber. The water is then filled in at a slow rate to minimize the disruption to the levelled bed before filling it to the 30 cm depth. The blade is switched on, initially at dial 0, before systematically increasing it until a threshold sediment motion is observed. The criterion for incipient sediment motion is taken as when the movement of sediment is observed at multiple locations, following the Kramer description. The blade rotation was obtained using a calibrated tachometer, where the critical value for each sample is taken as the averaged rotation from 10 readings. Once the critical value is recorded, the water is drained to allow the bottom chamber to be taken out.
The biostabilisation effect was investigated by introducing four different concentrations of EPS, i.e. at 0% representing physical cohesive sediment, 0.01% representing low primary production rates, 0.07% and 0.1% to imitate an environment with high primary production rates [Citation35]. The range of EPS applied here is comparable with the ranges obtained for sandy mud or sand with low mud content [Citation7]. Although the surficial biofilm can have a concentration of EPS up to 5% and 1% in the intertidal muds and freshwater muds, respectively, the lower concentration of EPS (<0.1%) is pervasively distributed [Citation35,Citation36] and played a dominant role [Citation7].
To prepare the sand-silt-EPS mixture, the key aspect is the EPS needs to be homogeneously mixed. In achieving the plastic limit within the range of 23.1 to 34.9, 25% (of the dry sediment weight) volume of water was added to the sediment mixture. A volume of 1% water was used to mix the EPS powder (in a separate container). The prepared EPS was slowly poured into an electric mixer with the remaining 24% water and thoroughly mixed until the homogeneity in the sand-silt-EPS mixture was achieved. The mixture was left (at room temperature) to be consolidated for 18 h before starting the experiments. This is to enhance the reproduction of organic matter in the mixture with the assistance of EPS. After the consolidation hours have been reached (usually the following day), the sediment is transferred into the erosion chamber, and the surface is made flat using a horizontal scraper. The water slowly flowed into the erosion chamber by controlling the discharge valve to avoid any disturbance on the flatbed. The water level was then gradually increased, reaching a height of 30 cm from the base of the erosion chamber.
We also expand the analysis to investigate the influence of oil and grease, the growing concern pollutants present in the wastewater [Citation18]. Using the same sediment sand-silt-EPS mixture, oil and grease (OG) of 5% and 10% v/v of sand were added to the container where the sediment-EPS mixture was remixed with the O&G to ensure homogeneity. The samples were left for 4 h to imitate the average of low flow hours. Note that we do not focus on the FOG deposits due to saponification but more on the immediate effect of OG on the deposited sediment in the sewer. The now sand-silt-EPS-OG was placed in the base of the erosion chamber. The characteristics of samples used for experiments, with varying sediment distribution, percentage of EPS and oil and grease, are presented in Table . A similar procedure to determine the threshold criteria of sediment motion as described above was conducted. This procedure is to determine the effect of oil and grease on the incipient motion of physical and biological cohesive sediment.
Table 2. The characteristics of samples, with varying fractions of fine sand-silt, percentages of EPS and O&G.
2.2. Statistical analysis
To explore the possible effect of independent variables, i.e. sediment mixture (denote after as Ratio, R), percentage of EPS (E), and oil and grease (denote as OG, O) on the dependent variable, incipient motion , the three-way Analysis of Variance (ANOVA) are conducted. Technically, the incipient motion,
was measured at a continuous level and the mean of
was independent between the groups of categorical variables, namely EPS, Ratio and the Oil and Grease levels.
Let R takes values i at 1,2,3,4,5; E takes values j at 1,2,3,4, and O takes values k at 1,2,3. Then, the notation of observations for the mean of at a different level of R, E and O can be summarized as a three-way contingency table, as shown in Table with 2 replicates. The table is a cross-classification of observations by the levels of the three categorical variables. The R, E and O for each observation in a sample of size n = 120 can be arranged in the vector of cell counts in the table with their
, respectively.
Table 3. The matrix of critical Shields parameter for each parameter.
Then, the three-way ANOVA model is stated as shown in (2) if the combination of all levels may be considered.
(2)
(2)
where
is the interaction effect of levels
and
;
is the interaction effect of levels
and
,
is the interaction effect of levels
and
, and
is the interaction effect of all levels. In this case, either level
,
, or (and)
may be changed due to the combination.
In this study, interaction effects determine the combined effects of EPS, Ratio and OG on the incipient sediment motion, . When an interaction impact is significant, the impact of OG depends on the level of Ratio and EPS. Thus, ANOVA is a good statistical method to be used due to its ability to assess and test the interaction effects. For preliminary analysis, three-way interaction can be visualized by plotting the two-way interactions (
separately by the level of the third variable (
. All analyses were done in Minitab, SAS and R. Post-hoc analysis of Fisher Least Significant Difference (LSD) was then performed on the data to investigate the statistically different parameters.
3. Results and discussion
The results of critical Shields parameter provide the baseline scenario of changes in threshold criteria from the non-cohesive sediment, whereby this will be first presented. The statistical analysis of one, two- and three-way ANOVA is discussed following this prior detailed discussion on the influence of Ratio, EPS and OG based on the LSD tests.
3.1. Influence of physical cohesion on the threshold criteria of sediment motion
It is critical to discuss the influence of silt in the sediment mixture as the baseline with the heterogeneity of sediment mixture found in the sewer pipes. The observations made on the influence of physical cohesion provide a reference on the sediment stabilizing capacity of the same sandy mixture (with the absence of EPS). It is worth highlighting that the obtained for the non-cohesive sediment was similar to the values predicted by van Rijn [Citation37], in a way validating the method and calibration procedure previously described to obtain the corresponding shear stress values (from the rotational propeller).
The increment of silt content in the mixture evidently increased the critical Shields parameter in an exponential manner. A small fraction of silt (i.e. 10%) does not provide significant changes in the cohesivity of the mixture, where a similar with the non-silty sediment was observed. In a sediment mixture with low content of silt, the sand particles are still in contact with each other forming a skeleton, whereby the silt fills the voids in between the sand grains [Citation38], behaving like a non-cohesive or less cohesive sediment mixture [Citation39]. Increasing the silt to 20%, however, gives 2.5 more of the shear stress needed to initiate sediment movement. For the sediment mixture with 30 and 40% of silt, higher
values were obtained, suggesting stronger bonds of sand-silt sediment mixtures. For a mixture with high silt content, the sand grain loses contact with each other and are juxtaposed in the mud matrix [Citation38], inclining to behave like a cohesive sediment [Citation39,Citation40]. The critical shear stress
increases monotonously with the increasing fraction of mud up to 50% before it ceases to provide any influence (and reaches a plateau) or decreases with increasing mud concentration [Citation38].
3.2. Individual mean plot for each factor
We also want to graphically explore how the average or mean of changes given specific levels of the parameters considered. Thus, we applied the mean plot to assess the importance of each factor as well as the univariate effects, as shown in Figure . All mean
values are plotted on the same scale to assist comparability and relative importance. In general, the mean of the
seems to change with all factors at different levels. We assess the importance of a single factor by looking at the difference between each means and compare with the overall mean as it is drawn as a horizontal line. This plot shows the mean for each level of grouping factor compared to the grand mean (the centre wider line). In this experiment, we are attempting to maximize the response. Thus, we identify the important factor that gives the biggest difference in mean, the second most important factor as the next biggest difference and the least important with the smallest difference. By excluding interactions between factors, we found that the Ratio is the most important factor, EPS is the second and Oil and Grease appear to be the least important factor. The combination of a 60/40 Ratio, 0.10% EPS and 10% Oil and Grease are the best settings in this experiment based on the maximum value of the mean of
. However, at 0% and 0.01% levels of EPS, the influence on the mean of
is not apparent, as the same results were obtained with the 100/0 and 90/10 levels of Ratio. Due to this reason, it is interesting to determine whether the combinations of the levels of the factors differently affect the averaged
through the analysis of three-way interactions.
Table shows the results of three-way ANOVA performed on the data to compare the effect of sediment ratio, fractions of EPS and oil and grease on the critical Shields parameter. Three-way ANOVA revealed that there is a statistically significant difference in the parameters, with p values all < 0.0001.
Table 4. Analysis of variance for θc.
3.3. Confirmation of one factor on threshold criteria of sediment motion
The LSD exercise on the mean for each parameter is shown in Figure . The alphabet in the plot indicates that each variation within the parameter is significantly different when the factor has a different associated alphabet.
Figure 5. Comparison of mean based on (a) Ratio, (b) EPS, and (c) O&G. Means with a similar letter(s) are not significantly different (α = 5%), using the least significant difference (LSD) test. The values a, b and c are individually based on each parameter analysed.

The influence of sediment composition on fine sand silt, as discussed in Section 3.1, is supported by the statistical analysis shown in Figure . Each ratio or sediment fraction is significantly different from the other, as shown by the differences in alphabets based on the LSD test. The inclusion of 10% silt does not affect much on the threshold sediment motion much, although it slightly increased from the 100% sand mixture. The increasing fraction of silt from 20% to 40% obviously changes the , and deviates from the critical value of sand without silt, indicating that underestimation of
is expected when using the homogenous sediment-based empirical equations.
LSD test showed that the concentration of EPS does have a significant influence on the values, except for a low concentration of 0.01%, which has similar behaviour with no EPS at all (0%). The presence of EPS in the mixture evidently increased the θc, particularly at a high concentration of EPS, with approximately
more than the sediment mixture without the influence of microbial cohesion. A similar linear relationship as the homogenous sediment (although higher in magnitude) was observed for the 90/10 sediment mixture. Microbial cohesion strengthens the tensile strength of the silt grains. The suitability of macro cohesion becomes more apparent for a mixture with a significant amount of silt (>20%). At high EPS concentrations, i.e. 0.07 and 0.1%, the increment of 10% silt (from 20%) increased the critical shear stress to about 2.2 times. Higher shear stress is needed as the disruption of the biofilm layer is first needed [Citation41] before exposing the sediment bed and entraining the particles into the water column. EPS increases the tensile strength of fine size sediment through the formation of polymer bridges between the sediment particles, subsequently increasing the inter-particle bonding strength [Citation14,Citation42]. Finer marine sediment can have an increment of
up to 77% higher than the non-biofilm-bound sediment [Citation8,Citation15]. Physical cohesion stabilizes and increases the strength of the sediment mixture by performing flocs [Citation29], providing more strength combined with microbial cohesion. The influence of biofilm on the incipient sediment motion depends on the sediment size, where it ceases to become important outside the 0.2 <
< 50 range [Citation43], where
denotes particle Reynolds number,
is the shear velocity,
is the sediment size, often described as
and
is the water kinematic viscosity.
The biofilm-secreted EPS strands bound the sediment grains together and increased the requirement of shear stress to break the binds and move them [Citation5]. Increasing silt fraction in the mixture creates mud-like characterization and further increases the resilience of the surface to hydrodynamic forcing. The highest percentage of EPS (discussed here) at 0.1% provided strong biostabilisation and increased the critical Shields parameter to more than 30-fold than non-biological influence sediment. The high strength of the bed was able to withstand high shear force and provide resistance to sediment entrainment.
Data shows that by increasing the silt percentage, the dimensionless critical shear stress was too increased. The presence of EPS was found to be influential, where the higher concentration of 0.1% EPS consistently had higher critical mean velocity than the sand-silt-EPS mixture with a low concentration of EPS of 0.01%. The formation of biofilm on the sediment influences the threshold sediment motion when the EPS concentration is more than 0.01%. The presence of EPS does not necessarily pose an immediate effect on the sediment movement, where at 0.01%, the mean is comparable with the baseline value. Increasing the EPS concentration to 0.07% affected the incipient sediment motion and even more at a higher concentration of 0.1%, with an increase of up to 2.5 orders of magnitude. The slimy texture of EPS acts like a glue, holding the particles (and ions) together [Citation44], in a way providing a layer of protection on the bed surface. The EPS connects the particles, improving their strength by enhancing the particle alignment [Citation44] and preventing the particles from moving independently [Citation7]. The biologically cohesive EPS binds to the sand grains and forms floccules with silt or mud, creating more resistance for the sediments to move [Citation45].
The presence of oil and grease on the sediment mixture also influences the threshold sediment motion, where the mean systematically increased with the increasing concentration of O&G on the sediment, as shown in Figure . Additional OG onto the sediment mixture proved to be statistically significant at both 5% and 10% concentration. Although by comparing the influence of EPS on the sediment movement, the effect of O&G is not as critical as the presence of biofilm on sediment.
3.4. Combination of two factors on threshold criteria of sediment motion
In this section, the two-way interaction effects of EPS, fractions of fine sediment and silt (Ratio) and O&G were examined, as shown in Figure . The mean of seems to increase when EPS is changed from 0% to 0.10%, independently of the range of Ratio and Oil and Grease. The mean of
also shows the increasing trend when the Ratio changed from 100/0 to 60/40 independently of the range of O&G. However. There may be little interaction between EPS and Ratio if the lines being close at 100/0 and 90/0 levels of Ratio are considered for both plots of two-way and three-way. Similar results are also recorded on the plot of two-way for EPS and O&G as little interaction exists between EPS and 0% and 5% O&G. Likewise, the plot of two-way for Ratio and O&G also shows little interaction between Ratio and 5% and 10% O&G.
Figure 6. Plot of the two-way interaction between (a) EPS-Ratio, (b) EPS-Oil and Grease and (c) Ratio-Oil and Grease using the mean of .

The results of LSD on the mean comparison of two parameters for the critical Shields parameter are shown in Tables . Recall that the alphabet presented in superscripted in the tables is based on each interaction. Table describes the fine sand-silt distribution (Ratio) and EPS, whilst the Ratio of sediment distribution and percentage of EPS and oil and grease is tabulated in Tables and , respectively.
Table 5. Comparison of mean in the interaction of Ratio × EPS.
Table 6. Comparison of mean in the interaction of Ratio × OG.
Table 7. Comparison of mean in the interaction of EPS × OG.
The comparison of mean between the fine sediment-silt Ratio and the concentration of EPS is shown in Table . Data shows that the sediment mixture of Ratio1/EPS1, Ratio2/EPS1 and Ratio1/EPS2 are not significantly different (due to the same m coefficient). The mixture of Ratio1/EPS3 and Ratio2/EPS2 (where both have coefficient k) too, are significantly similar. A low concentration of EPS at 0.01% is insignificant in the impression of incipient sediment motion, even with the presence of 40% silt. Threshold criteria of sediment motion changes for sediment with higher silt fraction and higher EPS concentration. This indicates that deposited sediment consisting of high silt fraction and prolonged deposition allowing the formation of biofilm will be more difficult to be entrained and move, requiring higher self-cleansing velocity for the removal. Higher EPS will be secreted by a thriving, high-productivity microorganism community in wastewater pipes, particularly with the readily available high amount of organic matter [Citation46].
The comparison of mean between the Ratio of fine sediment to silt and O&G is presented in Table . Additional OG does not provide a significant difference for homogenous sediment (Ratio1), with both 5 and 10% O&G having a similar coefficient k. However, increasing silt fraction evidently showed variation in the coefficients, whereby sediment mixture with silt > 20% has different coefficients. The influence of oil and grease is significant for sediment mixtures with a high fraction of silt (20–40%) and poses no effect on threshold sediment motion for samples with lower silt fractions.
The comparison of mean based on EPS and O&G is shown in Table . Data shows that each EPS/OG combination is significantly different from the other, except for EPS3/OG1 and EPS2/OG3.
3.5. Combination of three factors on threshold criteria of sediment motion
Plots of three-way interaction effects of EPS, Ratio and O&G are shown in Figures . The mean of seems to increase when EPS is changed from 0% to 0.10%, independently of the range of Ratio and Oil and Grease. The mean of
also shows the increasing trend when the Ratio changed from 100/0 to 60/40 independently of the range of O&G.
Figure 7. Plot of the three-way interaction between EPS, Ratio and 0% Oil and Grease using the mean of .

Figure 8. Plot of the three-way interaction between EPS, Ratio and 5% Oil and Grease using the mean of .

Figure 9. Plot of the three-way interaction between EPS, Ratio and 10% Oil and Grease using the mean of .

Table shows the interaction between each variable, the Ratio of fine sediment-silt (Ratio), the concentration of EPS and the percentage of oil and grease (O&G) in a sediment mixture, where the superscripted alphabet describes whether they are statistically significant with each other. To assist with the discussion, the results for Ratio 5 will be first explained as the LSD test only produced a single alphabet (coefficient) for each sediment mixture. The highest mean is observed for sediment mixture with a 60/40 ratio of fine sand to silt, with EPS concentration of 0.1% and O&G 10%. The samples with high fractions of silt (40%) are all significant with each other, and those samples with 30% silt and high EPS concentration (0.07–0.10%). Decreasing the fraction of silt to 30%, samples with O&G (5 and 10%) are not significant. At low concentrations of EPS, the presence of oil and grease influences the threshold criteria of sediment motion. A smaller fraction of silt at 20% provides more sediment mixtures statistically insignificant with each other, whereby the oil and grease provide no influence on the mean
. A consistent trend of increasing 0.01% EPS has no influence on the threshold sediment motion for all sets of fractions. For sediment mixture with no or 10% of silt, the presence of oil and grease is not significantly different and only becomes influential when EPS concentration is high.
Table 8. Comparison of mean for the interaction of Ratio × EPS × O&G.
4. Conclusion
The incipient sediment motion of a sediment mixture with effects from physical cohesion, in terms of varying silt fraction, microbial cohesion and presence of O&G, is investigated. Laboratory experiments and observations were used to evaluate the interactions between each parameter based on the statistical analysis. The combination of parameters provides insight into how physical, microbial cohesions and O&G alter the value of critical Shields parameter . Increasing
was observed for sediment mixture as the fraction of silt increased, and the presence of EPS and O&G up to more than 30 times than only homogenous sediment (with no EPS and O&G). The sediment mixture becomes cohesive, stronger and able to withstand high shear stress indicating higher “self-cleansing” velocity, and a different design approach in sewerage pipes is essential. Statistical analysis showed that the influence of physical cohesion is more significant than microbial cohesion, with the presence of O&G turning out to be less critical of the three.
Acknowledgement
The authors are grateful to the Ministry of Higher Education, Malaysia for the financial support through the Fundamental Research Grant Scheme FRGS/1/2018/TK01/UKM/02/4 and Mrs Nur Azura Abdullah and Mr Mohamed Zuraimi Abdullah for the technical support given.
Disclosure statement
No potential conflict of interest was reported by the author(s).
Additional information
Funding
References
- Regueiro-Picallo M, Naves J, Anta J, et al. Monitoring accumulation sediment characteristics in full scale sewer physical model with urban wastewater. Water Sci Technol. 2017;76(1):115–123.
- Wan Mohtar WHM, Afan H, El-Shafie A, et al. Influence of bed deposit in the prediction of incipient sediment motion in sewers using artificial neural networks. Urban Water J. 2018;15(4):296–302.
- Crabtree RW. Sediments in sewers. Water Environ J. 1989;3(6):569–578.
- Sharif K, Gormley M. Integrating the design of tall building, wastewater drainage systems into the public sewer network: a review of the current state of the Art. Water. 2021;13(22):3242.
- Tolhurst TJ, Gust G, Paterson DM. The influence of an extracellular polymeric substance (EPS) on cohesive sediment stability. In: JC Winterwerp, C Kranenburg, editors. Fine sediment dynamics in the marine environment, proceedings in marine science series, vol. 5. Amsterdam: Elsevier (incl. Pergamon); 2002. p. 409–425.
- Noffke N, Paterson D. Microbial interactions with physical sediment dynamics, and their significance for the interpretation of earth’s biological history. Geobiology. 2008;6:1–4.
- Malarkey J, Baas JH, Hope JA, et al. The pervasive role of biological cohesion in bedform development. Nat Commun. 2015;6(October):6257.
- Vignaga E, Sload DM, Lou X, et al. Erosion of biofilm-bound fluvial sediments. Nat Geosci. 2013;6:770–775.
- Baas JH, Davies AG, Malarkey J. Bedform development in mixed sand-mud: the contrasting role of cohesive forces in flow and bed. Geomorphology. 2013;182:19–32.
- Porhemmat M, Wan Mohtar WHM, Chuah RE, et al. The comparison of empirical formula to predict the incipient motion of weak cohesive sediment mixture. Jurnal Teknologi (Sci Eng). 2016;78(9–4):109–114.
- Fang HW, Chen YS, Huang L, et al. Biofilm growth on cohesive sediment deposits: laboratory experiment and model validation. Hydrobiologia. 2017;799:261–274.
- Tian T, Yang Q, Wei G, et al. Changes of substrate microbial biomass and community composition in a constructed mangrove wetland for municipal wastewater treatment during 10-years operation. Mar Pollut Bull. 2020;155:111095.
- Chen XD, Zhang CK, Paterson DM, et al. The effect of cyclic variation of shear stress on non-cohesive sediment stabilisation by microbial biofilms: The role of “biofilm precursors.”. Earth Surf Processes Landforms. 2019;44(7):1471–1481.
- Zhou Y, Yao X, Gu Y, et al. Biological effects on incipient motion behavior of sediments with different organic matter content. J Soils Sediment. 2021;21:627–640.
- Fang H, Shang Q, Chen M, et al. Changes in the critical erosion velocity for sediment colonised by biofilm. Sedimentology. 2014;61:648–659.
- Fang H, Huang L, Zhao H, et al. Biofilm growth and the impacts on hydrodynamics. In: H Fang, L Huang, H Zhao, et al., editors. Mechanics of Bio-sediment transport. Berlin: Springer; 2020.
- Cheng W, Fang H, Lai H, et al. Effects of biofilm on turbulence characteristics and the transport of fine sediment. J Soils Sediments. 2018;18:3055–3069.
- Husain IAF, Alkhatib MF, Jammi MS, et al. Problems, control and treatment of fat, oil, and grease (FOG): a review. J Oleo Sci. 2014;63(8):747–752.
- Wallace T, Gibbons D, O’Dwyer M, et al. International evolution of fat, oil and grease (FOG) waste management – A review. J Environ Manag. 2017;187:424–435.
- He X, de los Reyes FL, Leming ML, et al. Mechanisms of fat, oil and grease (FOG) deposit formation in sewer lines. Water Res. 2013;47(13):4451–4459.
- USEPA. (2004). Report to Congress: Impacts and control of CSOs and SSOs. Office of Water, United States Environmental Protection Agency, Washington D.C. EPA B33-R-04-001.
- Post JAB, Pothof IWM, ten Veldhuis M, et al. Statistical analysis of lateral house connection failure mechanisms. Urban Water J. 2016;13(1):69–80.
- Abd. Jalil J, Wan Mohtar WHM, Mohamod MT, et al. Subjectivity effect on the determination of incipient sediment motion. Jurnal Kejuruteraan - (Special Issue 1). 2017:25–30.
- Muhammad Azha NI, Wan Mohtar WHM. Threshold criteria of sediment motion for biological cohesive sediment mixture. Jurnal Kejuruteraan Special Issue. 2018;5:83–89.
- Shields A. Application of similarity principles and turbulence research to Bed-Load movement. Mitt Preuss Versuchsanst Wasserbau Schiffbau. 1936;26(5–24):47.
- Dey S. Sediment threshold. Appl Math Model. 1999;23:399–417.
- Mantz PA. Incipient transport of fine grains and flanks by fluids-extended shields diagram. J Hydraul Div. 1977;103(6):601–615.
- Grabowski RC, Droppo IG, Wharton G. Erodibility of cohesive sediment: the importance of sediment properties. Earth Sci Rev. 2011;105:101–120.
- Winterwerp JC, Van Kesteren WGM. Introduction to the physics of cohesive sediment in the marine environment. Amsterdam: Elsevier B.V.Wotton, R. S. in Oceanography and Marine Biology: An Annual Review 42 (Eds Gibson, R. N.) 57–94 (CRC, 2004); 2004.
- Kramer H. Sand mixtures and sand movement in fluvial models. Trans ASCE. 1935;100(1909):798–838.
- van Rijn LC. Unified view of sediment transport by currents and waves. II: suspended transport. J Hydraulic Eng. 2007;133(6):668–689.
- Travis MJ, Weisbrod N, Gross A. Accumulation of oil and grease in soils irrigated with greywater and their potential role in soil water repellency. Sci Total Environ. 2008;394:68–74.
- Parsons DR, Schindler RJ, Hope JA, et al. The role of biophysical cohesion on subaqueous bed form size. Geophys Res Lett. 2016;43(4):1566–1573.
- van de Lageweg WI, McLelland SJ, Parsons DR. Quantifying biostabilisation effects of biofilm-secreted and extracted extracellular polymeric substances (EPSs) on sandy substrate. Earth Surf Dynam. 2018;6:203–215.
- Lanuru M, Riethmüller R, van Bernem C, et al. The effect of bedforms (crest and trough systems) on sediment erodibility on a back-barrier tidal flat of the East Frisian Wadden Sea, Germany. Estuar Coastal Shelf Sci. 2007;72:603–614.
- Paterson DM, Tolhurst TJ, Kelly JA, et al. Variations in sediment properties, Skeffling mudflat, Humber Estuary, UK. Cont Shelf Res. 2000;20:1373–1396.
- van Rijn LC. Sediment transport. Part I: Bed load transport. J Hydraul Eng. 1984;110(10):1431–1456.
- Chen D, Zheng J, Zhang C, et al. Critical shear stress for erosion of sand-mud mixtures and pure mud. Front Mar Sci. 2021;8:713039.
- Wu W, Perera C, Smith J, et al. Critical shear stress for erosion of sand and mud mixtures. J Hydraul Res. 2017;56:1–15.
- Smith J, Perkey D, Priestas A. (2015). Erosion thresholds and rates for sand-mud mixtures, in Proceedings of the 13th International Conference on Cohesive Sediment Transport Processes, Leuven.
- Fang H, Fazeli M, Cheng W, et al. Transport of biofilm-coated sediment particles. J Hydraul Res. 2016;54(6):631–645.
- Chenu C, Guerif J. Mechanical strength of clay minerals as influenced by an adsorbed polysaccharide. Soil Sci Soc Am J. 1991;55:1076–1080.
- Gu Y, Zhang Y, Qian D, et al. Effects of microbial activity on incipient motion and erosion of sediment. Environ Fluid Mech. 2020;20(1):175–188.
- Costa YAO, Raaijmakers JM, Kuramae EE. Microbial extracellular polymeric substances: ecological function and impact on soil aggregation. Front Microbiol. 2018;9:1636.
- Wotton RS. The ubiquity and many roles of exopolymers (EPS) in aquatic systems. Sci Mar. 2004;68:13–21.
- Sheng GP, Yu HQ, Li XY. Extracellular polymeric substances (EPS) of microbial aggregates in biological wastewater treatment systems: A review. Biotechnol Adv. 2010;28:882–894.