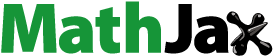
Abstract
This work aims to examine the flow of tangent hyperbolic (T-H) ternary hybrid nanofluid (THNF) over a rough-yawed cylinder generated by impulsive motion in a mixed convection mechanism with periodic magnetohydrodynamics. The surface roughness is depicted by a high-frequency sine wave with a small amplitude. The governing equations of a system were converted to a dimensionless form by using semi-similar transformations. After linearizing the equations with a Quasilinearization technique, they were discretized with the implicit finite difference method. Including a third component (i.e. MoS2) of the THNF decays the fluid velocity. Raising magnetic field parameter M from 0 to 2 boosts the for THNF by 19% at
. The
increases to a maximum of 288% for the T-H and 101% for the Newtonian THNF as the roughness attribute
increases from 0.001 to 0.01 for
at
. The findings were compared to previously published to demonstrate the numerical method's reliability.
1. Introduction
There are many different technological and industrial circumstances in which one can observe the consequences of heat transfer phenomena. Water, ethanol, and other common fluids, among others, have a heat transfer coefficient that is on the low end of the scale. The researchers look into the concept of nanofluid and hybrid nanofluid [Citation1–4] for the effective heat transfer. The idea of a ternary hybrid nanofluid is something that has been developed in order to improve the effectiveness of heat transfer. The mixture is called a hybrid nanofluid when multiple nanoparticle species coexist in the same fluid. Specifically, when three different nanoparticles are combined, a ternary hybrid nanofluid (THNF) is produced. Until recently, research on hybrid nanofluids has only involved those with two components. Because of the increased energy transfer rates displayed by THNF [Citation5–7], researchers are currently focusing on understanding their unique characteristics. These fluids interest scientists and engineers because of their potential applications in various fields, including the pharmaceutical and nuclear security sectors, the cooling of electrical devices, and many others. Oke [Citation5] has recently investigated the effect of a revolving surface on a THNF based on ethylene glycol. Gul and Saeed [Citation6] have recently conducted research on THNF flow in a porous medium over a nonlinearly expanding surface. Researchers Nazir et al. [Citation7] looked at using ternary hybrid nanomaterials in a partially ionized hyperbolic tangent material to generate thermal energy.
In response to a sudden change in the velocity field, inviscid flow develops instantly. The flow in the viscous layer close to the wall, on the other hand, grows more slowly but eventually stabilizes into a steady state. Over short time scales, viscous forces and unstable acceleration dominate the flow, whereas viscosity, pressure gradient, and convective acceleration dominate over long-time scales. In contrast to the big-time scale, where the flow is influenced by conditions at the leading edge and the stagnation point, the flow on the small-time scale is largely unaffected by these factors. The Rayleigh equation applies for a short period of time, while the Falkner-Skan equation governs the problem mathematically. Only a few studies have looked at impulsive motion on different geometries like cones, flat plates, and rotating spheres [Citation8–12]. In these problems, to simplify the governing equations while maintaining their essential structure, a semi-similar method was employed to simplify the number of independent variables. Since non-Newtonian fluids possess rheological properties distinct from those of Newtonian fluids, numerous models have been developed to describe them adequately. Some of these models include the Carreau, Williamson, Cross, Sisko, Jeffery, Ellis and tangent hyperbolic (T-H) [Citation13–15] models, amongst others. The last model describes a hyperbolic tangent fluid with pseudo-plastic properties. In this type of fluid, the viscosity of the fluid decreases as the shear rate increases. Blood, paints, whipped cream, and ketchup are all types of substances that can be considered examples of this substance. The diverse array of disciplines, including geophysics, biology, metallurgy, chemistry, petroleum, and many more, that use this rheological model, provides the impetus for the research being conducted on it. This non-Newtonian model is also frequently applied when evaluating the machinery used in the laboratory. In addition to this, the fluid accurately reflects the physical characteristics of blood and makes it possible to conduct a more accurate investigation into the phenomenon of shear thinning. Within the context of these implications, researchers have recently reported several different approaches. Awais et al. [Citation13] experimented with optimizing the entropy of a T-H nanofluid in a flat plate using the concept of activation energy. The flow of T-H nanofluid was studied by Ibrahim and Gizewu [Citation14] using a modified version of Fourier's and Fick's diffusion models. This model was used to study the flow of T-H nanofluid around a stretched surface. Jamshed et al. [Citation15] conducted research on a single-phase thermal T-H hybrid nanofluid in conjunction with an entropy study.
During the manufacturing process, the surface of a material may become rough. As a consequence, it significantly impacts the formation of boundary layer features in mixed convective flow over surfaces with a range of different roughness and geometries. Patil et al. [Citation16–18] investigated how surface roughness affects the boundary layer's mixed convective flow and found that it can delay the layer's point of separation across various geometries.
Magnetohydrodynamics (MHD) [Citation19,Citation20] takes into account the behaviour of a large volume of electrically conducting and flowing liquid. This fluid could be anything from plain water to blood plasma. The effects of magnetohydrodynamics (MHD) on liquid metal were related to developing an electromagnetic pump, which is frequently attributed to Hartman. The peculiarities and general behaviour of MHD flow have been investigated using a wide variety of numerical methods. Researchers have nonetheless devoted a lot of time to studying the periodic magnetic field in order to examine the flow patterns. Utilizing magnetohydrodynamic induction, electromagnetic induction drives supply power to induction models and are thus real-world examples of periodic MHD. In the metal purification industry, high-frequency periodic magnetic fields are used to induce eddy currents in a pool of molten metal, which then react in rapid succession to the magnetic field. Other significant applications include the aerospace industry, particularly missile aerodynamics, the petroleum industry, fluid droplet spray, electromagnetically driven heat sinks, seawater boundary drag modification, and so on. However, there is a dearth of research on periodic MHD combined with convection [Citation21–23].
Studying fluid flows around yawed cylinders [Citation24–29] in the design of fluid equipment used in various industrial transport processes has increased interest in this area of study. Yawed-shaped bodies are used in a wide variety of practical contexts, such as in overhead cables, bridge stay cables, chimney stacks, and many more. Engineers working with such systems must comprehend how yaw angle affects the rate of energy transfer via variables such as fluid velocity, temperature, etc. For the first time, Roy [Citation24] looked at the consequences of non-uniform mass transfer on a yawed cylinder. Later, Roy and Saikrishnan [Citation25] used the non-uniform slot suction they had constructed to further investigate yawed cylinders, building on the work of Roy [Citation24]. Thakur et al. [Citation26] analyzed the wake flow effects of both single and multiple cylinders that have been yawed. Revathi et al. [Citation27] conducted a study on forced convective flow through a yawed cylinder, taking temporal variations and non-uniform suction into account (blowing). It is important to know the impact of sudden change in the fluid motion (impulsive motion) and roughness on the fluid flow characteristics.
Based on the literature review, we can confidently assert that no academic has studied the effect of roughness and T-H ternary hybrid nanofluid flow around a yawed cylinder in the context of mixed convection caused by impulsive motion. Here, the surface roughness is depicted by a high-frequency sine wave with a small amplitude. The governing equations of a system were converted to a dimensionless form by using semi-similar transformations. After linearizing the equations with a Quasilinearization technique, they were discretized with the implicit finite difference method. This study aims to investigate the following novel cutting-edge concepts in particular:
Influence of cylinder's surface roughness on the flow.
Effects of ternary hybrid nanofluid and impulsive motion.
Influence of the tangent hyperbolic (T-H) fluid model on the flow characteristics.
Periodic MHD impact.
A comparison of various fluids is taken into account.
2. Mathematical analysis
Here, we assume a two-dimensional unsteady laminar incompressible T-H ternary hybrid nanofluid flow across a yawed cylinder with a yaw angle between 15° and 60° in mixed convection mechanism as seen in Figure . When , the cylinder is vertical and when
, it is horizontal. Thus, we have studied the yaw angle between 15° and 60° to achieve the effects of a yawed cylinder. Flow in the x-direction is called chordwise flow, and flow in the z-direction is called spanwise flow. Let u, v, and w stand for the x, y, and z components of velocities, respectively. The assumed wall temperature
is higher than the fluid's actual temperature of flow. Flow velocities along the chord and spanwise flow directions are represented by
and
, respectively. Let
denote the yaw angle and R the radius of the infinitely yawed cylinder. An impulsive motion caused by the freestream is considered here. A periodic magnetic field of strength B is applied in
-direction, which is also responsible for the sudden movement in the flow field. A high-frequency, low-amplitude sine wave represents surface roughness in simulations. To incorporate density variations into the momentum equation, the Boussinesq approximation has been used [Citation16–18,Citation30–32].
For a tangent hyperbolic fluid, we have the following constitutive equation [Citation13,Citation14]:
where,
is the extra stress tensor,
are zero and infinite shear rate viscosity, s is the power law index,
is material constant and
is given by:
where,
. Here,
as it is impossible to measure infinite shear rate and invoking the shear thinning effect (i.e.
) for the tangent hyperbolic fluid, extra stress tensor becomes,
With the above assumptions taken into account, once the boundary layer approximations are applied, the governing equations expressed as [Citation13–15,Citation16,Citation21,Citation22, Citation28,Citation29]:
(1)
(1)
(2)
(2)
(3)
(3)
(4)
(4)
Initial conditions:
(5)
(5) Boundary conditions (B. Cs.):
(6)
(6) Where,
.
Semi-similar transformations: ;
;
;
;
;
;
;
.
Therefore, we get and
.
With these semi-similar transformations, while Eq. (1) is identically satisfied, Eqs. (2)–(4) take the form:
(7)
(7)
(8)
(8)
(9)
(9)
The pertinent boundary constraints are:
(10)
(10) Where,
Also , as we have considered impermeable surface,
.
Coefficient of skin friction (in chordwise direction):
(11)
(11) Coefficient of skin friction (in spanwise direction):
(12)
(12) Heat transfer rate:
(13)
(13)
3. Numerical method
The following are the set of linear equations obtained by employing the Quasilineazation technique [Citation28,Citation33, Citation34–36] to the dimensionless Eqs. (7)–(9).
(14)
(14)
(15)
(15)
(16)
(16)
The equivalent boundary conditions are:
(17)
(17) The implicit finite difference technique [Citation37–39] is applied to the obtained set of linear equations (14)–(16) for discretization. After that, Varga's matrix inverse technique [Citation40] is implemented to solve the resulting finite difference equations with the matrix of coefficients in block tridiagonal form. Here, the step lengths
are taken to be 0.001. With a precision of 10−4, the findings iteratively converge to the desired values, i.e.:
The coefficients of equations (14)–(16) are:
Where,
3.1. Results comparison
For the authentication of the numerical approach, the current outcomes are compared with the results attained by Roy and Anilkumar [Citation41] and Takhar et al. [Citation42] for the Nusselt number in Table when Pr = 7.0,
and
. The comparison demonstrates a definitive agreement between the current findings and those obtained in [Citation41] and [Citation42].
Table 1. Comparison of heat transfer rate.
3.2. Grid discretization
The grid discretization of a numerical method is defined by the number of grids used (or the grid size). The accuracy and computing time of the solution will grow as the number of grids is increased until a particular ideal number of grids is reached. The grid discretization is shown in Figure with and
mesh sizes in the x and y directions. The grid is denoted by the ordered pair (i, j), and the total number of such pairings is called the grid number.
4. Results and discussion
In this section, various graphs on profiles and gradients were used to illustrate the typical effects of a number of variables, including the Weissenberg number , periodic magnetic field
, nanoparticle volume fraction
, Richardson number
, yaw angle
, roughness parameter
, frequency
, and power-law index
. Unless and until they were explicitly stated, some parameters, such as,
,
,
were assigned constant values throughout the numerical computation. In addition, for the current study, we considered a three-component or ternary hybrid nanofluid (THNF) with Ag (Silver), TiO2 (Titanium dioxide), and MoS2 (Molybdenum disulphide). Table shows the expressions for the various properties of these nanoparticles, and Table shows the thermal properties.
Table 2. Expressions for ternary hybrid nanofluid characteristics [Citation6].
Table 3. Thermal characteristics of the nanoparticles [Citation43–45].
4.1. Effects of 

Figures present the impact of on velocity profile
, and corresponding gradients in the chordwise
and spanwise
directions. The roughness of the surface is responsible for the sinusoidal oscillations in the chordwise friction coefficient. Here,
characterizes the Newtonian fluid, whereas
characterizes the tangent-hyperbolic (T-H) fluid (non-Newtonian). Compared to Newtonian THNF, the fluid velocity slows, and the chord and spanwise friction coefficients accelerate impulsively (i.e.
enhanced from 0 to 2). This has relied on the viscosity of the T-H THNF, which is more viscous than the Newtonian one. Notably, the highest percentage enhancement, approximately 241% and 148% in the chord and spanwise friction coefficients, are observed at
and
respectively when
enhanced from 0 to 1. Also, since the
serves as a favourable pressure gradient, one can observe the increasing friction coefficients through Figures and . As a result of the flow's impulsive nature, smaller
values reveal substantial fluctuations across all gradients.
Further, the inclusion of a third component (i.e. MoS2) of the THNF decays the as seen in Figures and . That is, the non-zero value of
causes such variations due to the addition of third component of the THNF makes the fluid thicker than that of the 2-components hybrid nanofluid (HNF). Following Figures and , the non-zero value of
upsurges both the chord and spanwise friction coefficient due to the collision of the nanoparticles caused by the impulsive motion of the fluid. Furthermore, it is clear from Figures and that Lorentz force is generated by periodic MHD and augments
and
for the growing values of M from 0 to 2. Exactly opposite nature of the results can be seen in the case of
. In particular, a change in the M from 0 to 2 improves the
19% and decays the
50% approximately at
in the THNF.
4.2. Effect of surface roughness
The variations of for various values of rough surface parameters
and frequency
are graphed in Figure (a–c) for both T-H and Newtonian THNF cases. In Figure (a), the rough surface with
varying encounters levels of skin friction with more rapid changes as frequency n increases. This impact is accentuated by T-H THNF (We = 1), as shown in Figure (a). As can be seen in Figure (b), the roughness amplitude
has a more pronounced impact on
, with more significant amplitude fluctuations, than the roughness amplitude
. In Figure (c), a substantial amplitude of skin-friction variations is observed for the roughness parameter
compared to smaller values of
due to the T-H fluid feature (We = 1) and the flow's impulsive nature. In addition, it is owing to the T-H fluid's shear-thinning behaviour and the fluid being trapped in the deeper cavities generated due to the more excellent surface asperities formed on the rough surface. In particular, the
increases to a maximum of 288% for the T-H and 101% for the Newtonian THNF as the
increases from 0.001 to 0.01 at
and frequency
.
4.3. Effects of 

The impacts of the power-law index and yaw angle
over
,
and
are portrayed in Figures . The fluid velocity and skin friction coefficient are observed to increase in the chordwise direction when the yaw angle
rises. In contrast, the opposite pattern holds true for
. In other words, as the cylinder's yaw angle (the degree to which it is tilted) grows, the flow starts impulsively, and so the fluid pressure inside it rises, increasing its chordwise velocity and friction coefficient. It is noticed that at
,
the chord and spanwise friction coefficients enhance and decay by approximately 38% and 69%, respectively, when
improving from 15° to 60°. Further, the effect of the power-law index
can be seen through Figures . The chordwise velocity distribution and the friction coefficients along both directions augment the higher values
. Evidence for this can be seen in the fact that as the power-law index m increases, the fluid's characteristic shifts from shear-thinning to shear-thickening. Also, it is clear that upsurging
from 0.2 to 0.5 enhance the chord and spanwise friction coefficients by approximately 56% and 69%, respectively at
.
4.4. Influence of 
and different fluids
Figures discuss the significance of periodic MHD and T-H ternary hybrid nanofluid for the purpose of very efficient heat transfer. Here, ,
,
,
, respectively represent the T-H base fluid, Ag T-H nanofluid, Ag-TiO2 two component T-H HNF and Ag-TiO2-MoS2 T-H THNF The temperature distribution
, energy transfer strength
and chordwise friction coefficient
escalate by adding different component's nanoparticles to the base fluid. In other words, especially the
is significantly less for the T-H base fluid and is large for the T-H THNF. Also, the
for the two components, HNF vary in between the T-H base fluid and T-H ternary HNF. In particular to say that, the
found to upsurge approximately by 15% for the T-H THNF than that of only T-H base fluid. Further, the period MHD significance is noticed in Figures . The non-zero values of M
indicate the presence of the periodic MHD while
representing the absence of periodic MHD. Due to the presence of Lorentz force generated by the periodic MHD, the
and
are found to be escalated while the
de-escalated.
Figure 13. Variation in fluid temperature for different fluids considered in the presence and absence of periodic magnetic field.

Figure 14. Variation in heat transfer rate for different fluids considered in the presence and absence of periodic magnetic field.

4.5. Nanoparticle's shape factor effect
Nanoparticle heat conduction critically depends on their shapes. Figures and throw light on how the shape factors of nanoparticles affect the fluid temperature and and energy transport strength
. Here, we discuss nanoparticles of various forms, including spherical (s = 3.0), hexahedral (s = 3.7221), tetrahedral (s = 4.0613), column (s = 6.3698), and lamina (s = 16.1576) [Citation36]. The shape factor s can be determined by knowing the sphericity of the nanoparticle's shape. The sphericity and shape factors are inversely proportional to one another, given by
, where
is the sphericity. It has been examined from Figures and that the emerging values of s boost the
and
i.e. changing the shapes of nanoparticles from spherical to other different shapes enhances the
and
. Concisely at
, the
boosted approximately by 41%, when the shapes of the nanoparticles were changed from spherical to the lamina. Further, different shapes of the nanoparticles are given in Figure .
Figure 18. Shape factors of various nanoparticles [Citation46]. (a) Sphere (s = 3.0); (b) Hexahedron (s = 3.7221); (c) Tetrahedron (s = 4.0613); (d) Column (s = 6.3698); (e) Lamina (s = 16.1576).
![Figure 18. Shape factors of various nanoparticles [Citation46]. (a) Sphere (s = 3.0); (b) Hexahedron (s = 3.7221); (c) Tetrahedron (s = 4.0613); (d) Column (s = 6.3698); (e) Lamina (s = 16.1576).](/cms/asset/92a1c1f3-7cfa-4ac1-9ead-34ce46c038c8/tusc_a_2199664_f0018_oc.jpg)
5. Conclusions
This study discussed the main topic of the tangent hyperbolic THNF flow across a rough-yawed cylinder caused by impulsive motion in a mixed convection mechanism with periodic MHD. The following are the key insights from this study:
The fluid velocity decelerates, and chord and spanwise friction coefficients impulsively accelerate for the T-H THNF compared to Newtonian THNF (i.e.
enhances from 0 to 2).
Notably, the highest percentage enhancement, approximately 241% and 148% in the chord and spanwise friction coefficients are observed at
and
respectively when
enhanced from 0 to 1.
Including the third component (i.e. MoS2) of the THNF decays the
.
The
augments for the presence of periodic magnetic field (i.e. non-zero M) in comparison to the case of absence of the magnetic field (M = 0).
A change in the M from 0 to 2 improves the
19% and decays the
50% approximately at
in the THNF.
The
increases to a maximum of 288% for the T-H and 101% for the Newtonian THNF as the
increases from 0.001 to 0.01 at
and frequency
.
The fluid velocity and skin friction coefficient are observed to increase in the chordwise direction when the yaw angle
rises.
It is noticed that at
,
the chord and spanwise friction coefficients enhance and decay by approximately 38% and 69%, respectively, when
improves from 15° to 45°.
The chordwise velocity distribution and the friction coefficients along both directions augment the emerging higher values of
.
The
found to upsurge approximately by 15% for the T-H ternary THNF than that of only T-H base fluid.
The emerging values of s boost the
and
, i.e. changing the shapes of nanoparticles from spherical to other different shapes enhances the
and
.
Nomenclature
= | Prandtl number | |
= | Richardson number | |
= | local Reynolds number | |
= | thermal conductivity | |
= | power-law index | |
= | non-dimensional frequency | |
= | Weissenberg number | |
= | non-dimensional velocities in chord and spanwise directions | |
= | acceleration due to gravity | |
= | periodic MHD parameter | |
= | Eckert number | |
= | non-dimensional temperature | |
= | fluid temperature | |
= | time | |
= | nanoparticle's shape factor | |
= | non-dimensional stream function | |
= | free stream velocities in |
Greek symbols
= | thermal expansion coefficient | |
= | volume fractions of nanoparticles | |
= | stream function | |
= | time constant | |
= | yaw angle | |
= | transformed variables | |
= | roughness parameter | |
= | non-dimensional time | |
= | frequency | |
= | dynamic viscosity | |
= | kinematic viscosity | |
= | electrical conductivity | |
= | density |
Abbreviations
T-H | = | tangent hyperbolic |
HNF | = | hybrid nanofluid |
THNF | = | ternary hybrid nanofluid |
Subscripts
= | partial derivatives with respect to | |
= | at the wall and ambient fluid, respectively | |
f | = | properties of the T-H fluid |
nf | = | properties of T-H nanofluid |
hnf | = | properties of T-H two-components hybrid nanofluid |
= | properties of T-H ternary hybrid nanofluid | |
i | = | initial condition |
Disclosure statement
No potential conflict of interest was reported by the author(s).
References
- Bhatti MM, Öztop HF, Ellahi R. Study of the magnetized hybrid nanofluid flow through a flat elastic surface with applications in solar energy. Materials (Basel). 2022;15:7507.
- Selimefendigil F, Oztop HF. Thermal management and performance improvement by using coupled effects of magnetic field and phase change material for hybrid nanoliquid convection through a 3D vented cylindrical cavity. Int J Heat Mass Transf. 2022;183:122233.
- Alsubie A. Boundary layer Darcy-Forchheimer couple stress hybrid nanofluid flow over a quadratic stretching surface due to nonlinear thermal radiation. J Taibah Univ Sci. 2021;15(1):1188–1195.
- Pandey AK, Upreti H, Joshi N, et al. Effect of natural convection on 3D MHD flow of MoS2–GO/H2O via porous surface due to multiple slip mechanisms. J Taibah Univ Sci. 2022;16(1):749–762.
- Oke AS. Heat and mass transfer in 3D MHD flow of EG-based ternary hybrid nanofluid over a rotating surface. Arab J Sci Eng. 2022;47(12):16015–16031. doi:10.1007/s13369-022-06838-x
- Gul T, Saeed A. Nonlinear mixed convection couple stress trihybrid nanofluids flow in a Darcy–Forchheimer porous medium over a nonlinear stretching surface. Waves Random Complex Media. 2022:1–18. doi:10.1080/17455030.2022.2077471
- Nazir U, Sohail M, Hafeez MB, et al. Significant production of thermal energy in partially ionized hyperbolic tangent material based on ternary hybrid nanomaterials. Energies. 2021;14(21):6911. doi:10.3390/en14216911
- Singh PJ, Roy S. Unsteady mixed convection flow over a vertical cone due to impulsive motion. Int J Heat Mass Transf. 2007;50(5-6):949–959. doi:10.1016/j.ijheatmasstransfer.2006.08.011
- Seshadri R, Sreeshylan N, Nath G. Unsteady mixed convection flow in the stagnation region of a heated vertical plate due to impulsive motion. Int J Heat Mass Transf. 2002;45(6):1345–1352. doi:10.1016/S0017-9310(01)00228-9
- Patil PM, Benawadi S, Sheremet M. The unsteady nonlinear convective flow of a nanofluid due to impulsive motion: triple diffusion and magnetic effects. Therm Sci Eng Progr. 2022;35:101456.
- El-Zahar ER, Mahdy AEN, Rashad AM, et al. Unsteady MHD mixed convection flow of non-Newtonian Casson hybrid nanofluid in the stagnation zone of sphere spinning impulsively. Fluids. 2021;6(6):197. doi:10.3390/fluids6060197
- Mahdy A, Ahmed SE. Unsteady MHD convective flow of non-Newtonian Casson fluid in the stagnation region of an impulsively rotating sphere. J Aerosp Eng. 2017;30(5):04017036. doi:10.1061/(ASCE)AS.1943-5525.0000700
- Awais M, Kumam P, Memoona AA, et al. Impact of activation energy on hyperbolic tangent nanofluid with mixed convection rheology and entropy optimization. Alex Eng J. 2021;60(1):1123–1135. doi:10.1016/j.aej.2020.10.036
- Ibrahim W, Gizewu T. Tangent hyperbolic nanofluid with mixed convection flow: an application of improved Fourier and Fick’s diffusion model. Heat Trans Asian Res. 2019;48(8):4217–4239. doi:10.1002/htj.21589
- Jamshed W, Nisar KS, Isa SSPM, et al. Computational case study on tangent hyperbolic hybrid nanofluid flow: single phase thermal investigation. Case Stud Therm Eng. 2021;27:101246. doi:10.1016/j.csite.2021.101246
- Patil PM, Kulkarni M. Nonlinear mixed convective nanofluid flow along moving vertical rough plate. Rev Mex de Fis. 2020;66(2):1153–1161.
- Patil PM, Shankar HF, Sheremet MA. Mixed convection of silica–molybdenum disulphide/water hybrid nanoliquid over a rough sphere. Symmetry. 2021;13(2):236.
- Patil PM, Doddagoudar SH, Hiremath PS. Impacts of surface roughness on mixed convection nanofluid flow with liquid hydrogen/nitrogen diffusion. Int J Numer Methods Heat Fluid Flow. 2019;29(6):2146–2174. doi:10.1108/HFF-11-2018-0703
- Zhang L, Tariq N, Bhatti MM. Study of nonlinear quadratic convection on magnetized viscous fluid flow over a non-Darcian circular elastic surface via spectral approach. J Taibah Univ Sci. 2023;17(1):2183702.
- Alarabi TH, Elgazery NS, Elelamy AF. Mathematical model of oxytactic bacteria’s role on MHD nanofluid flow across a circular cylinder: application of drug-carrier in hypoxic tumour. J Taibah Univ Sci. 2022;16(1):703–724.
- Ahmed R, Uddin R, Jewel Rana BMJ, et al. Study on periodic MHD flow with temperature dependent viscosity and thermal conductivity past an isothermal oscillating cylinder. World J Mech. 2016;06(11):419–441. doi:10.4236/wjm.2016.611030
- Al-Mamun A, Arifuzzaman SM, Reza-E-Rabbi S, et al. Numerical simulation of periodic MHD casson nanofluid flow through porous stretching sheet. SN Appl Sci. 2021;3(2):271. doi:10.1007/s42452-021-04140-3
- Kumar M, Mondal PK. Buoyancy driven flow of a couple stress fluid from an isothermal vertical plate: the role of spatially periodic magnetic field. Phys Scr. 2021;96(12):125014. doi:10.1088/1402-4896/ac2af3
- Roy S. Non-uniform mass transfer or wall enthalpy into a compressible flow over yawed cylinder. Int J Heat Mass Transf. 2001;44(16):3017–3024. doi:10.1016/S0017-9310(00)00355-0
- Roy S, Saikrishnan P. Non-uniform slot injection (suction) into water boundary layer flow past yawed cylinder. Int J Eng Sci. 2004;42(19-20):2147–2157. doi:10.1016/j.ijengsci.2003.12.008
- Thakur A, Liu X, Marshall JS. Wake flow of single and multiple yawed cylinders. J Fluids Eng. 2004;126(5):861–870. doi:10.1115/1.1792276
- Revathi G, Saikrishnan P, Chamkha AJ. Nonsimilar solutions for unsteady flow over a yawed cylinder with non-uniform mass transfer through a slot. Ain Shams Eng J. 2014;5(4):1199–1206. doi:10.1016/j.asej.2014.04.009
- Patil PM, Shashikant A, Roy S, et al. Mixed convection flow past a yawed cylinder. Int Commun Heat Mass Transf. 2020;114:104582. doi:10.1016/j.icheatmasstransfer.2020.104582
- Patil PM, Roy S. Corrigendum of “Mixed convection flow past a yawed cylinder” [ICHMT 114 (2020) 104582]. Int Commun Heat Mass Transf. 2021;124:105246. doi:10.1016/j.icheatmasstransfer.2021.105246
- Schlichting H, Gersten K. Boundary layer theory. Berlin Heidelberg: Springer; 2000.
- Öztop HF, Cosanay H, Selimefendigil F, et al. Analysis of melting of phase change material block inserted to an open cavity. Int Commun Heat Mass Transf. 2022;137:106240.
- Ugurlubilek N, Sert Z, Selimefendigil F, et al. 3D laminar natural convection in a cubical enclosure with gradually changing partitions. Int Commun Heat Mass Transf. 2022;133:105932.
- Radbill RJ, McCue AG. Quasilinearization and nonlinear problems in fluid and orbital mechanics. New York: Elsevier Publishing Co.; 1970.
- Bellman RE, Kalaba RE. Quasilinearization and nonlinear boundary value problems. New York: Elsevier Publishing Co.; 1965.
- Patil PM. Effects of free convection on the oscillatory flow of a polar fluid through a porous medium in the presence of variable wall heat flux. J Eng Phys Thermophys. 2008;81(5):905–922.
- Patil PM, Roy S, Pop I. Flow and heat transfer over a moving vertical plate in a parallel free stream: role of internal heat generation or absorption. Chem Eng Commun. 2012;199(5):658–672.
- Inouye K, Tate A. Finite-difference version of quasi-linearization applied to boundary-layer equations. AIAA J. 1974;12(4):558–560. doi:10.2514/3.49286
- Patil PM, Roy S, Momoniat E. Thermal diffusion and diffusion-thermo effects on mixed convection from an exponentially impermeable stretching surface. Int J Heat Mass Transf. 2016;100:482–489.
- Patil PM, Goudar B. Influence of activation energy on triple diffusive entropy optimized time-dependent quadratic mixed convective magnetized flow. J Taibah Univ Sci. 2022;16(1):689–702.
- Varga RS. Matrix iterative analysis. Prentice-Hall: Hoboken; 2000. p. 219–223.
- Roy S, Anilkumar D. Unsteady mixed convection from a moving vertical slender cylinder. J Heat Transf. 2006;128(4):368–373. doi:10.1115/1.2165206
- Takhar HS, Chamkha AJ, Nath G. Combined heat and mass transfer along a vertical moving cylinder with a free stream. Heat Mass Transf. 2000;36(3):237–246. doi:10.1007/s002310050391
- Benkhedda M, Boufendi T, Touahri S. Laminar mixed convective heat transfer enhancement by using Ag-TiO2-water hybrid nanofluid in a heated horizontal annulus. Heat Mass Transf. 2018;54(9):2799–2814. doi:10.1007/s00231-018-2302-x
- Acharya N, Das K, Kumar Kundu PK. Ramification of variable thickness on MHD TiO2 and Ag nanofluid flow over a slendering stretching sheet using NDM. Eur Phys J Plus. 2016;131(9):303. doi:10.1140/epjp/i2016-16303-4
- Ghadikolaei SS, Gholinia M. 3D mixed convection MHD flow of GO-MoS2 hybrid nanoparticles in H2O–(CH2OH)2 hybrid base fluid under the effect of H2 bond. Int Commun Heat Mass Transf. 2020;110:104371. doi:10.1016/j.icheatmasstransfer.2019.104371
- Lin Y, Li B, Zheng L, et al. Particle shape and radiation effects on Marangoni boundary layer flow and heat transfer of copper-water nanofluid driven by an exponential temperature. Powder Technol. 2016;301:379–386. doi:10.1016/j.powtec.2016.06.029