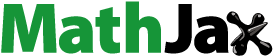
Abstract
New oblique fins with corrugated edges to incorporate into the rectangular channel have been suggested in current work to boost heat transfer. Current investigation aims to introduce and analyze an oblique-finned corrugated (OCF) to numerically evaluate a unique method for improving heat transfer. Vertical gap ratios (V) of 0.15, 0.20, and 0.25; horizontal gap ratios (H) of 0.03, 0.04, and 0.05; and oblique-angle (θ) values of 10°, 20°, and 40° are just a few of the design factors that are examined. The results show that the Nusselt number enhances by 73.5%, 74%, and 73.2% across the same range of Reynolds number at a group of gap ratios (V = 0.15, h = 0.04), (V = 0.2, h = 0.05), and (V = 0.25, h = 0.05), respectively. Besides, the design parameters V = 0.15, H = 0.05, and θ = 40° yielded the best PEC of 2.437. New correlations for the innovative oblique-finned corrugated model are also presented.
1. Introduction
Optimizing heat transfer devices has gained momentum in recent decades due to a focus on reducing resource use and utility costs [Citation1]. Improvements in heat transfer are particularly important in thermal devices because they allow for smaller sizes, higher efficiencies, and lower pumping powers [Citation2]. Scientists and engineers have therefore developed a number of active and passive techniques to improve heat transfer [Citation3]. Extended surfaces, or fins [Citation4], are frequently applied in industrial settings as a passive strategy to boost the rate of heat transfer. Rectangular fins are more common because they are easy to make and efficient, despite the fact that there are several types of extended surfaces with varying geometries [Citation5]. Numerous preceding articles have covered the topic of rectangular fins, as well as the fluid flow and heat transfer around them [Citation6].
Six different designs for the inside fin structure were analyzed by Garud et al. [Citation7] using both computational and experimental methods. The study found that the system's complete performance topped that of competing designs regarding overall efficiency and net power production by 1.88% and 35%, respectively. Grids of rectangular fins were modelled using computational fluid dynamics by Hosseini et al. [Citation8]. Their research proved that increasing the effectiveness of solar air heaters could be as simple as using longitudinal rectangular fins with appropriately spaced gaps. To optimize fin geometry for optimal heat transfer, Cong et al. [Citation9] devised a method based on the constructional design. They uncovered ways to boost thermal efficiency with unconventional fin geometry. Two new fin arrangements for cylinder heat exchangers utilized in thermoelectric producing systems were introduced and analyzed by Wang and Ma [Citation10]. The research revealed that the proposed fin structures may effectively boost the total performance. The thermal efficiency of a fin was established by Zhao et al. [Citation11] using numerical simulations. The study optimized the fin design to boost the thermal conductivity of the housing.
By simulating a wide variety of thermoelectric generator designs, Leontyev et al. [Citation12] disclosed that the use of fins inclined away from the axis of the thermoelectric generator offers the maximum increase in heat flow. Rabani and Rabani [Citation13] ran an experiment to measure how adding an array of rectangular thermal fins. Researchers discovered that installing the fin array within the Trombe wall led to a significant improvement in thermal performance. Numerically and using a heat exchanger with serrated fins, Li et al. [Citation14] implemented an investigation of the effect of varying fin diameters. In optimal setups, they discovered that adjusting the length of the interruption in the fins and the distance between them had the largest impact on system performance, improving it by 9.6%. The effects of the fin array with various knurling patterns on flow features were experimentally measured by Chikurde et al. [Citation15]. As a result, they found that the design of fin arrays is affected by a number of crucial geometric parameters related to knurling patterns. New designs of plate-fin-based heat sinks had their thermal features studied experimentally by Haghighi et al. [Citation16]. They found that fin form, spacing, and number all had significant effects. In a related study, Lee et al. [Citation17] examined the effects of perforated fins on a fin-tube heat exchanger's thermohydraulic performance. The outcomes indicated that the perforated fin casings exhibit a higher ratio of heat transfer to pressure decrease. Jafaryar et al. [Citation18] employed a wavy duct and two PCM layers for ventilation purposes and to model the discharging process. The utilized type of paraffin has a solidification temperature of 28 °C and, for augmentation of its thermal features, copper oxide nanoparticles. Their findings showed that dispersing CuO nanoparticles makes the time decrease by about 5.55%, and the solidification time declines by about 23.52% with the use of wavy duct. Augmentation of the thermal productivity of triplex tubes has been scrutinized with the installation of vase-shaped fins by Sheikholeslami and Jafaryar [Citation19]. SiO2 nanoparticles were utilized to expedite the process. They reported that with dispersing nanopowders, the melting time reduces by around 3.83%. Jafaryar & Sheikholeslami [Citation20] numerically used turbulators in a pipe, considering hybrid nanofluids to enhance heat transfer rate and decrease friction factor. The results revealed that the insertion of barriers causes the Nusselt number to intensify by about 28.43%, while the friction factor declines by about 3.6% with the rise of inlet velocity in the presence of barriers. In the same respect, Jafaryar and Sheikholeslami [Citation21] employed multi-helical tapes within the pipe of a parabolic-trough collector. A mixture of hybrid nanoparticles (Al2O3 and MWCNT) and oil was applied as the operating fluid. They claimed that the Nusselt number increased by about 10.17% and the friction factor increased by around 179.57% due to using their technique.
Azadi et al. [Citation22] analyzed the nanofluid flow and heat transfer in the mini-channel with finned surface and reported that the sinusoidal, triangular, square, and trapezoidal mini-fins generate up to 66.23%, 61.87%, 59.21%, and 57.80%, respectively, lower thermal irreversibility as compared with the smooth mini-channel. Akbarzadeh et al. [Citation23] studied thermal performance and entropy generation for water in a square heat exchanger duct equipped with several transverse twisted baffles to obtain optimal working conditions. The results of the study showed that thermal entropy generation decreases by about 210% using transversely twisted baffles in comparison with an empty duct. Esmaeili & Rashidi [Citation24] studied nanofluid flow through a 3D channel equipped with perforated transverse twisted-baffles. Due to their technique, the pressure loss decreased by 1.3% and the heat transport coefficient increased by about 3.9% as compared to the empty channel. Shamsabadi et al. [Citation25] performed a numerical simulation to investigate thermal and viscous irreversibility for Al2O3-water nanofluid inside a duct equipped with porous baffles. The results indicated that the viscous and thermal entropy generations decrease by increasing the number of baffles.
Jalili et al. [Citation26] studied convection heat transfer in a countercurrent double-tube heat exchanger with various fins in a turbulent flow. They confirmed that heat exchangers with a rectangular or curved fin have 81% and 85% better efficiency than those without a fin, respectively. Jalili et al. [Citation27] studied numerically a shell and tube heat exchanger with non-continuous helical baffles. They reported that the maximum heat transfer occurs at a helix angle of 20° and the optimum pressure drop occurs at a helix angle of 40°. In another respect, Jalili et al. [Citation28] concluded that the heat transmission rate at the lower penetrable circle consistently diminishes within the nearness of slip as the Reynolds number increments between two porous discs in the outer magnetic amplitude. Micro-polar nanofluid between two parallel plates in a rotating system with electric and magnetic fields was examined by Jalili et al. [Citation29]. The findings demonstrate that as the magnetic parameter rose, the velocity profile and micro-rotation velocity also increased. Increasing the rotation parameter also increases the velocity. In the same way, Jalili et al. [Citation30] employed semi-analytical techniques to solve the heat and mass transfer problem and reported that the proposed method has high accuracy and efficiency and can be used in many engineering and thermal problems that have nonlinear differential equations. Jalili et al. [Citation31] investigated the effect of the number of trial functions on the precision of the answers and recommended considering other semi-analytical methods to solve such a problem.
Seven different geometric parameters of staggered offset fins were utilized and analyzed by Du et al. [Citation32] in the investigation. The HEX was operated at different intake velocities to simulate a dual flow. After comparing the new design to the old, the authors found that it was 40% more effective at handling hydraulic loads. Liu et al. [Citation33] used numerical simulations with different flue gas mass fluxes to examine how the form and clearance of pin fins affected the efficiency of the devices. Results showed that the fin design with a 4 mm tip clearance outperformed the drop module in terms of thermo-hydraulic efficiency. Fin design was also considered by Xue et al. [Citation34], who used three distinct wavy plate fin HEX configurations to examine the effect at varying Reynolds number. At specific parameters, the simulation results showed that the proposed design was 1.24 times more efficient than the conventional and alternative wave fin designs. By conducting experiments, Unger et al. [Citation35] proposed new fin designs to improve the heat transfer for the system under consideration. Also, Ibrahim et al. [Citation36] evaluated the effect of using the square perforation technique to improve the thermal behaviour of heat sinks both experimentally and numerically. According to the study, the presence of holes decreased the amount of friction around the fin, which in turn lowered the amount of flow pumping power needed.
Based on the above survey, previous studied has established that fins with different preparation has a considerable impact on the thermal-hydraulic performance of many applications of thermal devices. However, there has been no published study on oblique-corrugated fins (OCF) or their use to improve heat transmission in HEX systems. More efficient methods for making and arranging oblique-corrugated fins are detailed here. Because of the individual nature of each OCF, they are all crafted with somewhat different deviations and sizing. These OCFs were designed to increase the system's PEC by producing a more uniform and appropriate temperature distribution. To better transfer heat, a novel form of heat exchanger was developed using oblique-corrugated fin structures.
2. Description of physical model
Figure depicts a model of the OCFs system. For simplicity's sake, let's just say that the geometry of this investigation is a symmetrical rectangle with identical top, bottom, inlet, outlet, and side walls. The proportional ratio of the model's length (L) to its breadth (W) is 5:1. Several oblique-corrugated fins have been designed on the model put to the test. The fins were of rectangular cross-sections. Both the top and bottom fin walls feature a triangular corrugation pattern in this investigation. For a fair comparison of the performance of the different designs of OCF systems, new parameters such as vertical gap ratios (V = v/W), horizontal gap ratios (H = h/L), and attack angles (θ) were defined in the current study. The vertical gap ratio, which is defined as the vertical distance between OCFs, includes three ratios: 0.15, 0.2, and 0.25. The horizontal gap ratio, which is defined as the horizontal distance between OCFs, includes three ratios: 0.03, 0.04, and 0.05. Attack angles (θ) represent the angle of inclination of the corrugated fins from the horizontal axis and also include three angles: 10°, 20°, and 40° (Table shows the geometric parameters). The standard case, which is comparable to commercial HEX, served as the basis for these parameter values. The researchers also account for the potential for manufacture. For instance, producing very small oblique-corrugated fins is challenging. In order to establish the values of these parameters, 25% of the standard case was added or subtracted.
Table 1. Design parameters of OCF.
A steady-state with constant thermophysical properties has been considered for incompressible air. Three-dimensional has been adopted in the current simulation as all simulations are done with a Re of between 10000 and 50000. Stability has been assumed in the studied velocity range, and the flow could be turbulent and fully developed. Besides, through a large temperature range, the thermal conductivity of walls and fins stays constant.
The computational domain of the model under consideration is constrained by the adopted boundary conditions. The computational domain's inlet, outlet, upper wall, bottom wall, and symmetry wall are all subject to boundary conditions. The current study employed air as a working fluid. The targeted channel's top and bottom walls are forced to have a constant temperature of 300 K, while the working fluid's entrance temperature is 673 K.
3. Governing equations
The complex flow in the current model is explained by the three-dimensional governing equations shown below:
Continuity equation [Citation37]:
(1)
(1)
Momentum equation [Citation37,Citation38]:
(2)
(2)
Then, energy equation [Citation38,Citation39]:
(3)
(3)
Then, Reynolds stresses equations [Citation40]:
(4)
(4)
(5)
(5)
The corresponding transport equations for turbulence kinetic energy and energy dissipation in the standard k-ϵ model are presented below [Citation40]:
(6)
(6)
(7)
(7)
Equation of turbulence flow viscosity [Citation39,Citation40]:
(8)
(8)
Also:
Where
,
,
,
, and
are constants.
Then, several important parameters are described as shown below [Citation40]:
(11)
(11)
(12)
(12)
(13)
(13)
(14)
(14)
(15)
(15)
Augmenting the heat transfer rate of oblique-corrugated fins due to the corrugations is proven by the PEC parameter. This measurement has the potential to be used to evaluate the thermal-hydraulic performance factor of modified fins against smooth fins (without corrugations) [Citation40]:
(16)
(16)
4. Computation domain solution
In the current study, the commercial computational fluid dynamics (CFD) software package (ANSYS-FLUENT-V19) is used to solve the governing equations in a Cartesian coordinate system based on the finite volume method (FVM) with corresponding boundary conditions [Citation40]. Therefore, the numerical calculations are performed using FVM by ANSYS Fluent as well as the κ-ϵ model. Additionally, the second-order upwind method that employs the SIMPLE algorithm's convective term, pressure and velocity coupling, is used. The flow equations’ convergence threshold is 10−6, while the energy equation's is 10−10, respectively. Using a computer with special specifications, the convergence is attained after 550 iterations, with each taking an average of 10 min. The grid independence test for the current investigation is shown in Figure .
For a numerical simulation to produce accurate results, a trustworthy grid must be built. To achieve the best accuracy in estimating the flow characteristics while reducing the computational cost, mesh analysis and selecting an appropriate grid are therefore important. To evaluate the independence from the grid size, five sets of grids, including 1086283, 1176785, 1208818, 1259777, and 1301970, were taken into consideration. Here, the mesh is fine-tuned until the changes of the average Nusselt number become negligible in order to choose the best grid for a sample corrugated fin, as depicted in Figure . The stability of the mesh numbers 1208818, 1259777, and 1301970 was then observed by comparing the results for three Reynolds number values, such as 10000, 30000, and 50000, with the maximum difference within 1%. Therefore, with a cell size of 0.025 mm, a mesh total of 1208818 is used to reduce processing time. The thickness of the first layer of the boundary layer mesh is specifically determined so that the value of y + meets the requirements of the turbulence model. However, the y + values are 0.05 and 3 for the lowest and highest Reynolds numbers, respectively, implying that the wall model will not influence the predictions to any noticeable degree. Figure shows the generated mesh of proposed channel.
Figure 3. Generated mesh of proposed channel and validation of the current numerical result with (a) Unger et al. [Citation35], and (b) Ibrahim et al. [Citation36].
![Figure 3. Generated mesh of proposed channel and validation of the current numerical result with (a) Unger et al. [Citation35], and (b) Ibrahim et al. [Citation36].](/cms/asset/e2229b2c-dafa-4bec-9bd3-4cb0d0cda85a/tusc_a_2234705_f0003_oc.jpg)
Both the circular plain fins and the non-perforated fins served as validation examples for the current numerical modelling. To validate the numerical approach described for oblique-corrugated fins, Unger et al. [Citation35]'s and Ibrahim et al. [Citation36]'s experimental studies were used. The comparison was done for both the 11 and 16 mm fin spacing of the circular plain fins in the first code with Unger et al. [Citation35]. The Figure (a) demonstrates that the numerical findings correspond well with the experimental data within a satisfactory margin of error. Nevertheless, the maximum deviation was 3.52- 8.7% across the consider range of Reynolds number.
Regarding the second code validation, non-perforated fins with Re between 10000 and 80000 have also been taken into account. Figure (b) displays the comparability between the Nusselt numbers of the present study with the experimental Nusselt numbers obtained from Ibrahim et al. [Citation36]. The maximum error obtained was with the experimental value and which is about 4.2–8.7%
Figure shows that the current results match up well with the experimental results of other researchers [Citation35,Citation36]. This shows that the current numerical approach to studying oblique-corrugated fins is acceptable.
5. Results and discussion
The effects of various triangular oblique-corrugated fin parameters on the turbulent airflow and thermal performance of a HEX system were investigated in this study.
As illustrated in Figure , the velocity, isotherms, turbulent kinetic energy, and vorticity contours were used to investigate and address the flow characteristics of air as a working fluid. This figure demonstrates how the proposed model of fin modifies the path of the main flow, letting the minor flow to swirl, which is thought to be the main driver of an increase in heat transfer. Temperature contours show that the employed new technique and their triangular corrugations often influence the temperature zones along channel walls. In the case of without corrugations, minor flows are created close to the edges, whereas in the case of corrugations, minor flows are recognized close to both the corrugations as well as edges of the fins. Subsequently, because the oblique fin of a triangular corrugation creates another flow in the opposite direction of the main flow, the resulting flow is more effective. Therefore, oblique fins with triangular corrugations have a thinner thermal boundary layer than oblique fins without corrugations. Convection heat transfer is more effective when flow mixing is increased and the boundary layer thickness is decreased. So, compared to oblique fins without corrugations, those with corrugations are expected to improve heat transfer the most.
Figure 4. Comparison between channel with an oblique fin without corrugation (right) and the other with triangular oblique-corrugated fin (left) in terms of various contours: (a) Isotherms, (b) Velocity, (c) Turbulent kinetic energy, and (d) Vortices contours, at Re = 10000.

The above explanations for velocity and isotherms contours make the explanation of turbulent kinetic energy contours simpler. The new model of fin (with corrugation) produces higher turbulence and velocity than those without corrugations, as shown by the given examples’ velocity contours. These methods produce secondary whirling flows as a result, which creates a rather long flow path in the tested model, which significantly improves heat transfer. This means that oblique-fins and corrugations greatly enhance thermal performance. Also, the pressure drop penalty also increases. Accordingly, the cases with triangular oblique-corrugated fins outperformed the cases without corrugations in terms of turbulent energy.
Another contour is the vorticity contour, which is thought to have the main influence on changes in the features of the earlier contours. The vorticity contours’ flow pattern showed a remarkable agreement with the turbulent kinetic energy contours. The created vortices considerably contribute in improving heat exchange since they have the favourable impact of boosting the air mixing. The main hypothesis is that the development of vortices has a major impact on the measured channel's thermal performance, which improves as vortices are produced. As was already mentioned, the presence of fins and corrugations significantly affects the vorticity's shape. This indicates that when compared to models without corrugations, the vorticities produced by the model with triangular oblique-corrugated fins are the largest. This implies that the use of triangular, oblique-corrugated fins clearly improves heat transfer.
Figure depicts transverse plane vorticity contours and isotherms superimposed with temperature maps at the same locations for H = 0.03, 0.04, and 0.05, respectively. In general, the proposed fin models (with corrugations) generate multiple vortices. These vortices are essential for the cooling of the working fluid as a result of their role in fostering the mixing of air in the channel's centre and adjacent to the wall. When H = 0.03, the number of fins in a given area increases, indicating that the horizontal distance between them is minimal and they are relatively near together. At H = 0.04 and 0.05, one can observe how vortices surge along the interior of the channel and interact with vortices generated by the walls. Consequently, when H is small, air mixing closes the wall is enhanced.
Figure 5. Isotherms and vortices contours of tested model with triangular oblique-corrugated fin under different horizontal gap ratios.

Figure (a, b) depicts the association between the average Nusselt number, friction factor, and Reynolds number at V = 0.15 and different H such as 0.03, 0.04, and 0.05. The model without corrugations has the lowest performance for both heat transfer and friction losses, which is an extremely significant point to make. As we turn to other cases, the findings show that the Nusselt number continuously rose with a growing Reynolds number, whereas the friction factor fell with this rise. Besides, Nusselt number drops with increasing magnitude of the horizontal gap in terms of dimensional interaction as a result of the drop in temperature brought on by improper air mixing. The friction factor acts similarly to the Nusselt number in that it lowers with rising H values. Therefore, the best heat transfer was seen at H = 0.03, which was also superior to others regarding friction factor. From a physical point of view, this increase in the Nusselt number or the decrease in the friction factor depends on the shape of the flow generated inside the channel by the effect of the extended corrugated surface of the oblique fins that were applied in this study.
Figure 6. Impacts of various horizontal gap ratios (H) on the heat transfer characteristics of triangular oblique-corrugated fin at oblique angle 40 and vertical gap ratio 0.15: (a) Average Nu, (b) Friction factor, and (c) PEC.

The association between the Reynolds number and PEC for different H ratios at 40° and V = 0.15 is shown in Figure (c). The PEC drastically augments as Re augments until it reaches 30000, at which point it nearly immediately increases again until it reaches its best rate at Re = 5000. PEC fell as H dropped. The model without extended surfaces had the lowest PEC, whereas optimal outcomes were achieved with the horizontal gap ratio set to its greatest value (0.05). This might be attributed to frictional loss, which can reduce the system's performance and hence the heat transfer's impact.
Figure (a, b) shows the association between the Reynolds number, average Nusselt number, and friction factor for a V of 0.2 and various H of 0.03, 0.04, and 0.05. It is vital to keep in mind that the models with smooth walls and no corrugated fins suffer from the least amount of heat transfer and friction. This shows the effectiveness of the proposed techniques applied in this inquiry. Also, the results show that when the Reynolds number is augmented, the friction factor goes down, but the Nu constantly increases. Due to the temperature drop caused by inadequate air mixing, it is obvious that the Nusselt number declines as the H grows in terms of dimensional interaction. High Reynolds numbers (more than 30,000) have a more noticeable effect, while low Reynolds numbers have almost no effect (less than 30,000). As the H rises, the friction factor reduces due to an increase in the distance between the fins and, therefore, a decrease in friction and obstructions in the flow path.
Figure 7. Impacts of various horizontal gap ratios (H) on the heat transfer characteristics of triangular oblique-corrugated fin at oblique angle 40 and vertical gap ratio 0.2: (a) Average Nu, (b) Friction factor, and (c) PEC.

Figure (c) illustrates the association between the PEC and Re for a range of H at 40° and V = 0.2. It is evident that the PEC grows considerably as Re grows until it reaches 30000, at which time it almost doubles until it reaches its best rate at Re = 5000. Also, as H declines, PEC declines. In general, the model with the biggest H produced the best results, while the model without corrugations had the lowest PEC (0.05). This behaviour can be accounted for by frictional loss, which has the potential to impair the model's overall performance by reducing the amount of heat transfer that occurs.
The average Nusselt number, friction factor, and Reynolds number are shown in relation to each other in Figure (a, b) for V = 0.25 and varied H of 0.03, 0.04, and 0.05. It is important to note that, when compared to other models, smooth models and models without corrugated fins have the smallest heat transfer and friction losses. This proves the potency of the proposed procedures devoted to this analysis. The statistics show that as the Re climbed, the Nu expanded steadily as the friction factor declined, expanding the investigation to additional tested models. Additionally, it follows that the Nu falls as the H rises because of the temperature reduction brought on by inadequate air mixing (both paths of fluid). This impact is less noticeable at low Re but more noticeable at high Re (greater than 30,000). (less than 30,000). Because the flow experiences less frictional resistance versus the solid edges of the model and around any blocks, the friction factor falls, much as the Nu does, as the H increases. Thus, the ratio of 0.0.03 presented the best heat transfer and performed better than the other values under consideration.
Figure 8. Impacts of various horizontal gap ratios (H) on the heat transfer characteristics of triangular oblique-corrugated fin at oblique angle 40 and vertical gap ratio 0.25: (a) Average Nu, (b) Friction factor, and (c) PEC.

Figure (c) illustrates the relationship between the Reynolds number and the PEC for various H at V = 0.25 40°. PEC increases dramatically as Reynolds number increases up to a point where it nearly doubles, reaching its maximum value at Re = 5000. In addition, PEC decreases when H decreases. The model without an extended surface had the lowest PEC, but the model with the highest H yielded the greatest results (0.05). This behaviour is attributable to frictional loss, which can negatively influence the model's overall performance and reduce heat transfer.
Concerning the second parameter, it has been investigated how vertical gap ratios influence the flow and heat transfer characteristics. Figure depicts isotherms at the same locations in the new model for V = 0.15, 0.20, and 0.25, with temperature maps and vorticity contours superimposed. Typically, corrugated fins produce numerous vortices, one at the margin of each fin and another in the space between fins that wall horizontally. These vortices are essential for cooling the hot air due to their function in facilitating the blending of the air in the core of the proposed model and those close to the walls. When V = 0.15, when the distance between fins is very small and they are relatively near together, the number of fins in a given area increases. Vortices are more evident at the corners of the fins; however, at somewhat lower V = 0.2 and 0.25, one can observe how vortices rush along the channel's interior. This integration, which is fundamentally responsible for the cooling working fluid at V = 0.15 due to greater air mixing near the wall, appears to be quite extensive and obvious when V is low.
Figure 9. Isotherms and vortices contours of tested model with triangular oblique-corrugated fin under different vertical gap ratios.

In Figure , the Nusselt number, friction ratio, and PEC are plotted versus Re for the investigated models with V values of 0.15, 0.2, and 0.25 and H = 0.03. It appears that as Re increases, heat transfer increases while friction decreases. According to the results, V = 0.15 has the maximum Nusselt number and friction factor, followed by V = 0.2 and 0.25, respectively. Figure (a) reveals that, in comparison to models with uniform channels, all instances of oblique fins with corrugations result in enhanced heat transfer. This is demonstrated by the fact that the air mixing within the channel with the corrugated fin improved at specific Reynolds values. V = 0.2, another crucial factor, has slowed in relation to Nu and friction factor. Figure b demonstrates that, similar to the previous trend, the model without an extended surface has the lowest friction factor when compared to the others. In terms of heat transmission, the proposed models with a rectangular cross section may be superior to the smooth model. Consequently, this finding indicates that the inert strategy recommended by the suggested model is preferable to the slandered model. As depicted in Figure (c), the analysed PEC reveals that the proposed model with V = 0.2 demonstrates the highest performance in all circumstances. In addition, the increase in PEC was rapid up until Re = 3000, after which it scarcely increased. This suggests that Reynolds numbers influence PEC outcomes within a given range. Due to the effects of the oblique-corrugated fins, the smooth channel typically provides the lowest PEC compared to others.
Figure 10. Impacts of various vertical gap ratios (V) on the heat transfer characteristics of triangular oblique-corrugated fin at oblique angle 40 and horizontal gap ratio 0.03: (a) Average Nu, (b) Friction factor, and (c) PEC.

Nusselt number, friction ratio, and PEC are plotted against Reynolds number in Figure for models with differing vertical gaps of 0.15, 0.2, and 0.25 and H = 0.04. Evidently, as Re increases, heat transfer increases and the friction factor decrease. The results indicate that V = 0.15 has the maximum Nusselt number and friction ratio, followed by V = 0.2 and V = 0.25. Figure (a) reveals that, in comparison to a rectangular fin without any extended surfaces, all proposed models exhibit enhanced heat transfer. Notable also is the fact that the Nusselt number and friction factor are greatest at V = 0.2. As shown in Figure (b), the Nusselt number and friction factor rate followed a similar trajectory, with the smooth model having the lowest value. Based on the friction factor rate, the novel model at V = 0.2 has the greatest losses, a low inclination, and a high Reynolds number under all conditions. As shown in Figure .c, the proposed model at V = 0.2 exhibits the highest performance under all conditions. In addition, the PEC rose rapidly until Re = 3000, after which it rose gradually. This indicates that Re influences PEC within a certain range. Due to the influence of the oblique-corrugated fins, the model with no extended surfaces has, on average, the lowest PEC among all models.
Figure 11. Impacts of various vertical gap ratios (V) on the heat transfer characteristics of triangular oblique-corrugated fin at oblique angle 40 and horizontal gap ratio 0.04: (a) Average Nu, (b) Friction factor, and (c) PEC.

Figure shows the Nusselt number, friction ratio, and PEC vs. Reynolds number for the investigated models at different values of V = 0.15, 0.2, and 0.25 and H = 0.05. It seems that when the Re goes up, the heat transfer goes up but the friction factor goes down. The outcomes show that V = 0.15 is deemed to have the highest Nusselt number and friction ratio, and then V = 0.2 and 0.25, respectively. A closer look at Figure (a) demonstrates that, when compared to smooth models, all models with the new technique have improved in terms of heat transfer enhancement. This is supported by the observation that when the Reynolds number was changed, the mixing of air through the proposed model improved. Additionally, the best rate regarding friction factor was reported for V = 0.20. The model without extended surfaces recorded the lowest friction factor rate when compared to other models, according to Figure (b), which follows a similar trend to Nusselt's number. When V = 0.2, the suggested model provides the biggest losses, and it has a low inclination with a high Re in all circumstances, based on the rate of friction factor. The model at V = 0.2 with a high Re in all models performs the best, according to the PEC, as shown in Figure (c). Furthermore, the rise in PEC was rapid until Re = 3000, after which it just barely rose. This implies that Reynolds number influences PEC outcomes within a given range. Because of the impacts of the oblique-corrugated fins, the smooth model generally offers the lowest PEC when compared to the other investigated models.
Figure 12. Impacts of various vertical gap ratios (V) on the heat transfer characteristics of triangular oblique-corrugated fin at oblique angle 40 and horizontal gap ratio 0.05: (a) Average Nu, (b) Friction factor, and (c) PEC.

Based on the above findings, various V and H gaps have been compared, depending on the best PEC. Then, three ratios have been established to discuss the best one, such as (V = 0.15, h = 0.03), (V = 0.15, h = 0.04), and (V = 0.15, h = 0.05). Figure shows Nu, friction factor, and PEC against Re. It is obvious that as the Re rises, the Nu does too, but the friction factor falls. The findings indicate that a small V ratio has a bigger impact than others on both the rate of heat transfer and friction losses. Thus, regarding Nu and friction factor, the model with V = 0.15 and h = 0.03 offered the highest rate. The excellent air mixing, as previously mentioned, is the cause of this.
Figure 13. Comparison of various vertical and horizontal gap ratios on the heat transfer characteristics, (a) Average Nu, (b) Friction factor, and (c) PEC.

As seen, for all gaps considered in this work, the Nusselt number tends to rise as the Reynolds number rises. For instance, at the gaps of (V = 0.15, h = 0.04), (V = 0.2, h = 0.05), and (V = 0.25, h = 0.05), respectively, the Nusselt number grew by 73.5%, 74%, and 73.2% as the Reynolds number increased from 10,000–50,000. Also, the horizontal gap ratios have a considerable effect on the Nusselt number of the tested model. For instance, when H decreases from 0.05–0.04, the Nusselt number of the model increases by around 4.8%, whereas it increases by approximately 4.3% when H decreases from 0.05–0.03, across the same range of Reynolds number. Besides, the small vertical gap ratio was bigger than the others, but as the Reynolds number grew, the friction factor decreased, indicating a preference for a large gap ratio in terms of friction losses.
The model with the high horizontal gap, H = 0.05, exhibits greater performance, and the factor that measures thermal-hydraulic performance shows this. The factor shows a rapid increase until Re = 30,000 for all cases, and then a little increase until it achieves the best rate at Re = 50,000. For instance, the thermal-hydraulic performance factor of a model with H = 0.03, H = 0.04, and H = 0.05 increases by around 13.2%, 13.4%, and 13.7%, respectively, when the Reynolds number goes from 10000 to 30000. In contrast, the thermal-hydraulic performance factor of a model with H = 0.03, H = 0.04, and H = 0.05 increases by roughly 2.5%, 2.6%, and 2.7%, respectively, when the Reynolds number is increased from 30000 to 50000. As a result, when compared to other models, the model with H = 0.05 is the best. The principal flow velocity dramatically increases with rising Reynolds numbers, and the turbulent flow eventually takes on the role of the main driving force for increasing heat transfer. As a result, as Re grew, the thermal-hydraulic performance factor rose for all evaluated models with various vertical and horizontal gaps. It is interesting to notice that the thermal-hydraulic performance factor value for H = 0.05 was the highest compared to others at the maximum Re, or 50,000. At design parameters of H = 0.05, V = 0.15, and 40°, the best thermal-hydraulic performance factor of 2.437 has typically been observed.
The attack angles of the oblique-corrugated fin include 10, 20, and 40, which are considered the third parameter in this investigation, having been tested to determine a PEC factor for the proposed models. Figure shows that while the friction factor declines as the Re rises, the Nusselt number rises. According to the research, a larger angle has a bigger impact on friction losses and heat transfer rate than a smaller angle. In other words, the angle of 40° had the highest rate for both Nu and friction factor. Again, the excellent air mixing, as previously mentioned, is the cause of this. The Nusselt number tends to rise as the Reynolds number of all angles under investigation rises, as seen in the figure. For instance, the Nusselt number climbed by 74.0%, 72.0%, and 70.0% for angles of 40°, 20°, and 10°, respectively, when the Re increased from 10,000–50,000. Raising the angles also has a considerable impact on the tested model's Nusselt number. For instance, the Nusselt number increases by roughly 69.0% when the angle is raised to 40° and by roughly 17.0% when the angle is raised to 20°. The huge angle, however, was greater than the others, and the friction factor reduced as the Reynolds number climbed, suggesting that a small angle is preferable regarding pressure and friction losses. The proposed style with the greatest angle, 40°, performs better, as shown by the analysed PEC, which shows a sharp increase in all scenarios up to Re = 30,000 and then a gradual increase up to the best rate at Re = 50,000. For instance, the PEC of channels with 40°, 20°, and 10° boosts by roughly 14.0%, 19.0%, and 12.0%, respectively, as the Re goes from 10000 to 30000. In contrast, the PEC of channels with angles of 40°, 20°, and 10° increases by roughly 2.5%, 4.0%, and 1.2%, respectively, when the Reynolds number is increased from 30000 to 50000. The principal flow velocity dramatically increases with rising Reynolds numbers, and the turbulent flow eventually takes on the role of the main driving force for increasing heat transfer. A similar tendency emerged when the angle of the suggested model was augmented. As a result, the PEC rose at all tested angles with different V and H gaps as the Reynolds number increased. It's important to note that the PEC value at 40° was higher than the others at the greatest Reynolds number, 50,000.
Figure 14. Impacts of oblique-corrugated fin angles on the heat transfer characteristics, (a) Average Nu, (b) Friction factor, and (c) PEC.

The authors used current data to propose a correlation equation in terms of Nusselt number (Nu) and friction factor (f) in the range between Re 10,000 and 50,000 based on the significant findings of all geometrical parameters under consideration. To fit the computational simulation data, the exponent constants are calculated using SSPS's multi-regression technique. R2 determination coefficients for correlations are 0.95 and 0.93, respectively. As shown in Figure , the relative errors for the Nu are less than 6% and for the f they are less than 7%.
(17)
(17)
(18)
(18)
6. Conclusion
This study investigates the thermo-hydraulic performance of a new oblique fin, namely, oblique-finned corrugated (OCF). The OFC has been made inside a rectangular channel with different geometrical parameters and a wide range of Reynolds numbers. The proposed systems are subjected to turbulent air flows that are simulated using the finite volume technique (FVM) and the κ-ϵ model under constant temperature circumstances (T = 300 K). The following conclusions from the study can be drawn:
Compared to others, introducing the new style of oblique-corrugated fins (OCF) has a significant impact on changing the flow structure, which consequently leads to augmenting the thermal-hydraulic performance factor.
While friction loss decreased as the Reynolds number increased, the rate of heat transfer increased as the Reynolds number increased.
The proposed model with the modest vertical gap, V = 0.2, performs better, with a significant improvement up to Re = 30,000 for all cases, followed by a minor improvement up to Re = 50,000, where it reaches its best rate.
OCF is superior to the unmodified model, and the PEC rates were highest at pitch angles of 40°, followed by 20° and 10°.
The Nusselt number increases by approximately 69.0% when the angle is increased from 20° to 40° and by approximately 15.0% when the angle is increased from 20° to 40°.
The optimal PEC has been found to be 2.437 at V = 0.15, which is regarded as a small value, and H = 0.05, which is regarded as a high value.
Using OCF, new correlations for Nusselt number and friction factor are developed as functions of the fluid flow parameters and geometric properties.
Finally, it has been demonstrated that OCF could be a passive method for creating a high-performance cooling and heating system.
The conclusions of the presented work can be the basis for further research, e.g. on other extended surfaces, cooling air temperatures, channel height, spacing of corrugations, inline and offset configuration, and shape and position of OFC in the channel. As well, it is very important to replace the working fluid with others such as water, oil, and nanofluids to study the flow behaviour that will be configured inside the tested channel.
Nomenclature
= | model constants | |
= | hydraulic diameter, mm | |
f | = | Friction factor |
FVM | = | Finite volume method |
G | = | generation of turbulent kinetic energy, kg/ms3 |
h | = | Horizontal gap, mm |
= | Heat transfer coefficient, (W/m2. K) | |
H | = | Horizontal gap ratio, |
HEX | = | Heat exchangers |
= | turbulent intensity | |
L | = | Length of tested model, mm |
κ | = | turbulent kinetic energy, (m2/s2) |
k | = | conduction heat transfer coefficient, W/m. K |
Nu | = | Nusselt number |
OCF | = | oblique-corrugated fins |
p | = | pressure, Pa |
Δp | = | pressure drop, Pa |
Pr | = | Prandtl number |
PEC | = | Thermal-hydraulic performance factor |
Re | = | Reynolds number, Re = |
T | = | temperature, K |
TR OCF | = | width to the channel width ratio (0.03) |
V | = | Vertical gap, mm |
V | = | Vertical gap ratio |
U | = | velocity component, m/s |
X, y, z | = | cartesian x, y, z-coordinate |
W | = | width of tested model, mm |
Greek symbols
= | dynamic viscosity of the fluid, kg/m. s | |
= | density, kg/m3 | |
= | Turbulent kinetic dissipation, m2/s2 | |
= | Diffusion Prandtl number for k | |
= | Attack angles |
Subscripts
Av | = | average |
in | = | inlet |
m | = | mean |
mod | = | modified |
f | = | fluid |
ref | = | smooth channel |
Disclosure statement
No potential conflict of interest was reported by the author(s).
References
- Ajeel RK, Salim WI, Hasnan K. Design characteristics of symmetrical semicircle-corrugated channel on heat transfer enhancement with nanofluid. Int J Mech Sci. 2019;151:236–250. doi:10.1016/j.ijmecsci.2018.11.022
- mohammed Hussein HA, Zulkifli R, Mahmood WMFBW, et al. Structure parameters and designs and their impact on performance of different heat exchangers: A review. Renewable Sustainable Energy Rev. 2022;154:111842. doi:10.1016/j.rser.2021.111842
- Ajeel RK, Sopian K, Zulkifli R. Thermal-hydraulic performance and design parameters in a curved-corrugated channel with L-shaped baffles and nanofluid. J Energy Storage. 2021;34:101996. doi:10.1016/j.est.2020.101996
- Ajeel RK, Zulkifli R, Sopian K, et al. Numerical investigation of binary hybrid nanofluid in new configurations for curved-corrugated channel by thermal-hydraulic performance method. Powder Technol. 2021;385:144–159. doi:10.1016/j.powtec.2021.02.055
- Ajeel RK, Sopian K, Zulkifli R. A novel curved-corrugated channel model: Thermal-hydraulic performance and design parameters with nanofluid. Int. Commun. Heat Mass Transfer. 2021;120:105037. doi:10.1016/j.icheatmasstransfer.2020.105037
- Ajeel RK, Salim WI, Hasnan K. Numerical investigations of heat transfer enhancement in a house shaped-corrugated channel: combination of nanofluid and geometrical parameters. Therm Sci Eng Progr. 2020;17: 100376. doi:10.1016/j.tsep.2019.100376
- Garud KS, Seo JH, Patil MS, et al. Thermal–electrical–structural performances of hot heat exchanger with different internal fins of thermoelectric generator for low power generation application. J Therm Anal Calorim. 2021;143(1):387–419. doi:10.1007/s10973-020-09553-7
- Hosseini SS, Ramiar A, Ranjbar AA. Numerical investigation of rectangular fin geometry effect on solar chimney. Energy Build. 2017;155:296–307. doi:10.1016/j.enbuild.2017.09.017
- Cong R, Ozaki Y, Machado BS, et al. Constructal design of a rectangular fin in a mixed convective confined environment. Inventions. 2018;3(2):27. doi:10.3390/inventions3020027
- Wang T, Ma S. Thermoelectric generator heat performance study about improved fin structures. Therm Science. 2018;22(1 Part A):101–112. doi:10.2298/TSCI150801064W
- Zhao W, Mozumder AK, Das PK. (2018, July). Mixed convection of CuO-water nanofluid in a square enclosure with an intruded rectangular fin. In AIP Conference Proceedings (Vol. 1980, No. 1, p. 050004). AIP Publishing LLC.
- Leontyev AI, Onishchenko DO, Arutyunyan GA. Selecting the optimum method of heat transfer intensification to improve efficiency of thermoelectric generator. Thermophys Aeromech. 2016;23(5):747–754. doi:10.1134/S0869864316050139
- Rabani M, Rabani M. Heating performance enhancement of a new design trombe wall using rectangular thermal fin arrays: An experimental approach. J Energy Storage. 2019;24:100796. doi:10.1016/j.est.2019.100796
- Li K, Wen J, Liu Y, et al. Application of entransy theory on structure optimization of serrated fin in plate-fin heat exchanger. Appl Therm Eng. 2020;173:114809. doi:10.1016/j.applthermaleng.2019.114809
- Chikurde RC, Kothavale BS, Sane NK. (2018, November). Natural convection heat transfer with horizontal rectangular fin array using straight knurling patterns on fins: An Experimental Study. In ASME International Mechanical Engineering Congress and Exposition (Vol. 52125, p. V08BT10A007). American Society of Mechanical Engineers.
- Haghighi SS, Goshayeshi HR, Safaei MR. Natural convection heat transfer enhancement in new designs of plate-fin based heat sinks. Int J Heat Mass Transfer. 2018;125:640–647. doi:10.1016/j.ijheatmasstransfer.2018.04.122
- Lee HJ, Ryu J, Lee SH. Influence of perforated fin on flow characteristics and thermal performance in spiral finned-tube heat exchanger. Energies. 2019;12(3):556. doi:10.3390/en12030556
- Jafaryar M, Majdi HS, Basem A, et al. Heat storage modeling for air ventilation usage considering freezing of paraffin through a sinusoidal duct. J Energy Storage. 2022;55:105296. doi:10.1016/j.est.2022.105296
- Sheikholeslami M, Jafaryar M. Performance of energy storage unit equipped with vase-shaped fins including nanoparticle enhanced paraffin. J Energy Storage. 2023;58:106416. doi:10.1016/j.est.2022.106416
- Jafaryar M, Sheikholeslami M. Intensification of performance of pipe with nanoparticle flow along turbulator with obstacles. Chem Eng Process-Process Intensification. 2021;165:108426. doi:10.1016/j.cep.2021.108426
- Jafaryar M, Sheikholeslami M. Efficacy of turbulator on performance of parabolic solar collector with using hybrid nanomaterial applying numerical method. Renewable Energy. 2022;198:534–548. doi:10.1016/j.renene.2022.08.037
- Azadi M, Hosseinirad E, Hormozi F, et al. Second law analysis for nanofluid flow in mini-channel heat sink with finned surface: a study on fin geometries. J Therm Anal Calorim. 2020;140:1883–1895. doi:10.1007/s10973-019-08921-2
- Akbarzadeh M, Rashidi S, Masoodi R, et al. Effect of transverse twisted baffles on performance and irreversibilities in a duct. J Thermophys Heat Transfer. 2019;33(1):49–62. doi:10.2514/1.T5373
- Esmaeili Z, Rashidi S. Entropy production analysis for nanofluid flow through a channel with perforated transverse twisted-baffles. Energy Sources Part A. 2022: 1–20. doi:10.1080/15567036.2022.2041131
- Shamsabadi H, Rashidi S, Esfahani JA. Entropy generation analysis for nanofluid flow inside a duct equipped with porous baffles. J Therm Anal Calorim. 2019;135:1009–1019. doi:10.1007/s10973-018-7350-4
- Jalili B, Aghaee N, Jalili P, et al. Novel usage of the curved rectangular fin on the heat transfer of a double-pipe heat exchanger with a nanofluid. Case Studies Therm Eng. 2022;35:102086. doi:10.1016/j.csite.2022.102086
- Jalili P, Kazerani K, Jalili B, et al. Investigation of thermal analysis and pressure drop in non-continuous helical baffle with different helix angles and hybrid nano-particles. Case Studies Therm Eng. 2022;36:102209. doi:10.1016/j.csite.2022.102209
- Jalili B, Roshani H, Jalili P, et al. The magnetohydrodynamic flow of viscous fluid and heat transfer examination between permeable disks by AGM and FEM. Case Studies in Thermal Engineering. 2023;45:102961. doi:10.1016/j.csite.2023.102961
- Jalili P, Narimisa H, Jalili B, et al. A novel analytical approach to micro-polar nanofluid thermal analysis in the presence of thermophoresis, Brownian motion and Hall currents. Soft comput. 2023;27(2):677–689. doi:10.1007/s00500-022-07643-2
- Jalili P, Azar AA, Jalili B, et al. Heat transfer analysis in cylindrical polar system with magnetic field: A novel hybrid analytical and numerical technique. Case Studies Therm Eng. 2022;40:102524. doi:10.1016/j.csite.2022.102524
- Jalili B, Ganji AD, Jalili P, et al. Thermal analysis of Williamson fluid flow with Lorentz force on the stretching plate. Case Studies Therm Eng. 2022;39:102374. doi:10.1016/j.csite.2022.102374
- Du J, Yang MN, Yang SF. Correlations and optimization of a heat exchanger with offset fins by genetic algorithm combining orthogonal design. Appl Therm Eng. 2016;107:1091–1103. doi:10.1016/j.applthermaleng.2016.04.074
- Liu F, Shao M, Zhang R, et al. The influence of tip clearance and shape of pin fins on the heat transfer performance and friction factor in aluminum silicon alloy heat exchanger. Numer Heat TR A: Appl. 2020;77(1):51–68. doi:10.1080/10407782.2019.1678320
- Xue Y, Ge Z, Du X, et al. On the heat transfer enhancement of plate fin heat exchanger. Energies. 2018;11(6):1398. doi:10.3390/en11061398
- Unger S, Beyer M, Gruber S, et al. Experimental study on the air-side thermal-flow performance of additively manufactured heat exchangers with novel fin designs. Int J Therm Sci. 2019;146:106074. doi:10.1016/j.ijthermalsci.2019.106074
- Ibrahim TK, Al-Sammarraie AT, Al-Jethelah MS, et al. The impact of square shape perforations on the enhanced heat transfer from fins: experimental and numerical study. Int J Therm Sci. 2020;149:106144. doi:10.1016/j.ijthermalsci.2019.106144
- Ajeel RK, Salim WI, Hasnan K. Thermal and hydraulic characteristics of turbulent nanofluids flow in trapezoidal-corrugated channel: symmetry and zigzag shaped. Case Studies Ther Eng. 2018;12:620–635. doi:10.1016/j.csite.2018.08.002
- Ajeel RK, Salim WI, Hasnan K. Influences of geometrical parameters on the heat transfer characteristics through symmetry trapezoidal-corrugated channel using SiO2-water nanofluid. Int Commun Heat Mass Transfer. 2019;101:1–9. doi:10.1016/j.icheatmasstransfer.2018.12.016
- Ajeel RK, Salim WI, Hasnan K. Thermal performance comparison of various corrugated channels using nanofluid: numerical study. Alexandria Eng J. 2019;58(1):75–87. doi:10.1016/j.aej.2018.12.009
- Ajeel RK, Salim WI, Sopian K, et al. Turbulent convective heat transfer of silica oxide nanofluid through corrugated channels: An experimental and numerical study. Int J Heat Mass Transfer. 2019;145:118806. doi:10.1016/j.ijheatmasstransfer.2019.118806