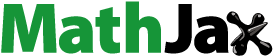
Abstract
The classical viscous theory is limited to illustrating the characteristics of several materials like pseudoplastic and dilatant fluids. Sutterby fluid has the features of shear thinning and shear thickening fluids because of its Power law index. Therefore, this study considered an incompressible, time-independent and electrically conducting Sutterby fluid flow across a rotating and stretchable disk. The disk experiences the effect of porous space. The energy equation has variable conductivity, heat source and thermal relaxation time features while mass equation exploits the influence of chemical reaction. The aspects of Buongiorno nanofluid theory are also examined in the Sutterby flow model. The phenomenon of Stefan blowing is analysed through mass transfer rate at the surface of disk. The flow expressions are first transferred into a new system of single independent variable and then treated numerically via Runge–Kutta–Fehlberg (RKF) method combined through shooting process. The behaviour of distinguished physical quantities is discussed graphically on momentum, mass species and thermal fields. The numeric data of drag force, Sherwood number and Nusselt number is calculated against several physical parameters.
Nomenclature
= | cylindrical coordinate system | |
= | angular velocity | |
= | constant stretching rate | |
= | wall temperature | |
= | wall concentration | |
= | ambient fluid concentration | |
= | magnetic field | |
= | electrical conductivity | |
= | porosity of porous space | |
= | variable thermal conductivity | |
= | ratio of heat capacities | |
= | thermophoretic diffusion coefficient | |
= | thermal relaxation time | |
= | viscosity at weaker shear rate | |
= | dimensionless number | |
= | first Rivlin–Ericksen tensor | |
= | transpose gradient | |
= | dimensionless velocities | |
= | dimensionless nanoparticles concentration | |
= | material parameter | |
= | Prandtl number | |
= | heat source parameter | |
= | stretching parameter | |
= | thermal relaxation parameter | |
= | chemical reaction parameter | |
= | shear stresses | |
= | heat flux | |
= | Sherwood number | |
= | velocity components | |
= | temperature | |
= | density | |
= | concentration | |
= | ambient fluid temperature | |
= | fluid concentration | |
= | uniform magnetic field strength | |
= | viscosity | |
= | permeability of porous space | |
= | specific heat | |
= | Brownian diffusion coefficient | |
= | heat source rate | |
= | chemical reaction rate | |
= | variable thermal conductivity parameter | |
= | characteristic time | |
= | velocity gradient | |
= | similarity variable | |
= | dimensionless temperature | |
= | Reynolds number | |
= | magnetic parameter | |
= | Schmidt number | |
| = | Brownian motion parameter |
= | thermophoretic parameter | |
= | porosity parameter | |
= | Stefan blowing parameter | |
= | mass flux | |
= | skin-friction coefficients | |
= | Nusselt number |
1. Introduction
The analysis of fluid flow phenomenon is inspected in various physical situations [Citation1–7]. Such physical problems have various applications such as determination of fuel rate through pipelines, scheming of oceanographic streams and moments of aircrafts estimation. More precisely, the phenomenon of stretchable/rotating flows has significance in spin coating, swirl generators and surface curvature in bends [Citation8–11]. The analysis of rotating flows also features the thermal stability of condensers, electro chemical reactions, heat exchangers and jet engines. Mahanthesh et al. [Citation12] visualized the impacts of non-linear thermal radiation in hydromagnetic fluid flow with mixture of nanoparticles under the rotating disk configuration. The numerical solution of thin transient film flow of non-Newtonian fluid about a revolving disk is addressed by Ahmed et al. [Citation13]. The influence of magnetic field was found worthwhile in reducing the film thickness. Turkyilmazoglu [Citation14] presented the interactional phenomenon of suspended particles and fluid about a spinning stretchable disk and analysed the resulting heat transfer rate and flow behaviour. The flow features of Oldroyd-B fluid flow near stagnation point subjected to the stretchable disk rotation were observed by Hafeez et al. [Citation15]. Numerical investigations predicted that the velocity is enhanced by the larger stretched parameter. Abbasi et al. [Citation16] discussed the bioconvective flow of viscoelastic fluid induced by a revolving stretching disk. Shehzad et al. [Citation17] investigated the phenomenon of Casson–Maxwell fluid flow across a rotating disk in numerical manner. They demonstrated that Deborah number and Casson parameter are responsible in the reduction phenomenon of the radial velocity field while the tangential velocity is increased by the rotation parameter.
Various materials which are involved in engineering processes revealed non-Newtonian fluids behaviour. The classical theory of Newtonian fluid is limited to certain materials and is lacking in illustration especially the features of dilatant and pseudoplastic fluids. To describe the deformation of such fluids, various theories for non-Newtonian fluids have been proposed. Among which Sutterby fluid model has significant features of Power law index n due to which it preserves the characteristics of shear thinning, Newtonian and shear thickening fluids. Hayat et al. [Citation18] analysed the mass and thermal transport phenomenon in Sutterby nanofluid flow about a rotating disk. It was examined that velocity field is declined by the material parameter. The aspects of irreversibility in Sutterby fluid flow through rotating parallel disks were demonstrated by Hayat et al. [Citation19]. Khan et al. [Citation20] studied the effects of chemically reactive flow of Sutterby fluid through rotating disk. Hayat et al. [Citation21] scrutinized the features of nonlinear thermal flow in Sutterby fluid near rotating stretchable disk. They found that velocity field is enhanced by the disk thickness. Rehman et al. [Citation22] examined the phenomenon of thermal stratification in nanoliquid Sutterby flow deformed through stretchable surface. They determined that velocity field is declined through increase in fluid parameter. Sohail and Naz [Citation23] developed a mathematical model of Sutterby nanofluid across a stretchable cylinder characterized by the porous space. Usman et al. [Citation24] characterized the significant features of Sutterby nanofluid flow above a stretchable wedge saturated by a porous region. Aldabesh et al. [Citation25] featured the flow and non-linear thermal aspects in Sutterby nanofluid flow by a stretched cylinder. They inspected that Sutterby fluid parameter has strong impact in the deterioration of velocity field. Abbas et al. [Citation26] demonstrated the flow phenomenon of Sutterby fluid through a stretchable cylinder with thermophysical properties, thermal slip and Darcy resistance.
The energy transformation through the production of cooling/heating in industrial processes has attained vital significance. Several techniques have been implemented to enhance the productivity of thermal systems, but all methods contain instability features of low thermal conductivity. Choi [Citation27] established stable and prolonged solution by dispersing nanometer sized refined particles into conventional basefluid and termed nanofluid. Because of less in dimension, the nanoparticles minimize issues like homogeneity, particle blockage and sedimentation. Moreover, nanofluids are used in various industrial applications such as power lasers, metallurgical sector, power generation, twisted helical tapes, photovoltaic systems and solar collectors [Citation28,Citation29]. Rehman et al. [Citation30] highlighted the slips effects in flow of Casson nanofluid yield through a rotating disk. The heat production/absorption and velocity slip features of nanofluid flow over a spinning disk were presented by Aziz et al. [Citation31]. The numerical study through ND-solve procedure predicted that the Brownian motion parameter has opposite impact on concentration and temperature fields. Ijaz et al. [Citation32] analysed the mechanism of activation energy in nonlinear thermal nanofluid flow generated by a rotating disk. Ahmed et al. [Citation33] investigated the effects of heat flux in thin film movement of Maxwell nanofluid caused by the stretchable spiraling disk. A reducing nature of surface heat flux is found through thermophoresis parameter. The heat augmentation analysis in Reiner–Rivlin nanofluid about a rough revolving disk was examined by Naqvi et al. [Citation34]. Zaib et al. [Citation35] exploited the potential of nanomaterials in mixed convective micropolar fluid flow from a wedge. Khan et al. [Citation36] discussed the significance of radiative nanoliquid particles in Sisko fluid flow about a curved surface. Upreti et al. [Citation37] studied the entropy generation in convective flow of hybrid nanofluids about a stretchable porous surface. Pandey et al. [Citation38] examined the magnetized flow characteristics of chemically reactive hybrid nanofluid with multiple slip mechanism. Joshi et al. [Citation39] discussed the impacts of suction/blowing in Darcy–Forchheimer flow of hybrid nanofluid passed a stretching surface. Upreti et al. [Citation40] examined the thermal behaviour of nanofluid across a rotating disk with temperature-dependent fluid properties. Upreti et al. [Citation41] analysed the thermal features of nanoparticles in magnetized Casson fluid flow subjected to a Riga plate.
In numerous industrial processes, heat is produced by the internal resistance. The temperature difference in such industrial procedures is changed remarkably by physical phenomena such as thermal conductivity mainly in the lubrication theory which is used in the design process of fluid bearing. Many scientists adopted the temperature-dependent conductivity relationship for the refinement of the product such as in wear and viscometery. Rauf et al. [Citation42] scrutinized the nature of viscous fluid flow induced by the oscillation of rotatory disk. Dual solutions were obtained for viscous fluid flow near a stretchable/shrinkable revolving disk under temperature-dependent conductivity by Naganthran et al. [Citation43]. Rafiq et al. [Citation44] formulated the flow problem of viscous fluid across a spinning disk by considering the effects of temperature-dependent fluid properties. They investigated in numerical way that variable thermal conductivity altered overall thermal rate. Shehzad et al. [Citation45] elaborated the thermophysical features (density, thermal conductivity, and viscosity) of compressible viscous fluid flow through oscillatory spinning disk. Khan et al. [Citation46] explored the thermophysical revolution of Newtonian fluid flow across a heated revolving disk. It was calculated that the temperature distribution is enhanced through variable thermal conductivity. Khan et al. [Citation47] studied the gas and liquid phenomenon close to the region of boundary layer subjected to a disk rotation.
Considering the characteristics of shear thinning and thickening fluids, the Flow model of Sutterby is considered in the presence of externally applied magnetic field. The fluid flow is subjected to the stretchable rotating disk which is characterized by the porous space. The energy equation is incorporated by the Buongiorno nanofluid Cattaneo–Christov theories with the features of variable thermal conductivity. Such expressions are important in the enhancement and to stable the heat transfer process. The phenomenon of mass transfer at the disk surface is considered through Stefan blowing [Citation48–50]. In Stefan effect, an extensive mass diffusion across impermeable surface to ambient occurs such as evaporation in the process of paper dying. The numerical study of Sutterby nanofluid flow across rotating disk with Stefan blowing phenomenon makes the study very interactive and an appealing one to the scientist’s community.
2. Problem statement
An electrically conducting Sutterby nanofluid flow is scrutinized over a stretchable and rotating disk. The disk is placed in the plane , stretchable along radial axis with constant stretching rate
and rotates with constant angular speed
(see Figure ). The fluid is occupied in the region
and flow is characterized by the impacts of porous space, heat generation, thermal relaxation time, chemical reaction and the Stefan blowing. The disk surface maintains wall temperature
and wall concentration
, while ambient fluid temperature is
and concentration
. Magnetic field having uniform strength
is implemented through axial direction. With low Reynolds number assumption, the induced magnetic field is ignored. The electric field is also neglected. The axi-symmetric flow is assumed and therefore the calculations are omitted along the tangential axis
. Following the above assumptions, the governing system is given as [Citation18,Citation20,Citation48]:
(1)
(1)
(2)
(2)
(3)
(3)
(4)
(4)
(5)
(5)
with the prescribed boundary conditions [Citation18,Citation48]:
(6)
(6)
Here, the velocity components are elaborated by
, density
, electric conductivity
dynamic viscosity
, temperature
, applied magnetic force strength
, ratio of heat capacities
, specific heat
, the coefficient of Brownian diffusion
, coefficient of thermophoresis diffusion
, heat source rate
, ambient temperature
, concentration
, reaction rate
, thermal time relaxation time
, temperature dependent thermal conductivity
, porosity of the porous space
and permeability of the porous space
.
In numerous industrial processes such as glass blowing and paper manufacturing, mass transportation appeared between stretching surfaces and liquid flows. Therefore, we considered an interesting phenomenon of Stefan blowing due to which mass diffusion can occur from stretching surface to ambient. The Stefan blowing theory is different form porous surface in case of mass injection/blowing owing to the transpiration.
The variable property is defined as [Citation50]:
where thermal conductivity parameter is determined by
.
The viscosity relation formula for Sutterby fluid model is expressed as
(7)
(7)
where the viscosity at weaker shear rates is
, dimensionless quantity
and characteristic time
are positive parameters and shear rate is represented by
. The Sutterby model demonstrates the viscous fluid at
and converted to Eyring model when
. Through Binomial expansion, one can write:
(8)
(8)
The shear rate can be reported as
(9)
(9)
where the first-order Rivlin–Erickson tensor is reported by
and can be expressed as
Here,
and
stand for velocity gradient and transpose respectively.
Considering the following similarity variables [Citation18]:
(10)
(10)
Continuity formula is verified automatically. The other expressions have the forms:
(11)
(11)
(12)
(12)
(13)
(13)
(14)
(14)
The specified boundary conditions are [Citation18,Citation48]:
(15)
(15)
where
,
,
,
,
.
Here, we denoted the material parameter , Reynolds number
, magnetized parameter
, Prandtl number
, Schmidt number
, heat source parameter
, Brownian motion parameter
, stretching parameter
, thermophoresis parameter
,thermal relaxation time parameter
, Porosity parameter
, chemical reaction parameter
and Stefan bowling parameter
.
The drag forces , Nusselt number
and Sherwood number
are defined as [Citation18,Citation20]
(16)
(16)
where mass flux
, heat flux
, shear stresses
and
are given by [Citation18,Citation20]
(17)
(17)
After substituting Equation (17) in Equation (16), we get
(18)
(18)
Here,
indicates the local Reynolds number.
3. Numerical procedure
The reduced system (11)–(15) represents the nonlinear behaviour. To express these nonlinear problem solutions, RKF method via shooting process is executed. The solution procedure begins with initial guess. The mess selection in such process assures the accurate continuous solution. However, in the case of singular Jacobian the solver may diverge for the system.
For the numerical procedure we set:
(19)
(19)
Now by using Equation (19) into system of Equations (11)–(14), we get
(20)
(20)
(21)
(21)
(22)
(22)
(23)
(23)
In view of (19), Equation (15) is converted as
(24)
(24)
The missing conditions in (24) are guessed through Newton–Raphson algorithm with shooting method. At last, the problem is integrated at
to attain the condition. The criterion for the convergence is adjusted to at least 10−6.
4. Results and discussion
This section elaborates the results generated against the dissimilar values of physical constraints on quantities of practical implications. The graphical results are drawn on velocity, temperature and concentration fields while the tabular benchmarks are computed for drag force, Nusselt number and Sherwood number. For the numerical computations, we fixed and
.
Figure (a and b) discussed the stretching parameter influence on radial and tangential velocity fields, respectively. Figure (a) declares that the radial velocity profiles are enhanced by the stretching parameter. Since the stretching constraint is directly related to the stretching rate of disk, therefore, an enhancement in the stretching constraint leads to an increment in the disk stretching rate. According to which, the radial profiles are depicting modifying behaviour. The tangential velocity field is declined by the stretching ratio constraint (see Figure b). As stretching parameter is inversely proportional to the disk angular speed therefore increase in values of , causes a reduction in angular speed. According to which a reducing nature of tangential velocity profile is visualized. Figure (a and b) is drawn to discuss the domination of magnetic factor on
and
respectively. In Figures (a) and (b), both the velocity fields (
&
) representing the reducing nature against the enlarged magnetic factor values. The strengthened magnetic field is appeared against the higher magnetic factor that produces a resistance in the flow and consequently the velocity field is declined as reported by Figure (a and b). The outcomes of porosity parameter onto the velocity fields
and
are illustrated in Figure (a and b). The profiles of radial and tangential velocities are reporting a reducing scenario for the modified values of
. The fluid flow becomes more viscous due to enhancement in the porosity parameter. As a result, the resistance between the layers of fluid flow increases, therefore, the velocity field is decreased (see Figure a and b).
The investigations of material parameter on velocity curves are demonstrated through Figure (a and b). The involvement of the characteristic time in the material parameter has strong impact on velocity field, therefore the velocity profiles are reduced through increased material parameter. Similar to the behaviours of magnetic and porosity factors, the velocity fields ( &
) also prescribing the reducing nature against an increased Reynolds number (see Figure a and b). The outcome of variable conductivity constraint on temperature field is mentioned by Figure (a). The thermal curves along its boundary layer thickness are strengthen due to the magnification in
, which means that more heat is added the thermal phenomenon by the temperature-dependent thermal conductivity. Figure (b) exemplifies the influence of heat source parameter of thermal field. With increase in
, the more heat is added to the fluid phenomenon therefore an upsurge in thermal curves with its boundary layer is noticed. Figure (c) is organized to show the action of thermal relaxation time parameter on temperature field. Modification in
causes a depletion in the temperature curves. Due to increase in relaxation time, the liquid particles are taking higher time for the energy transmission to the closest particles. According to this physical factor, the temperature profiles preserve the decaying phenomenon. Figure (a and b) are sketched to demonstrate the phenomenon of thermophoresis and Brownian motion parameters of temperature field. Figure (a and b) describe that the amplified
and
values affect the thermal rate strongly. An upsurge in the nanofluid temperature is identified for enhancing values of
and
. Physically, thermophoresis parameter expresses the temperature difference of nanofluid between fluid temperature at infinity and hot fluid at the surface. Therefore, the temperature difference at the surface and at infinity increases due to enhancement in
and hence temperature profiles are enhanced. Figure (a) predicted the curves of Schmidt number on concentration field. The profiles are observed to be a reducing function of Schmidt number. Objectively, the Schmidt number has inversely related with the Brownian diffusion. The diffusion process is decreased due to increase in
values and so as and outcome the concentration field is reduced. The influence of Stefan blowing parameter onto the concentration field is demonstrated through Figure (b). The concentration curves are attained increasing trend against enhanced values of Stefan blowing parameter. Due to rise in
values, the more mass is transferred into fluid through disk surface and that is why the concentration field is enhanced. Furthermore, the case
shows that there is no mass blowing through the surface of the disk. Figure (c) reported that the concentration profiles are fall off by the chemical reaction parameter.
Figure (a and b) are sketched to describe the effect of porosity parameter on drag forces. The drag forces are enhanced by the porosity parameter as can be seen in Figures (a) and (b), Figure is portrayed to illustrate the influence of thermal relaxation time parameter on Nusselt number. The Nusselt number is enhanced owing to increase in the values of . The Sherwood number is an enhancing function of chemical reaction parameter as shown in Figure .
Drag force is calculated along radial and tangential directions against various parameters in Table . Drag force
is enhanced at the disk surface against increased Reynolds number and magnetic parameter values, however, a decreasing trend in
and
is noted for modified values of the material parameter. Table depicts the effects of several physical parameters such as Reynolds number, conductivity and heat source on Nusselt number. Nusselt number is demonstrating increasing nature for enlarged
, while decreasing trend is observed against enhanced
and
values. The outcomes of
on Sherwood number is illustrated by Table . Sherwood number is modified through Schmidt number, whereas it is decreased against the rising values of Stefan blowing parameter. Table is plotted for the validity of numerical scheme under limiting scenario. Heat transfer rate is calculated against the values of thermophoretic parameter, Brownian motion parameter and the Prandtl number while keeping
. An excellent agreement is numerical values of heat transfer rate noted in Table .
Table 1. Numeric data of and
against dissimilar values of
and
.
Table 2. Numerical data of against dissimilar values of
and
.
Table 3. Numeric data of against dissimilar values of
and
.
Table 4. Comparison of numerical results with [Citation18] in terms of heat transfer rate against
and
values by fixing
.
5. Conclusion
An incompressible magnetized flow of Sutterby about a rotating and stretchable disk is examined. Cattaneo–Christov expression along with the features of temperature dependent thermal conductivity and heat source are adopted in the energy equation. A well-known Buongiorno nanofluid theory is established in the flow model of Sutterby fluid. Mass transportation across the disk surface is also examined through Stefan blowing effect. Flow governing system is first reduced to a non-linear system of coupled ordinary differential equations and then solved by RKF numerical procedure. Following main points are explored:
Radial and tangential velocities are reduced for increased material parameter values. Similar effects are observed in the velocity profiles of magnetic parameter and porosity parameter.
The temperature is enhanced by the variable conductivity constraint and is decreased through thermal relaxation time factor.
Concentration profiles are modified due to enhanced Stefan blowing parameter.
Drag force along the radial direction is increased by the Reynolds number, while it is declined by the material parameter.
A reduction in Nusselt number is noted against the heat source parameter and an enhancement in Sherwood number is observed for modified chemical reaction parameter.
6. Future directions
This study can be extended to the following in future:
Influence of variable thermal conductivity in magnetized flow of Sutterby nanofluid about a rotating stretchable disk with Arrhenius activation energy.
The impact of horizontal magnetic field in flow of Sutterby nanofluid above a rotating disk.
Disclosure statement
No potential conflict of interest was reported by the author(s).
References
- Zaib A, Khan U, Khan I, et al. Entropy generation and dual solutions in mixed convection stagnation point flow of micropolar Ti6Al4V nanoparticle along a Riga surface. Processes. 2020;8:14. doi:10.3390/pr8010014
- Khan U, Zaib A, Shah Z, et al. Impact of magnetic field on boundary-layer flow of Sisko liquid comprising nanomaterials migration through radially shrinking/stretching surface with zero mass flux. J Mater Res Technol. 2020;9:3699–3709. doi:10.1016/j.jmrt.2020.01.107
- Singh K, Pandey AK, Kumar M. Numerical solution of micropolar fluid flow via stretchable surface with chemical reaction and melting heat transfer using Keller–Box method. Propul Power Res. 2021;10:194–207. doi:10.1016/j.jppr.2020.11.006
- Fang F, Kumar RN, Prasannakumara BC, et al. Aspects of uniform horizontal magnetic field and nanoparticle aggregation in the flow of nanofluid with melting heat transfer. Nanomaterials. 2022;12:1000. doi:10.3390/nano12061000
- Ali B, Shafiq A, Siddique I, et al. Significance of suction/injection gravity modulation, thermal radiation, and magnetohydrodynamic on dynamics of micropolar fluid subject to an inclined sheet via finite element approach. Case Stud Therm Eng. 2021;28:101537. doi:10.1016/j.csite.2021.101537
- Ali B, Thumma T, Habib D, et al. Finite element analysis on transient MHD 3D rotating flow of Maxwell and tangent hyperbolic nanofluid past a bidirectional stretching sheet with Cattaneo Christov heat flux model. Therm Sci Eng Prog. 2022;28:101089. doi:10.1016/j.tsep.2021.101089
- Ali B, Siddique I, Ahmadian A, et al. Significance of Lorentz and Coriolis forces on dynamics of water based silver tiny particles via finite element simulation. Ain Shams Eng J. 2022;13:101572. doi:10.1016/j.asej.2021.08.014
- Shevchuk IV. Convective heat and mass transfer in rotating disk systems. Heidelberg: Springer Verlag, Berlin; 2009.
- Shevchuk IV. Modelling of convective heat and mass transfer in rotating flows. Springer Berlin, Heidelberg: Springer International Publishing Switzerland; 2016.
- Bhatti MM, Shahid A, Sarris IE, et al. Spectral relaxation computation of Maxwell fluid flow from a stretching surface with quadratic convection and non-Fourier heat flux using Lie symmetry transformations. Int J Mod Phys B. 2023;37:2350082. doi:10.1142/S0217979223500820
- Zhang L, Tariq N, Bhatti MM. Study of nonlinear quadratic convection on magnetized viscous fluid flow over a non-Darcian circular elastic surface via spectral approach. J Taibah Univ Sci. 2023;17:2183702. doi:10.1080/16583655.2023.2183702
- Mahanthesh B, Gireesha BJ, Shehzad SA, et al. Nonlinear radiated MHD flow of nanoliquids due to a rotating disk with irregular heat source and heat flux condition. Phys B. 2018;537:98–104. doi:10.1016/j.physb.2018.02.009
- Ahmed J, Khan M, Ahmad L. Transient thin film flow of nonlinear radiative Maxwell nanofluid over a rotating disk. Phys Lett A. 2019;383:1300–1305. doi:10.1016/j.physleta.2019.01.024
- Turkyilmazoglu M. Suspension of dust particles over a stretchable rotating disk and two-phase heat transfer. Int J Multiphase Flow. 2020;127:103260. doi:10.1016/j.ijmultiphaseflow.2020.103260
- Hafeez A, Khan M, Ahmed J. Flow of Oldroyd-B fluid over a rotating disk with Cattaneo–Christov theory for heat and mass fluxes. Comput Methods Programs Biomed. 2020;191:105374. doi:10.1016/j.cmpb.2020.105374
- Abbasi A, Mabood F, Farooq W, et al. Bioconvective flow of viscoelastic nanofluid over a convective rotating stretching disk. Int Commun Heat Mass Transfer. 2020;119:104921. doi:10.1016/j.icheatmasstransfer.2020.104921
- Shehzad SA, Mabood F, Rauf A, et al. Rheological features of non-Newtonian nanofluids flows induced by stretchable rotating disk. Phys Scr. 2021;96:035210. doi:10.1088/1402-4896/abd652
- Hayat T, Ahmad S, Khan MI, et al. Modeling chemically reactive flow of Sutterby nanofluid by a rotating disk in presence of heat generation/absorption. Commun Theor Phys. 2018;69:569–576. doi:10.1088/0253-6102/69/5/569
- Hayat T, Afzal S, Khan MI, et al. Irreversibility aspects to flow Sutterby fluid subject to nonlinear heat flux and Joule heating. Appl Nanosci. 2019;9:1215–1226. doi:10.1007/s13204-019-01015-3
- Khan MI, Qayyum S, Hayat T, et al. Stratified flow of Sutterby fluid with homogeneous–heterogeneous reactions and Cattaneo–Christov heat flux. Int J Numer Methods Heat Fluid Flow. 2019;29:2977–2992. doi:10.1108/HFF-12-2018-0762
- Hayat T, Masood F, Qayyum S, et al. Sutterby fluid flow subject to homogeneous-heterogeneous reactions and nonlinear radiation. Physica A. 2020;544:123439. doi:10.1016/j.physa.2019.123439
- Rehman S, Mir NA, Farooq M, et al. Analysis of thermally stratified flow of Sutterby nanofluid with zero mass flux condition. J Mater Res Technol. 2020;9:1631–1639. doi:10.1016/j.jmrt.2019.11.088
- Sohail M, Naz R. Modified heat and mass transmission models in the magnetohydrodynamic flow of Sutterby nanofluid in stretching cylinder. Physica A. 2020;549:124088. doi:10.1016/j.physa.2019.124088
- Usman PL, Ghaffari A. Heat and mass transfer in a steady flow of Sutterby nanofluid over the surface of a stretching wedge. Phys Scr. 2021;96:065003. doi:10.1088/1402-4896/abecf7
- Aldabesh A, Haredy A, Al-Khaled K, et al. Darcy resistance flow of Sutterby nanofluid with microorganisms with applications of nano-biofuel cells. Sci Rep. 2022;12. doi:10.1038/s41598-022-11528-7
- Abbas N, Shatanawi W, Shatnawi TAM, et al. Theoretical analysis of induced MHD Sutterby fluid flow with variable thermal conductivity and thermal slip over a stretching cylinder. AIMS Mathematics. 2023;8:10146–10159. doi:10.3934/math.2023513
- Choi SUS. Enhancing thermal conductivity of fluids with nanoparticles, developments and applications of non-Newtonian flows. Am Soc Mech Eng. 1995;66:99–105.
- Li Z, Sheikholeslami M, Jafaryar M, et al. Investigation of nanofluid entropy generation in a heat exchanger with helical twisted tapes. J Mol Liq. 2018;266:797–805. doi:10.1016/j.molliq.2018.07.009
- Sheikholeslami M, Farshad SA, Ebrahimpour Z, et al. Recent progress on flat plate solar collectors and photovoltaic systems in the presence of nanofluid: a review. J Cleaner Prod. 2021;293:126119. doi:10.1016/j.jclepro.2021.126119
- Rehman KU, Malik MY, Zahri M, et al. Numerical analysis of MHD Casson Navier’s slip nanofluid flow yield by rigid rotating disk. Results Phys. 2018;8:744–751. doi:10.1016/j.rinp.2018.01.017
- Aziz A, Alsaedi A, Muhammad T, et al. Numerical study for heat generation/absorption in flow of nanofluid by a rotating disk. Results Phys. 2018;8:785–792. doi:10.1016/j.rinp.2018.01.009
- Ijaz M, Ayub M, Khan H. Entropy generation and activation energy mechanism in nonlinear radiative flow of sisko nanofluid: rotating disk. Heliyon. 2019;6:e01863. doi:10.1016/j.heliyon.2019.e01863
- Ahmed J, Khan N, Ahmed L. Radiative heat flux effect in flow of Maxwell nanofluid over a spiraling disk with chemically reaction. Physica A. 2020;551:123948. doi:10.1016/j.physa.2019.123948
- Naqvi SMRS, Kim HM, Muhammad T, et al. Numerical study for slip flow of Reiner–Rivlin nanofluid due to a rotating disk. Int Commun Heat Mass Transfer. 2020;116:104643. doi:10.1016/j.icheatmasstransfer.2020.104643
- Zaib A, Haq RU, Sheikholeslami M, et al. Numerical analysis of effective Prandtl model on mixed convection flow of γAl2O3–H2O nanoliquids with micropolar liquid driven through wedge. Phys Scr. 2020;95:035005. doi:10.1088/1402-4896/ab5558
- Khan U, Zaib A, Ishak A. Magnetic field effect on Sisko fluid flow containing gold nanoparticles through a porous curved surface in the presence of radiation and partial slip. Mathematics. 2021;9:921. doi:10.3390/math9090921
- Upreti H, Pandey AK, Kumar M. Assessment of entropy generation and heat transfer in three-dimensional hybrid nanofluids flow due to convective surface and base fluids. J Porous Media. 2021;24:35–50. doi:10.1615/JPorMedia.2021036038
- Pandey AK, Upreti H, joshi N, et al. Effect of natural convection on 3D MHD flow of MoS2GO/H2O via porous surface due to multiple slip mechanisms. J Taibah Univ Sci. 2022;16:749–762. doi:10.1080/16583655.2022.2113729
- Joshi N, Upreti H, Pandey AK. MHD Darcy–Forchheimer Cu–Ag/H2O–C2C6O2 hybrid nanofluid flow via a porous stretching sheet with suction/blowing and viscous dissipation. Int J Comput Methods Eng Sci Mech. 2022;23:527–535. doi:10.1080/15502287.2022.2030426
- Upreti H, Uddin Z, Pandey AK, et al. Particle swarm optimization based numerical study for pressure, flow, and heat transfer over a rotating disk with temperature dependent nanofluid properties. Numer Heat Transf. Part A: Appl. 2023;83:815–844. doi:10.1080/10407782.2022.2156412
- Upreti H, Pandey AK, Joshi N, et al. Thermodynamics and heat transfer analysis of magnetized Casson hybrid nanofluid flow via a Riga plate with thermal radiation. J Comput Biophys Chem. 2023;22:321–334. doi:10.1142/S2737416523400070
- Rauf A, Abbas Z, Shehzad SA, et al. Characterization of temperature-dependent fluid properties in compressible viscous fluid flow induced by oscillation of disk. Choas Solitons Fract. 2020;132:109573. doi:10.1016/j.chaos.2019.109573
- Naganthran K, Mustafa M, Mushtaq A, et al. Dual solutions for fluid flow over a stretching/shrinking rotating disk subject to variable fluid properties. Physica A. 2020;556:124773. doi:10.1016/j.physa.2020.124773
- Rafiq T, Mustafa M, Farooq MA. Modeling heat transfer in fluid flow near a decelerating rotating disk with variable fluid properties. Int Commun Heat Mass Transfer. 2020;116:104673. doi:10.1016/j.icheatmasstransfer.2020.104673
- Shehzad SA, Abbas Z, Rauf A, et al. Effectiveness of Hall current and thermophysical properties in compressible flow of viscous fluid through spinning oscillatory disk. Int Commun Heat Mass Transfer. 2020;116:104678. doi:10.1016/j.icheatmasstransfer.2020.104678
- Khan M, Salahuddin T, Stephen SO. Thermo-physical characteristics of liquids and gases near a rotating disk. Choas Solitons Fract. 2020;141:110304. doi:10.1016/j.chaos.2020.110304
- Khan M, Salahuddin T, Stephen SO. Variable thermal conductivity and diffusivity of liquids and gases near a rotating disk with temperature dependent viscosity. J Mol Liq. 2021;333:115749. doi:10.1016/j.molliq.2021.115749
- Latiff NA, Uddin MJ, Ismail AIM. Stefan blowing effect on bioconvective flow of nanofluid over a solid rotating stretchable disk. Propulsion Power Res. 2016;5:267–278. doi:10.1016/j.jppr.2016.11.002
- Zohra FT, Uddin MJ, Ismail AIM, et al. Anisotropic slip magneto-bioconvection flow from a rotating cone to a nanofluid with Stefan blowing effects. Chin J Phys. 2018;56:432–448. doi:10.1016/j.cjph.2017.08.031
- Mabood F, Rauf A, Prasannakumara BC, et al. Impacts of Stefan blowing and mass convection on flow of Maxwell nanofluid of variable thermal conductivity about a rotating disk. Chin J Phys. 2021;71:260–272. doi:10.1016/j.cjph.2021.03.003