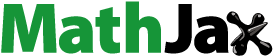
Abstract
The research explores the use of iron oxide nanoparticles to enhance the thermal properties of fluids like water and ethylene glycol. These nanoparticles find applications in engineering and medicine, including wastewater treatment and coatings. The study investigates magnetic nanoparticle movement in a permeable enclosure, considering heat, magnetic field, and electromagnetic effects. Using the MAC method, dimensionless equations were solved, and results were presented through graphs, charts, and tables. Findings show nanoparticle volume significantly impacts heat transfer in nanofluids compared to base fluids. Increasing nanoparticle volume from 0 to 0.05 improved nanofluid heat transfer by 0.278% and base fluid by 0.303%. Heat absorption and generation improved nanofluid transfer by 15.819% and base fluid by 0.596%. Nusselt number increased with higher Eckert number and heat source/sink parameters in both nanofluids and base fluids.
Nomenclature
= | Dimensional Cartesian coordinates [m] | |
= | Dimensionless Cartesian coordinate system [–] | |
= | Dimensional velocity components in x, y directions [ms−1] | |
= | Length of the enclosure [m] | |
= | Acceleration due to gravity [ms−2] | |
= | Dimensional pressure [Pa] | |
= | Dimensionless velocities along X and Y directions [–] | |
= | Permeability of the porous medium [m2] | |
= | Magnetic field strength [kg s−2A−1] | |
= | Height of the enclosure [m] | |
= | Constant reference velocity [ms−1] | |
= | Temperature of the hot wall [K] | |
= | Dimensional heat generation or absorption [W m−3] | |
= | Temperature of the cold wall [K] | |
= | Dimensionless pressure [–] | |
= | Dimensional temperature of the fluid [K] | |
= | Reynolds number [–] | |
= | Richardson number [–] | |
= | Grashof number [–] | |
= | Dimensional time [s] | |
= | Prandtl number [–] | |
= | Dimensionless heat generation/absorption coefficient [–] | |
= | Eckert number [–] | |
= | Nusselt number [–] | |
= | Darcy number [–] | |
= | Hartmann number [–] |
Greek symbols
= | Density [kg m−3] | |
= | Kinematic viscosity [m2 s−1] | |
= | Dynamic viscosity [kg m−1s−1] | |
= | Thermal diffusivity [m2 s−1] | |
= | Thermal expansion coefficient [K−1] | |
= | Dimensionless temperature [−] | |
= | Electrical conductivity [S m−1] |
Subscripts
f | = | Fluid |
nf | = | Nanofluid |
p | = | Nanoparticle |
Abbreviations
NP | = | Nanoparticle |
PW | = | Paraffin Wax |
1. Introduction
Nanofluids are used in numerous sectors like medical applications, electrical elements, transportation, etc. [Citation1–5]. In general, neither metal nor metal oxide solid particles (,
,
,
,
, and
) should be used in nanoliquids. There are a number of studies [Citation6–12] done theoretically or experimentally to examine the nanoliquid flow and its energy transmission characteristics.
In current times, NPs are attracting considerable attention from many researchers owing to their applications like space heating, thermal-energy storage, bone cancer treatment, removal of inorganic/organic pollutants, spin polarization, noble metals, and magnetic resonance imaging. Zhao et al. [Citation13] investigated the
nanoparticles in permeable lid walls. In their study, they found that higher Nusselt numbers were associated with porous materials. Hu et al. [Citation14] studied the nanocomposites for
-paraffin wax (PW) in a square geometry. They concluded that because the PW was doped with a greater mass fraction of
, the energy transmission efficiency within a square geometry loaded with the nanocomposites increased more rapidly. Molana et al. [Citation15] analyzed the convective
nanoliquid hydrothermal behaviour in a novel shaped cavity. They reported that an elevated Hartmann number value enhances the rate of energy transmission. Rahman et al. [Citation16] scrutinized the MHD
water conveying hybrid nanoliquid in a ⊥-shaped enclosure. They revealed that the NP volume fraction raises the average of the Bejan number. He et al. [Citation17] reported the
nanoparticles melting behaviour in a cubic enclosure. The researchers disclosed that the thermal storage capacity of the
-PW phase change nanocomposites has undergone a substantial decrease. The
and
conveying nanoparticles have been investigated by many other researchers [Citation18–21].
Porous enclosures containing a nanoliquid have gained considerable attention from many other authors. Chamkha and Al-Naser [Citation22] studied an inclined rectangular cavity filled with a porous medium. According to their findings, the permeable medium reduced fluid circulation inside the enclosure. Grosan et al. [Citation23] deliberate the energy transmission in a square geometry loaded with a permeable medium. They revealed that the elevated values of the porosity parameter enhanced energy transmission in the interior of the enclosure. Mehmood et al. [Citation24] evaluated the nanoliquid flow in square permeable enclosures. They concluded that the porosity parameter augments the kinetic energy and average heat transmission. Alsabery et al. [Citation25] analysed the
conveying of nanofluids in a wavy-walled permeable enclosure. They concluded that the isothermal lines are denser at elevated values of the Darcy number. Sumithra et al. [Citation26] analyzed the time-dependent non-Newtonian nanofluid flow over a plate, stagnation point, and wedge. They concluded that the rate of energy transmission raise with encouraging values of the Darcy number. Sumithra and Sivaraj [Citation27] studied the convective nanoliquid flow over a permeable square geometry. They found that the fluid flow is magnified by the amplification of Darcy number values. Sumithra and Sivaraj [Citation28] assessed the flow of convective nanoliquid via a rectangular porous enclosure. They concluded that the transfer of heat enhances the rise of the permeability parameter.
The applications of MHD nanofluid flow fields are used in solar collectors, MEMS, medicine, heat exchanges, and lubrication. Chamkha [Citation29] studied the natural convective MHD nanofluid flow via a vertical plate. They found that the local Nusselt number diminished with raising values of the Hartmann number. Oztop et al. [Citation30] investigated the convective MHD nanoliquid flow via a wavy-shaped enclosure. The researchers concluded that increasing values of the magnetic field parameter enhanced heat transmission. MHD convective nanoliquid flow in dissimilar geometries has been investigated by several researchers [Citation31–36].
The process by which a fluid’s viscosity absorbs heat from fluid motion and transforms it into internal fluid energy that serves as a heat source is known as ohmic heating (viscous dissipation). Viscous dissipation plays an essential role, particularly in high-velocity systems. Rahman et al. [Citation37] numerically evaluated the effects of ohmic heating in a square geometry. They concluded that the fluid flow was significantly enhanced by an elevated value of the joule heating parameter. Ghaffarpasand [Citation38] studied the water-conveying nanofluid with the effect of viscous dissipation over a lid-driven enclosure. They reveal that the entropy generation escalates with Eckert number. Kumar et al. [Citation39] studied the time-dependent ohmic dissipation from a stretching sheet. They revealed that the thermal boundary layer thickness rise for escalating Eckert number. Reddy and Panda [Citation40] studied the ohmic heating effects over a wavy-shaped permeable trapezoidal enclosure. They found that the temperature distribution was enhanced by raising the Eckert number.
In recent years, investigations on convective energy transmission under the effect of a heat source/sink have acquired several applications in the agricultural and engineering fields. Like, geophysics, fuel debris, geothermal systems, packed-bed reactors, etc. There are several studies [Citation41–47] done numerically to investigate the different geometrical configurations of the nanoliquid flow and its energy transfer attributes with the impact of the heat source/sink. The renewable energy and heat transfer performances were analyzed by several researchers [Citation48–50].
Currently, no scientific literature has mentioned this work to the best of the author’s knowledge to investigate the inclined (oblique) magnetic field effect on mixed convective nanoliquid flow within a square permeable cavity with cold and hot slits. The intention of this present analysis is to evaluate the heat transmission features of and
nanofluid flow via a square geometry with radiation, heat source or sink, porous, and a transverse magnetic field. The partially heated square geometry is used in many other engineering applications like, cooling heated electronic devices, air conditioning, thermally insulated walls, refrigerators, etc. The heat transfer features of
and
are analyzed over a square geometry. The primary objective of this study is to address the following research inquiries: 1. Which combination of nanofluids (Iron-Oxide + water nanofluid and Iron-Oxide + Ethylene Glycol nanofluid) gives the better heat transfer? 2. How does the porous medium affect nanofluid flow within the enclosure? 3. What is the impact of buoyancy on fluid flow? 4. The MAC method is employed to solve the problem. The outcomes of the present results are illustrated through graphs, bar chart, and tables.
2. Mathematical model
Considering the mixed convective unsteady 2D flow and heat transmission of a permeable square geometry (shown in Figure ) loaded with electrically conducting and
nanofluids. The Cartesian coordinates are denoted by
and
.
and
represent the length and height of the cavity. The following assumptions are considered for the study,
The square enclosure consists of four sides, with a portion of each side being heated. The middle portion of the enclosure is designated as the cold wall.
The energy equation considers the impact of heat source/sink, viscous dissipation, and ohmic heating.
The fluid flow equations along
and
directions include the effects are porous, transverse magnetic field, and buoyancy term for using the Boussinesq approximation.
The force of gravitation acts normal to the direction.
In view of the above assumptions, the governing equations are considered as follows [Citation28, Citation40, Citation44, Citation45]:
Dimensional governing equations are,
(1)
(1)
(2)
(2)
(3)
(3)
(4)
(4)
The following non-dimensional variables are acquainted:
(5)
(5) Nanofluids thermophysical properties are essential for raising the working fluid’s thermal characteristics. According to the Tiwari-Das nanoscale model, the following describes the thermophysical properties of (Figure ) nanoliquids:
Density:
Thermal expansion coefficient:
Heat capacitance:
Thermal diffusivity:
Thermal conductivity:
Nanofluid’s dynamic viscosity:
Electrical (Tables and ) conductivity:
Dimensionless forms of the governing equations by virtue of Equation Equation(5)
(6)
(6) are as follows,
(6)
(6)
(7)
(7)
(8)
(8)
(9)
(9)
Table 1. Dimensional initial and boundary conditions are.
Table 2. Thermophysical properties of NPs and base fluid [Citation15, Citation18, Citation21].
The nondimensional parameters are,
(10)
(10) The local and average Nusselt number is,
(11)
(11)
(12)
(12)
3. MAC solution and validation
The MAC technique was initially proposed by Harlow and Welch in 1965. The MAC technique is employed for solving Equations (6)-(9) using dimensionless initial and boundary conditions, as specified in Table . Reference [Citation51] contains a comprehensive explanation of the MAC method procedure.
Table 3. Dimensionless initial and boundary conditions are.
The discretized X and Y velocity equations are,
(13)
(13)
(14)
(14) Here,
The discretized energy equation is,
(15)
(15) Here,
4. Result and discussion
The behaviour of mixed convective MHD nanoliquid flow in a permeable square geometry with heat source/sink, viscous dissipation, and ohmic heating was analyzed. The outcomes of the present results are shown in tables and graphs. The range of the Reynolds number , heat source/sink
, magnetic parameter
, Joule heating and Darcy number
, Eckert number
, nanoparticle volume fraction (
), Richardson number
, and Prandtl number
, used in this study are:
Prandtl number
respectively. Throughout the study, the pertinent parameters values are frozen with
and
unless otherwise specified.
The impact of on streamline is displayed in Figure . In general, the porosity and permeability are typically related. It describes the capacity of a porous medium to permit fluid passage, and an increase in porosity improves permeability, which results in an enhancement in velocity. The characteristics of
on the streamline contour of water conveying iron oxide nanofluid are represented in Figure (A). In
, the three circulatory cells appear, and the secondary and primary circulatory cell sizes are enlarged compared with the single-layer tertiary cell. Whenever increasing the
values from 0.01–0.1, the small circulatory cell vanished, and we have only two cells that cover the whole space of the enclosure. The characteristics of
on the streamline contour of ethylene glycol conveying iron oxide nanofluid are revealed in Figure (B). At
, the double convective cells appear, and they are stretched at both horizontal walls. The secondary circulatory cell has three layers. At
, the secondary circulatory cell’s third layer merges with the primary circulatory cell, and a new tertiary cell is observed on the enclosure’s bottom right. At
, a single-layer tertiary cell eventually moves to left middle enclosure. In the overall case, Figure concludes that the porous media does not affect the influence of the fluid flow, but the streamline circulation changes significantly within the enclosure. Increases in
do not cause any changes in the magnitude of the streamlines.
The streamline contour for various is revealed in Figure . Figure (A) depicts the influence of
on streamlines contour for
nanofluid. At
, the two convective circulation zones are established, and they are located in the opposite diagonal direction. The secondary circulation zone has a smaller streamline magnitude value compared with the primary circulation zone. The primary circulation streamline magnitude is 1.001. An increase in
(
and 100), streamlines circulation inside the cavity, which is markedly changed. The interior of the streamlines core and magnitude values are abundantly shrinking. Uplifting values of
fluid flow decrease from 1.001–1.0003 and 1.0001. The fluid flow contour presents a declining tendency for elevated magnetic parameter values
. Figure (B) demonstrates the effect of
on streamlines contour for
nanofluid. The streamline magnitude value decreases, the shape of the circulation cells shrinks, and new cells are appeared for raising values of
. Uplifting values of
fluid flow decrease from 1.0003–1.0001. The Lorentz force is a combination of the magnetic and electric forces on a moving point charge due to electric fields. With an augmentation in Hartmann number, Lorentz force enhances, which opposes the velocity. It is evident from these figures that the fluid flow diminishes in magnitude when the magnetic field parameter
increases.
Figure describes the features of the . Figure (A) demonstrates the influence of
on isotherms contour for
nanofluid. Here,
represented the heat absorption,
represented the non-existence of the heat absorption/generation case, and
represented the heat generation case. Energy transfer in the enclosure depends on
. In general, both
and
cases, inside the square geometry, the yellow colour represented high thermal conductivity, and the blue colour represented low thermal conductivity. A low heat transmission was visible on the side of the cold walls, while a large heat transmission was seen on the side of the hot walls. At
the maximum of the isotherm lines evolves from the cold wall side, and the minimum of the isotherm lines appeared on the hot wall side. In the middle of the portion, there is a square-shaped cell with a single layer. In the absence of
case the heat transfer increases from 0.80078–0.90041. At
the heat generation case the internal heat transmission increases from 0.90041–0.97195. An uplifting value of the
enhances the isotherm magnitude value from 0.80078–0.90041 and 0.97195. Figure (B) demonstrates the influence of
on isotherms contour for
nanofluid. Ethylene glycol has more sites for hydrogen bonding than
As a consequence of this, it is substantially more viscous than water and has strong intermolecular forces of attraction. The raising value of the
parameter enhances the heat transmission and the isotherm magnitude value from 0.9–0.90025 and 0.90289. Physically, the increasing values of
enhance more heat in the interior of the enclosure. This implies that the isotherm profiles exaggerate with the amassed estimation of
. Therefore, the influence of
parameter is more dominant in the
nanofluid compared to the
nanofluid. It can be noted from the contours that the heat transmission enhances in magnitude when
increases on both
and
nanofluid. By replacing the heat absorption
with heat generation
the transfer of heat can be improved up to 15.819% for
nanofluid and 0.596% for
nanoliquid. So, increasing
from −6 to 6 enhances energy transfer in enclosure for both
and
nanofluids.
The fluid flow of the nanoliquids is not remarkably influenced by the volume fraction’s variation. Figure (A-B) reveals that the streamline contour of and
nanoliquids are unchanged as the volume fraction enhances. It is distinguished that in the
nanofluid flow, circulation of the vortices forms in the whole portion of the cavity. In
case,
, the enclosure has three convection cells: top, bottom, and left middle. At
the third cell is merged with primary circulatory cell. At
a new cell appeared at the bottom right of the enclosure.
Figure illustrates the volume fraction factor on isotherm contours for
and
nanofluids. The outcomes of this study exhibit that the heat transmission performance of the considered nanoliquids rises significantly with the volume fraction of the nanoparticles. As the volume fraction increases, energy transfer rates increase due to irregular and unpredictable particle movements, and nanoliquid thermal distribution improves. Figure (A) reveals the
nanofluid for distinct values of
. Mounting values of
parameter enhance isotherm contour magnitude values and energy transmission in the hot side wall directions. The maximum of the isotherm magnitude values rises from 0.90083–0.90086 and 0.90087. Figure (B) reveals the
nanoliquid for distinct values of
. In this case, the maximum of the isotherm lines occurs on the cold wall side, and the heated wall has a high isotherm magnitude value. The rising value of
from 0 to 0.05 transfer of heat can be improved up to 0.278% for
nanofluid and 0.303% for
nanoliquid. So, mounting the value of
from 0 to 0.05 has the tendency to improve the energy transfer within the enclosure for both
and
nanofluids.
Figure shows the streamline contour for various Richardson numbers. The Richardson number represents the mode of convection. Here is the forced convection.
is the mixed convection,
is the natural convection. Figure (A and B) illustrates the
and
nanofluid streamline contour. In both combinations of water conveying iron oxide and ethylene glycol conveying nanofluid, the streamline contour does not change. Water conveying iron oxide nanofluid interior values of the streamline’s magnitude increases from 1 to 1.0001 and 1.0012. Ethylene glycol conveying iron oxide nanofluid interior values of the streamlines magnitude increases from 1 to 1.0001 and 1.0009. Therefore, the influence of
parameter is more dominant in the
nanofluid compared to the
nanofluid.
The transport properties of viscous fluids are significantly influenced by the Reynolds number. The Reynolds number is used in weather forecasting systems. Figure (A) illustrates the nanofluid in a streamline contour. At
the four convective circulation zones are formed, and they are grouped close to the cavity’s right side. At
the circulation cells count decreases, and it appears on both horizontal sides of the enclosure. At
the third circulation cell merged with the primary circulation cell. This implies that the streamline magnitude values rise from 1 to 1.0001. Figure (B) illustrates the
nanofluid in a streamline contour. At
in this case, three circulation zones occurred. At
the third circulation cell, a new cell form in the enclosure’s right midsection., and at the primary circulation cell, the magnitude of the streamlines increases from 1 to 1.0001. At
the number of circulation cells decreases.
Figure (A) demonstrates the local Nusselt number profile of nanofluid, and Figure (B) reveals the local Nusselt number profile of
nanofluid for various values of
. Enlarging the
value from 0 to 0.05, the local rate of heat transmission profiles are enhanced on both
and
nanofluids.
Figure (A) demonstrates the profile of
nanoliquid, and Figure (B) reveals the local Nusselt number profile of
nanofluid for various values of
. Enlarging the
value from −6 to 6, the local rate of heat transmission profiles is enhanced on both
and
cases. An uplifting value of
produced more energy in the interior of the square geometry.
Figure displays the average heat transfer for and
on the bar diagram for a different value of
In
case the raising value of
enhances the mean heat transfer from 1.80372–1.80374 and finally 1.80375. In
case the raising values of
enhance the mean heat transfer from 12.94453–12.94454 and finally 2.94455.
Figure demonstrates the average Nusselt number of and
on the bar diagram for different values of
In
case the raising values of
enhances the mean heat transfer from 1.80375–2.33965 and finally 2.92114. In
case the raising values of
enhances the mean heat transfer from 12.94455–13.04112 and finally 13.13802.
Figure portrays the effect of nanoparticle ( and
) volume fractions on a bar diagram for distinct values of
In
case the raising values of
enhance the mean heat transmission from 1.7988–1.8036 and finally 1.8038. In
case the raising values of
enhance the mean heat transmission from 11.88777–12.51868 and finally 12.94455. In general, the enhancement of
leads to an improvement in overall energy transport; this can be expected owing to the fact that the change in
percentage primarily enhances the thermal conductivity of nanofluid and for this reason, elevates flow strength in both
and
cases.
Tables and illustrate the rate of heat transmission of and
with
and
. The ethylene glycol conveying iron oxide has a higher percentage value compared with the ethylene glycol conveying iron oxide nanofluid. This means that the base fluid ethylene glycol increases, the
on
nanoparticles, raising the values of
and
. The Eckert number improves fluid temperature by improving thermal conduction.
Table 4. Average heat transfer for rising values of .
Table 5. Average heat transfer for rising values of .
5. Conclusions
The present study investigates the influence of the heat transferred MHD mixed convective nanofluid flow inside a square cavity with the effects of heat source/sink, viscous dissipation, and ohmic heating. The thermal analyses of and
are investigated. The enclosure’s four walls are partially heated. The MAC technique is used to resolve the dimensionless governing equations. The outcome of the present results is illustrated via graphs, tables, and a bar chart. This analysis gives the following findings:
The permeability of the porous medium does not affect the velocity on both
and
nanofluids.
Fluid flow diminishes for mounting values of the magnetic field parameter
on both
and
nanofluids.
The influence of
parameter is more dominant in the
nanofluid compared to the
nanofluid flow.
The rising value of
from 0 to 0.05 transfer of heat can be improved up to 0.278% for
nanofluid and 0.303% for
nanofluid.
The heat absorption
with heat generation
the transfer of heat can be improved up to 15.819% for
nanofluid and 0.596% for
nanofluid.
The
increases for rising values of
,
, and
parameter in both
and
nanofluids.
Magnifying
and
values, enhances the
.
In the current analysis, the thermal analysis of MHD-mixed convective nanofluids is evaluated. Forthcoming investigations may extend the present analyses to include hybrid nanofluids in a different geometrical configuration.
Disclosure statement
No potential conflict of interest was reported by the author(s).
References
- Kakaç S, Pramuanjaroenkij A. Review of convective heat transfer enhancement with nanofluids. Int J Heat Mass Transfer. 2009;52:3187–3196. doi:10.1016/j.ijheatmasstransfer.2009.02.006
- Godson L, Raja B, Lal DM, et al. Enhancement of heat transfer using nanofluids – an overview. Renew Sust Energ Rev. 2010;14:629–641. doi:10.1016/j.rser.2009.10.004
- Saidur R, Leong KY, Mohammed HA. A review on applications and challenges of nanofluids. Renew Sust Energ Rev. 2011;15:1646–1668. doi:10.1016/j.rser.2010.11.035
- Sheikholeslami M, Ganji DD. Nanofluid convective heat transfer using semi analytical and numerical approaches: a review. J Taiwan Inst Chem Eng. 2016;65:43–77. doi:10.1016/j.jtice.2016.05.014
- Krishna MV, Chamkha AJ. Hall and ion slip effects on unsteady MHD convective rotating flow of nanofluids – application in biomedical engineering. J Egypt Math Soc. 2020;28:1, doi:10.1186/s42787-019-0065-2
- Minea AA. Hybrid nanofluids based on Al2O3, TiO2 and SiO2: numerical evaluation of different approaches. Int J Heat Mass Transfer. 2017;104:852–860. doi:10.1016/j.ijheatmasstransfer.2016.09.012
- Li Z, Sheikholeslami M, Chamkha AJ, et al. Control volume finite element method for nanofluid MHD natural convective flow inside a sinusoidal annulus under the impact of thermal radiation. Comput Methods Appl Mech Eng. 2018;338:618–633. doi:10.1016/j.cma.2018.04.023
- Alsabery AI, Ismael MA, Chamkha AJ, et al. Mixed convection of Al2O3-water nanofluid in a double lid-driven square cavity with a solid inner insert using Buongiorno’s two-phase model. Int J Heat Mass Transfer. 2018;119:939–961. doi:10.1016/j.ijheatmasstransfer.2017.11.136
- Izadi S, Armaghani T, Ghasemiasl R, et al. A comprehensive review on mixed convection of nanofluids in various shapes of enclosures. Powder Technol. 2019;343:880–907. doi:10.1016/j.powtec.2018.11.006
- Abbas I, Hobiny A, Marin M. Photo-thermal interactions in a semi-conductor material with cylindrical cavities and variable thermal conductivity. J Taibah Univ Sci. 2020;14:1369–1376. doi:10.1080/16583655.2020.1824465
- Mousa MM, Ali MR, Ma WX. A combined method for simulating MHD convection in square cavities through localized heating by method of line and penalty-artificial compressibility. J Taibah Univ Sci. 2021;15:208–217. doi:10.1080/16583655.2021.1951503
- Deshmukh K, Karmare S, Patil P. Experimental investigation of convective heat transfer performance of TiN nanofluid charged U-pipe evacuated tube solar thermal collector. Appl Therm Eng. 2023;225:120199, doi:10.1016/j.applthermaleng.2023.120199
- Zhao D, Hedayat M, Barzinjy AA, et al. Numerical investigation of Fe3O4 nanoparticles transportation due to electric field in a porous cavity with lid walls. J Mol Liq. 2019;293:111537, doi:10.1016/j.molliq.2019.111537
- Hu Y, Shi L, Zhang Z, et al. Magnetic regulating the phase change process of Fe3O4-paraffin wax nanocomposites in a square cavity. Energy Convers Manag. 2020;213:112829, doi:10.1016/j.enconman.2020.112829
- Molana M, Dogonchi AS, Armaghani T, et al. Investigation of hydrothermal behavior of Fe3O4-H2O nanofluid natural convection in a novel shape of porous cavity subjected to magnetic field dependent (MFD) viscosity. J Energy Storage. 2020;30:101395, doi:10.1016/j.est.2020.101395
- Rahman MM, Chamkha AJ, Elmasry Y, et al. The heat transfer behavior of MHD micro-polar MWCNT- Fe3O4/Water Hybrid Nano-fluid in an inclined⊥ shaped cavity with semi-circular heat source inside. Case Stud Therm Eng. 2022;38:102316, doi:10.1016/j.csite.2022.102316
- He W, Zhuang Y, Chen Y, et al. Experimental and numerical investigations on the melting behavior of Fe3O4 nanoparticles composited paraffin wax in a cubic cavity under a magnetic-field. Int J Therm Sci. 2023;184:107961, doi:10.1016/j.ijthermalsci.2022.107961
- Khan MI, Shah F, Waqas M, et al. The role of γAl2O3− H2O and γAl2O3 − C2H6O2 nanomaterials in Darcy-Forchheimer stagnation point flow: an analysis using entropy optimization. Int J Therm Sci. 2019;140:20–27. doi:10.1016/j.ijthermalsci.2019.02.004
- Elshehabey HM, Raizah Z, Öztop HF, et al. MHD natural convective flow of Fe3O4− H2O ferrofluids in an inclined partial open complex-wavy-walls ringed enclosures using non-linear Boussinesq approximation. Int J Mech Sci. 2020;170:105352, doi:10.1016/j.ijmecsci.2019.105352
- Taher MA, Siddiqa S, Kamrujjaman M, et al. Free convection of temperature-dependent thermal conductivity based ethylene glycol-Al2O3 nanofluid in an open cavity with wall heat flux. Int Commun Heat Mass Transf. 2022;138:106379, doi:10.1016/j.icheatmasstransfer.2022.106379
- Farooq U, Waqas H, Muhammad T, et al. Computation of nonlinear thermal radiation in magnetized nanofluid flow with entropy generation. Appl Math Comput. 2022;423:126900, doi:10.1016/j.amc.2021.126900
- Chamkha AJ, Al-Naser H. Double-diffusive convection in an inclined porous enclosure with opposing temperature and concentration gradients. Int J Therm Sci. 2001;40:227–244. doi:10.1016/S1290-0729(00)01213-8
- Groşan T, Revnic C, Pop I, et al. Free convection heat transfer in a square cavity filled with a porous medium saturated by a nanofluid. Int J Heat Mass Transfer. 2015;87:36–41. doi:10.1016/j.ijheatmasstransfer.2015.03.078
- Mehmood K, Hussain S, Sagheer M. Numerical simulation of MHD mixed convection in alumina–water nanofluid filled square porous cavity using KKL model: Effects of non-linear thermal radiation and inclined magnetic field. J Mol Liq. 2017;238:485–498. doi:10.1016/j.molliq.2017.05.019
- Alsabery AI, Mohebbi R, Chamkha A, et al. Effect of local thermal non-equilibrium model on natural convection in a nanofluid-filled wavy-walled porous cavity containing inner solid cylinder. Chem Eng Sci. 2019;201:247–263. doi:10.1016/j.ces.2019.03.006
- Sumithra A, Sivaraj R, Benazir AJ, et al. Nonlinear thermal radiation and activation energy effects on bioconvective flow of Eyring-Powell fluid. Comput Therm Sci. 2021;13:85–99. doi:10.1615/ComputThermalScien.2021039113
- Sumithra A, Sivaraj R. Chemically reactive magnetohydrodynamic mixed convective nanofluid flow inside a square porous enclosure with viscous dissipation and Ohmic heating. Eur Phys J Plus. 2022;137:1193, doi:10.1140/epjp/s13360-022-03409-9
- Sumithra A, Sivaraj R. Impact of exothermic chemical reaction on MHD unsteady mixed convective flow in a rectangular porous cavity filled with nanofluid. Waves Random Complex Media. 2022: 1–22. doi:10.1080/17455030.2022.2139014
- Chamkha AJ. MHD-free convection from a vertical plate embedded in a thermally stratified porous medium with Hall effects. Appl Math Model. 1997;21:603–609. doi:10.1016/S0307-904X(97)00084-X
- Öztop HF, Sakhrieh A, Abu-Nada E, et al. Mixed convection of MHD flow in nanofluid filled and partially heated wavy walled lid-driven enclosure. Int Commun Heat Mass Transf. 2017;86:42–51. doi:10.1016/j.icheatmasstransfer.2017.05.011
- Chamkha AJ, Dogonchi AS, Ganji DD. Magnetohydrodynamic nanofluid natural convection in a cavity under thermal radiation and shape factor of nanoparticles impacts: a numerical study using CVFEM. Appl Sci. 2018;8:2396, doi:10.3390/app8122396
- Krishna MV, Chamkha AJ. Hall effects on MHD squeezing flow of a water-based nanofluid between two parallel disks. J Porous Media. 2019;22:209–223. doi:10.1615/JPorMedia.2018028721
- Krishna MV, Swarnalathamma BV, Chamkha AJ. Investigations of Soret, Joule and Hall effects on MHD rotating mixed convective flow past an infinite vertical porous plate. J Ocean Eng Sci. 2019;4:263–275. doi:10.1016/j.joes.2019.05.002
- Devi NR, Moolya S, Öztop HF, et al. A review on ferrofluids with the effect of MHD and entropy generation due to convective heat transfer. Eur Phys J Plus. 2022;137:482, doi:10.1140/epjp/s13360-022-02616-8
- Geridonmez P, Oztop H. Natural convection in a sinusoidally heated cavity filled with ferrofluid in the presence of partial variable magnetic field. Int J Numer Methods Heat Fluid Flow. 2023;33:411–435. doi:10.1108/HFF-01-2022-0053
- Dogonchi AS, Chamkha AJ, Ganji DD. A numerical investigation of magneto-hydrodynamic natural convection of Cu–water nanofluid in a wavy cavity using CVFEM. J. Therm. Anal. Calorim. 2019; 135:2599–2611. doi:10.1007/s10973-018-7339-z
- Rahman MM, Alim MA, Sarker MMA. Numerical study on the conjugate effect of joule heating and magnato-hydrodynamics mixed convection in an obstructed lid-driven square cavity. Int Commun Heat Mass Transf. 2010;37:524–534. doi:10.1016/j.icheatmasstransfer.2009.12.012
- Ghaffarpasand O. Numerical study of MHD natural convection inside a sinusoidally heated lid-driven cavity filled with Fe3O4-water nanofluid in the presence of Joule heating. Appl Math Model. 2016;40:9165–9182. doi:10.1016/j.apm.2016.05.038
- Kumar M, Reddy GJ, Kumar NN, et al. Reconstruction of an informative railway wheel defect signal from wheel–rail contact signals measured by multiple wayside sensors. Proc Inst Mech. 2019;233:49–62. doi:10.1177/0954409718784362
- Reddy ES, Panda S. MHD natural convection nanofluid flows in a wavy trapezoidal porous enclosure with differentially heated side walls. J Natl Sci Found. 2020;48:57–68. doi:10.4038/jnsfsr.v48i1.9934
- Khanafer KM, Chamkha AJ. Hydromagnetic natural convection from an inclined porous square enclosure with heat generation. Numer Heat Transf A: Appl. 1998;33:891–910. doi:10.1080/10407789808913972
- Rashad AM, Ismael MA, Chamkha AJ, et al. MHD mixed convection of localized heat source/sink in a nanofluid-filled lid-driven square cavity with partial slip. J Taiwan Inst Chem Eng. 2016;68:173–186. doi:10.1016/j.jtice.2016.08.033
- Mansour MA, Siddiqa S, Gorla RSR, et al. Effects of heat source and sink on entropy generation and MHD natural convection of Al 2 O 3 -Cu/water hybrid nanofluid filled with square porous cavity. Therm Sci Eng Prog. 2018;6:57–71. doi:10.1016/j.tsep.2017.10.014
- Alsabery AI, Gedik E, Chamkha AJ, et al. Effects of two-phase nanofluid model and localized heat source/sink on natural convection in a square cavity with a solid circular cylinder. Comput Methods Appl Mech Eng. 2019;346:952–981. doi:10.1016/j.cma.2018.09.041
- Tian Z, Shahsavar A, Al-Rashed AA, et al. Numerical simulation of nanofluid convective heat transfer in an oblique cavity with conductive edges equipped with a constant temperature heat source: Entropy production analysis. Comput Math Appl. 2021;81:725–736. doi:10.1016/j.camwa.2019.12.007
- Yang Y, Pu W, Yao Z, et al. Experimental and numerical investigations on the intermittent heat transfer performance of rectangular cavity plate fin phase change material based heat sink. J Energy Storage. 2023;60:106607, doi:10.1016/j.est.2023.106607
- El Moutaouakil L, Boukendil M, Hidki R, et al. Analytical solution for natural convection of a heat-generating fluid in a vertical rectangular cavity with two pairs of heat source/sink. Therm Sci Eng Prog. 2023;40:101738, doi:10.1016/j.tsep.2023.101738
- Sohani A, Shahverdian MH, Sayyaadi H, et al. An optimum energy, economic, and environmental design based on DEVAP concept to reach maximum heat recovery in a PV-wind turbine system with hydrogen storage. Energy Convers Manag. 2023;288:117147, doi:10.1016/j.enconman.2023.117147
- Wang D, Feng D, Peng H, et al. Implications of steep hilly terrain for modeling wind-turbine wakes. J Clean Prod. 2023;398:136614, doi:10.1016/j.jclepro.2023.136614
- Sohani A, Cornaro C, Shahverdian MH, et al. Building integrated photovoltaic/thermal technologies in Middle Eastern and North African countries: Current trends and future perspectives. Renew Sustain Energy rev. 2023;182:113370, doi:10.1016/j.rser.2023.113370
- Harlow FH, Welch JE. Numerical calculation of time-dependent viscous incompressible flow of fluid with free surface. Phys Fluids. 1965;8:2182–2189. doi:10.1063/1.1761178