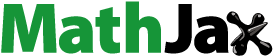
Abstract
Antimony selenide thin films of 140–190 nm in thickness, with different compositions, were prepared at room temperature by using the dual-thermal evaporation technique. The compositional, structural, morphological and optical studies were performed in the as-deposited state and after annealing at 250°C for an hour, using many analytical techniques. The carrier-type, resistivity, carrier concentration and mobility of the annealed films were determined. The obtained thin films, regardless of their composition, exhibited a smooth surface with a high absorption coefficient of greater than 105 cm−1. The optical band gap of the near-stoichiometric film was 1.56 eV, which decreased to 1.32 eV after annealing. However, higher values were determined with increasing Se elements in the films, but these values did not exceed 1.77 eV. This study shows that antimony selenide thin films can be obtained at room temperature using a thermal evaporation technique, and the properties of the films can be improved with annealing.
1. Introduction
Thin-film solar cells rely on inorganic absorbers such as copper indium gallium selenide Cu(In,Ga)Se2 and Cadmium telluride (CdTe) to achieve high device stability and efficiency [Citation1–3]. However, the high cost of gallium and indium, as well as cadmium’s high toxicity, may preclude further developments of Cu(In,Ga)Se2 and CdTe solar cells. In recent years, perovskite-based solar cells have taken the lead role as their conversion efficiency has the highest reported value of 28%, superseding Cu(In,Ga)Se2 and CdTe [Citation4]. However, the low stability of the absorber layer and the high toxicity of lead could prevent the commercialization of these solar cells [Citation5]. Hence, it is critical to find a new eco-friendly and earth-abundant material for thin-film solar cells.
Antimony selenide (Sb2Se3) is an earth-abundant non-toxic material that has a high absorption coefficient (greater than 105 cm−1), a direct bandgap of (1.1–1.3) eV and a long carrier lifetime (around 60 ns) [Citation6–9]. These good properties make antimony selenide an extremely appropriate absorber layer for thin-film solar cells. According to the theoretical studies, the conversion efficiency of Sb2Se3 can reach 30% [Citation10]. Experimentally, the conversion efficiency of a Sb2Se3 solar cell rises quickly from 1.9% to 7.6%, though it has been investigated for only a few years [Citation8,Citation11–13]. Consequently, antimony selenide has grown in importance among researchers worldwide.
Many methods have been used to prepare Sb2Se3 thin films, such as e-beam evaporation [Citation14], electrodeposition [Citation15,Citation16] and chemical bath deposition [Citation17,Citation18]. Indeed, many studies of Sb2Se3 thin-film solar cells based on solution methods are low-cost methods [Citation19–28]. However, these methods employ toxic chemicals and solvents or easily introduce impurities into the film as a result of conducting the processes in a non-vacuum environment. Vacuum-based methods are reliable and simple and have been used in the industrial production of CdTe and Cu(In,Ga)Se2 solar cells [Citation11,Citation29]. Additionally, Sb2Se3 has a high vapour pressure [Citation30] and low melting point [Citation21] and prefers easy thermal evaporation over magnetron sputtering [Citation5,Citation31]. Some groups have deposited Sb2Se3 thin films by thermal evaporation at high substrate temperatures, using commercially available Sb2Se3 powder as a source material [Citation1,Citation11]. Other researchers prepared the films by co-evaporation and selenization of the metal stack grown with sputtering [Citation32,Citation33]. In both methods, a large amount of Se powder should be used. Especially with increasing substrate temperature because of the high sublimation rate of Sb2Se3 from the substrate [Citation32,Citation34]. In this paper, we prepare antimony selenide thin films with different compositions directly using the dual-thermal evaporation technique at room temperature. The prepared samples were annealed at 250°C for an hour. The detailed characterization by means of EDX, XRD, Raman spectroscopy, AFM and UV/VIS/NIR spectroscopy, 4-point probe and Hall system has been discussed in the present study.
2. Experimental details
Antimony selenide thin films were fabricated utilizing a homemade dual-thermal evaporation technique, following the methods explained in reference [Citation35] for the deposition of Cu(InGa)Se2 thin films. Two boats are used, with selenium (Se: 99.99%) powder placed in boat 1 (applying a current of 50 A) and antimony (Sb: 99.99%) shot (1–2 mm) placed in boat 2 (applying a current of about 100 A). The name of the supplier of the chemicals is SIGMA-ALDRICH. In this context, one should note that the used approach in this study to deposit antimony selenide thin films requires a separation of the two boats. This separation is necessary to vary the evaporation temperature. The boats were heated instantly to deposit the films on the glass substrates at room temperature. Thin films with different compositions were prepared by weighing Se and Sb powders in different amounts. All films were grown at a high vacuum of 10−5 mbar. The deposited thin films were then annealed in a tube furnace in a nitrogen atmosphere at 250°C for an hour.
The compositional, structural, morphological and optical studies were performed in the as-deposited films and also after annealing using different techniques. EDX, which is attached to a desktop scanning electron microscope (JEOL- JCM 6000), was used to determine the chemical composition. The crystal structure of the films was studied with X-ray diffraction (Shimadzu XRD-6000), using a CuKα radiation source produced at an X-ray tube current and voltage of 30 mA and 40 kV, respectively. Also, Raman spectroscopy (Bruker Senterra) was employed to study the structure of the prepared thin films, using an excitation laser with a wavelength of 532 nm (5 mW). The surface morphology was studied by atomic force microscopy (AFM) (Veeco CP-II) in non-contact mode, utilizing Si tips at a 1 Hz scan rate. A UV–VIS–NIR spectrophotometer (Shimadzu) was used at room temperature to investigate the optical properties of the prepared samples with a resolution of 0.1 nm. A stylus profilometer (Dektak150) was used to determine the thicknesses of the films. Also, the electrical resistivity of the films was investigated by a computerized 4-point probe system using a Keithley 2420 instrument. The carrier-type, carrier concentration and mobility were measured using a Hall system at room temperature (Ecopia HMS 3000 Hall measurement system).
3. Results and discussion
Five samples with various compositions were prepared by means of a dual-thermal evaporation technique. The EDX spectra of the deposited thin films are shown in Figure (a). The samples were named as SS-0.6, SS-1, SS-1.5, SS-2 and SS-3, depending on the atomic ratio of the Se/Sb as shown in Table . The sample SS-1.5 shows a near-stoichiometric Sb2Se3 thin film as it illustrates atomic ratios of Se/Sb = 1.48. The samples SS-2 and SS-3 reveal Sb-poor thin films with different atomic concentration ratios of Se/Sb, which are 2.04 and 3.09, respectively. However, the sample SS-0.6 reveals an Sb-rich thin film with an atomic ratio of Se/Sb of 0.61. Moreover, nearly equal concentrations of the elements are revealed in SS-1, with a 1.11 atomic ratio of Se/Sb.
Figure 1. EDX spectra of the prepared thin films. (a) as-deposited thin films and (b) after annealing

Table 1. Elemental compositions of as-deposited thin films.
The EDX spectra of the films after annealing are illustrated in Figure (b). The annealing process slightly decreased the atomic percentage of Se for all films, as shown in Table . This may be because of a weak bond of these atoms to the sample surface.
Table 2. Elemental compositions of thin films after annealing.
XRD and Raman spectroscopy were employed to characterise the structural properties of the fabricated thin films before and after annealing. As illustrated in Figure , the XRD diffraction peaks of the as-deposited samples SS-1, SS-1.5, SS-2 and SS-3 match the available XRD pattern for Sb2Se3 (JCPDS 15-0861) [Citation11,Citation36]. Mainly, for SS-1, a broad peak (130) can be detected with a small intensity, which indicates inadequate crystallization. However, for the three samples SS-1.5, SS-2 and SS-3; (130) and (212) peaks are observed. In addition, (211) and (240) peaks are seen with small intensities in the near-stoichiometric sample SS-1.5, whereas SS-0.6 showed no peak, which means an amorphous film. The Raman spectra of these deposited thin films are shown in Figure . No peak is detected for sample SS-0.6. However, the characteristic peaks for SS-1, SS-1.5 and SS-3 samples agree with previous studies reported in the literature. The bands arising at about 83, 175, 192 and 188 cm−1 are due to vibrational modes of the Sb2Se3 phase [Citation14,Citation27,Citation36,Citation37]. The Raman spectra of the SS-1.5 near-stoichiometric thin film reveal a peak at around 192 cm−1; this is generally assigned to the Se–Sb stretching vibration mode. Also, the peak observed at 188 cm−1 for the SS-3 sample appears because of the stretching of the Se–Sb–Se bonds.
The crystallinity of the prepared samples was improved with annealing, as shown in Figure . The XRD diffraction peaks of all samples after annealing match with the available XRD pattern for Sb2Se3 (JCPDS 15-0861) [Citation11,Citation36].
This result was confirmed with Raman spectroscopy, as shown in Figure . The detected peaks for all annealed samples agree with the reported previous studies. The detected peaks at about 184, 187, 155, 190 and 192 cm−1 are generally attributed to vibrational modes of the Sb2Se3 phase [Citation6,Citation11,Citation37,Citation38].
The crystallite size (D) of the annealed thin films was calculated using Scherrer's equation:
where λ is the wavelength of X-rays (1.54 Å for Cu-Kα radiation), θ is Bragg’s angle and β is the FWHM of the (120) XRD peak in radians. The crystallite size of the samples varies between 151.67 and 154.20 Å for all samples except SS-3, as shown in Table . The crystal grain diameter of the Se-extra film, SS-3, is about 240 Å may be due to the increase of Se in the film.
Table 3. Crystallite size of the annealed thin films.
The surface morphology of the samples was investigated by AFM, as shown in Figures and . All the deposited films display a smooth surface with a root mean square roughness of only a few nm. Also, it can be seen that all surfaces are composed of small grains that are distributed over the surfaces. However, annealing led to improvements in the morphology of the surfaces, as shown in Figure .
The optical properties of the deposited and annealed thin films were examined by a UV–VIS–NIR spectrometer. The absorption spectra of the deposited thin films are shown in Figures and , respectively. Generally, absorbance reveals good values for all films in the visible region before and after annealing. Before annealing, the samples SS-0.6 and SS-1 clearly have a higher value than other deposited samples. No big change was seen in these samples after annealing. However, annealing led to improved absorbance values in the samples SS-1.5, SS-2 and SS-3.
For the calculation of the absorption coefficient (α) of the films, we used the following formula:
(1)
(1) where T is the optical transmission, R is the reflectance and d is the thickness of the prepared film [Citation4]. We found that all prepared films, before and after annealing, exhibit a high absorption coefficient of greater than 105 cm−1, which matches the reported value in the literature [Citation7,Citation9]. To estimate the optical energy gap (Eg), we used the following formula for direct band gap materials:
(2)
(2) where A is a constant and υ is the light frequency. We plotted (αhν)2 versus hν, as shown in Figures and . The Eg values of the sample SS-1.5 were 1.56 eV for the as-deposited film and 1.32 eV after annealing. The reduction of energy gap after annealing has been also observed by Tang et al. [Citation39]. Obviously, this value of around 1.3 eV agrees well with the reported values for the stoichiometric Sb2Se3 [Citation14,Citation27]. The Eg values were 1.16 and 1.42 eV for as-deposited films in SS-0.6 and SS-1 samples, respectively. These values changed after annealing to 1.25 and 1.16 eV. On the other hand, SS-2 and SS-3 samples illustrate Eg values of 1.72 and 1.77 eV for as-deposited films, which decreased after annealing to 1.45 and 1.50 eV, respectively. Generally, increasing Se elements in the films leads to an increase in Eg, compared to the stoichiometric Sb2Se3. This observation matches the result reported by Tiwari et al., where increasing Se leads to an increase in Eg [Citation14].
Figure 10. The plot of (αhν)2 versus hν of deposited thin films with different atomic ratios (Se/Sb).

The resistivity, carrier concentration, carrier-type and mobility of the annealed thin films were listed in Table . Clearly, increasing Se elements in the films leads to an increase in the resistivity regardless of the carriers-type, which shows the higher values with the sample SS-2 and SS-3. These samples illustrate the lower values of carrier concentration in comparison to other films. However, the variation in the mobility values is small and SS-1.5 shows the highest value of mobility.
Table 4. Resistivity, carrier concentration and mobility of the annealed thin films.
4. Conclusion
In this work, the dual-thermal evaporation technique has been successfully used to deposit antimony selenide thin films at room temperature. Films with different compositions have been prepared to study the effect of the composition on their properties. The obtained thin films, before and after annealing, exhibited a smooth surface with a high absorption coefficient of greater than 105 cm−1, regardless of their composition. The band gap of the near-stoichiometric film was 1.56 eV, which was reduced to 1.32 eV after annealing. However, higher values were defined with increasing Se elements in the films, but these values do not go over 1.77 eV. Electrical measurements show that increasing Se elements in the films leads to an increase in the resistivity and reduction in carrier concentration regardless of the carrier type. However, the variation in the mobility values is small and SS-1.5 shows the highest value of mobility. This study shows that antimony selenide thin films can be obtained at room temperature using a thermal evaporation technique, and the properties of the films can be improved with annealing.
Acknowledgements
The authors extend their appreciation to the Deputyship forResearch & Innovation, Ministry of Education in Saudi Arabia for funding this research work through the projectnumber 442/101. Also, the authors would like to extend their appreciation to Taibah University for its supervisionsupport.
Disclosure statement
No potential conflict of interest was reported by the author(s).
Additional information
Funding
References
- Zhou Y, Wang L, Chen S, et al. Thin-film Sb2Se3 photovoltaics with oriented one-dimensional ribbons and benign grain boundaries. Nat Photonics. 2015;9(6):409–415. doi:10.1038/nphoton.2015.78
- Jackson P, Hariskos D, Wuerz R, et al. Properties of Cu (In, Ga) Se2 solar cells with new record efficiencies up to 21.7%. Phys Status Solidi (RRL)–Rapid Res Lett. 2015;9(1):28–31. doi:10.1002/pssr.201409520
- Green MA, Dunlop E, Hohl-Ebinger J, et al. Solar cell efficiency tables (version 52). Prog Photovoltaics Res Appl. 2018;26(7):427–436. doi:10.1002/pip.3040
- Singh P, Ghorai N, Thakur A, et al. Temperature-dependent ultrafast charge carrier dynamics in amorphous and crystalline Sb2Se3 thin films. J Phys Chem C. 2021;125(9):5197–5206. doi:10.1021/acs.jpcc.0c11327
- Tang R, Chen XY, Liang GX, et al. Magnetron sputtering deposition and selenization of Sb2Se3 thin film for substrate Sb2Se3/CdS solar cells. Surf Coat Technol. 2019;360:68–72. doi:10.1016/j.surfcoat.2018.12.102
- Kumar V, Artegiani E, Kumar A, et al. Effects of post-deposition annealing and copper inclusion in superstrate Sb2Se3 based solar cells by thermal evaporation. Sol Energy. 2019;193:452–457. doi:10.1016/j.solener.2019.09.069
- Li Z, Liang X, Li G, et al. 9.2%-efficient core-shell structured antimony selenide nanorod array solar cells. Nat Commun. 2019;10(1):1–9. doi:10.1038/s41467-018-07882-8
- Wen X, Chen C, Lu S, et al. Vapor transport deposition of antimony selenide thin film solar cells with 7.6% efficiency. Nat Commun. 2018;9(1):1–10. doi:10.1038/s41467-017-02088-w
- Spalatu N, Krautmann R, Katerski A, et al. Screening and optimization of processing temperature for Sb2Se3 thin film growth protocol: interrelation between grain structure, interface intermixing and solar cell performance. Sol Energy Mater Sol Cells. 2021;225:111045. doi:10.1016/j.solmat.2021.111045
- Liu X, Chen C, Wang L, et al. Improving the performance of Sb2Se3 thin film solar cells over 4% by controlled addition of oxygen during film deposition. Prog Photovoltaics Res Appl. 2015;23(12):1828–1836. doi:10.1002/pip.2627
- Liu X, Chen J, Luo M, et al. Thermal evaporation and characterization of Sb2Se3 thin film for substrate Sb2Se3/CdS solar cells. ACS Appl Mater Interfaces. 2014;6(13):10687–10695. doi:10.1021/am502427s
- Leng M, Luo M, Chen C, et al. Selenization of Sb2Se3 absorber layer: an efficient step to improve device performance of CdS/Sb2Se3 solar cells. Appl Phys Lett. 2014;105(8):083905. doi:10.1063/1.4894170
- Wang L, Li DB, Li K, et al. Stable 6%-efficient Sb2Se3 solar cells with a ZnO buffer layer. Nature Energy. 2017;2(4):1–9. doi:10.1038/nenergy.2017.46
- Tiwari KJ, Ren MQ, Vajandar Sk, et al. Mechanochemical bulk synthesis and e-beam growth of thin films of Sb2Se3 photovoltaic absorber. Sol Energy. 2018;160:56–63. doi:10.1016/j.solener.2017.11.074
- Lai Y, Chen Z, Han C, et al. Preparation and characterization of Sb2Se3 thin films by electrodeposition and annealing treatment. Appl Surf Sci. 2012;261:510–514. doi:10.1016/j.apsusc.2012.08.046
- Shi X, Tian Y, Shen C, et al. Electrodeposition of Sb2Se3 on indium-doped tin oxides substrate: nucleation and growth. Appl Surf Sci. 2012;258(6):2169–2173. doi:10.1016/j.apsusc.2011.02.097
- Rodríguez-Lazcano Y, Pena Y, Nair MTS, et al. Polycrystalline thin films of antimony selenide via chemical bath deposition and post deposition treatments. Thin Solid Films. 2005;493(1–2):77–82. doi:10.1016/j.tsf.2005.07.238
- Maghraoui-Meherzi H, Nasr TB, Dachraoui M. Synthesis, structure and optical properties of Sb2Se3. Mater Sci Semicond Process. 2013;16(1):179–184. doi:10.1016/j.mssp.2012.04.019
- Choi YC, Mandal TN, Yang WS, et al. Sb2Se3-sensitized inorganic–organic heterojunction solar cells fabricated using a single-source precursor. Angew Chem. 2014;126(5):1353–1357. doi:10.1002/ange.201308331
- Ngo TT, Chavhan S, Kosta I, et al. Electrodeposition of antimony selenide thin films and application in semiconductor sensitized solar cells. ACS Appl Mater Interfaces. 2014;6(4):2836–2841. doi:10.1021/am405416a
- Zhou Y, Leng M, Xia Z, et al. Solution-processed antimony selenide heterojunction solar cells. Adv Energy Mater. 2014;4(8):1301846. doi:10.1002/aenm.201301846
- Choi YC, Lee YH, Im SH, et al. Efficient inorganic-organic heterojunction solar cells employing Sb2 (Sx/Se1-x) 3 graded-composition sensitizers. Adv Energy Mater. 2014;4(7):1301680. doi:10.1002/aenm.201301680
- Calixto-Rodriguez M, Garcia HM, Nair MTS, et al. Antimony chalcogenide/lead selenide thin film solar cell with 2.5% conversion efficiency prepared by chemical deposition. ECS J Solid State Sci Technol. 2013;2(4):Q69. doi:10.1149/2.027304jss
- Lokhande C, Sankapal BR, Sartale SD, et al. A novel method for the deposition of nanocrystalline Bi2Se3, Sb2Se3 and Bi2Se3–Sb2Se3 thin films—SILAR. Appl Surf Sci. 2001;182(3–4):413–417. doi:10.1016/S0169-4332(01)00461-5
- Rajpure K, Bhosale C. Effect of Se source on properties of spray deposited Sb2Se3 thin films. Mater Chem Phys. 2000;62(2):169–174. doi:10.1016/S0254-0584(99)00173-X
- Lai Y, Han C, LV x, et al. Electrodeposition of antimony selenide thin films from aqueous acid solutions. J Electroanal Chem. 2012;671:73–79. doi:10.1016/j.jelechem.2012.02.018
- Yang J, Lai Y, Fan Y, et al. Photoelectrochemically deposited Sb2Se3 thin films: deposition mechanism and characterization. RSC Adv. 2015;5(104):85592–85597. doi:10.1039/C5RA16055C
- Choi YC, Lee DU, Noh JH, et al. Highly improved Sb2Se3 sensitized-inorganic–organic heterojunction solar cells and quantification of traps by deep-level transient spectroscopy. Adv Funct Mater. 2014;24(23):3587–3592. doi:10.1002/adfm.201304238
- Jackson P, Hariskos D, Lotter E, et al. New world record efficiency for Cu (In, Ga) Se2 thin-film solar cells beyond 20%. Prog Photovoltaics Res Appl. 2011;19(7):894–897. doi:10.1002/pip.1078
- Speight J. Lange’s handbook of chemistry. New York: McGraw-Hill; 2005.
- Carcia P, Mclean RS, Reilly MH, et al. Transparent ZnO thin-film transistor fabricated by rf magnetron sputtering. Appl Phys Lett. 2003;82(7):1117–1119. doi:10.1063/1.1553997
- Li Z, Chen X, Zhu H, et al. Sb2Se3 thin film solar cells in substrate configuration and the back contact selenization. Sol Energy Mater Sol Cells. 2017;161:190–196. doi:10.1016/j.solmat.2016.11.033
- Yuan C, Jin X, Jiang G, et al. Sb2Se3 solar cells prepared with selenized dc-sputtered metallic precursors. J Mater Sci: Mater Electron. 2016;27(9):8906–8910. doi:10.1007/s10854-016-4917-3
- Alamri S, Alsadi M. Growth of Cu (In, Ga) Se2 thin films by a novel single flash thermal evaporation source. J Taibah Univ Science. 2020;14(1):38–43. doi:10.1080/16583655.2019.1701389
- Alamri S, Almohammadi A. The effect of substrate temperature on Cu (In, Ga) Se2 layers deposited by dual thermal evaporation. J Taibah Univ Sci. 2021;15(1):442–448. doi:10.1080/16583655.2021.1978808
- Liang G-X, Zhang XH, Ma HL, et al. Facile preparation and enhanced photoelectrical performance of Sb2Se3 nano-rods by magnetron sputtering deposition. Sol Energy Mater Sol Cells. 2017;160:257–262. doi:10.1016/j.solmat.2016.10.042
- Goyal D, Goyal CP, Ikeda H, et al. Study of CuSbSe2 thin films grown by pulsed laser deposition from bulk source material. Mater Sci Semicond Process. 2021;121:105420. doi:10.1016/j.mssp.2020.105420
- Ma X, Zhang Z, Wang X, et al. Large-scale growth of wire-like Sb2Se3 microcrystallines via PEG-400 polymer chain-assisted route. J Cryst Growth. 2004;263(1-4):491–497. doi:10.1016/j.jcrysgro.2003.11.004
- Tang R, Chen S, Zheng ZH Heterojunction annealing enabling record open-circuit voltage in antimony triselenide solar cells. Adv Mater. 2022;34(14):2109078. doi:10.1002/adma.202109078