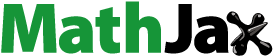
Abstract
This study explores green-synthesized delafossite CuAlO2 as a hole transport layer (HTL) in a CH3NH3SnI3-based perovskite solar cells (PSCs) with an FTO/CuAlO2/CH3NH3SnI3/WO3/Au structure. The performances of the cell have been theoretically investigated using SCAPS-1D. Interface defects significantly impact cell efficiency, as defect density increases from 1014 cm−3 to 1020 cm−3 efficiency reducing from 25.3% to 24.45% in the HTL/perovskite junction and from 25.2% to 17.8% in the perovskite/electron transport layer (ETL) interface. PCBM as buffer layer at perovskite/ETL interface compensates for power conversion efficiency (PCE) losses. Optimizing parameters reveals the delafossite CuAlO2-based, lead-free perovskite solar cell achieving peak efficiency at 26.74%, with VOC, JSC, and FF values of 0.99 V, 33.43 mA cm−2, and 81.05%, respectively. This research underscores delafossite CuAlO2 as a promising HTL for eco-friendly, stable CH3NH3SnI3-based perovskite solar cells, emphasizing its potential in enhancing device performance.
1. Introduction
Over the last few years, much time and efforts have been given in improving the efficiency and stability of perovskite solar cells (PSCs). PSC is a challenger to the most commercially established silicon solar cell due to its easy processing and fabrication technique, low cost, variation in composition, wide range of spectrum absorption ability [Citation1–6]. Environmental issues such as moisture, oxygen, light, heat, intrinsic molecular dissociation, and ion movement inside perovskites are all important factors that impact the stability of PSCs [Citation7,Citation8]. The main restriction of PSCs before industrialization is device durability. Due to the features such as long-term durability, cost-effectiveness, low-temperature processability, and decreased hysteresis properties, inverted PSCs have been explored extensively. Compared to the record 25.7% power conversion efficiency (PCE) of regular perovskite solar cell structure, inverted PSC declares 22.1% highest PCE [Citation9–12]. Pb toxicity is another major problem; thus, researchers have explored device performance by substituting Pb with Sn, Ge, Cu, Bi, and so on, but the findings are not satisfactory. Among them, tin (Sn) based perovskites are a potential candidate in this area because of their appropriate bandgap, outstanding photovoltaic characteristics, low manufacturing cost, great performance, and environmental friendliness [Citation13–15]. The oxidation of Sn2+ to Sn4+ in tin-based perovskites is a critical challenge that affects the stability and performance of these materials in solar cells. Encapsulation and passivation techniques are vital strategies to counteract this issue and improve the stability of tin perovskite-based devices. Despite several efforts, the maximum efficiency is found at 13.24% for SnX3-based PSCs and stability at 3800 h, which are both lower than the Shockley–Queasier (SQ) limit [Citation16–19]. There is still plenty of space for advancement in SnX3-based PSCs.
Material engineering regarding the hole transport material (HTM) in inverted PSCs plays a vital role in cell performance because light penetrates through the HTM before absorbed by the perovskite in this structure. So, optoelectronic properties of the HTM should match with the perovskite layer. Organic HTMs such as Spiro-OMeTAD, PTAA, PEDOT: PSS, P3HT, etc. are commonly used HTMs in perovskite solar cells [Citation20]. Improving the electrical properties of organic hole transport layer (HTL) materials require doping or the addition of chemicals, which causes the perovskite layer to degrade rapidly. To resolve these restrictions, scientists have used the most robust, inexpensive, and abundant inorganic materials as appropriate HTMs for PSCs. Delafossite oxides (ABO2 where, “A” monovalent, “B” trivalent cation) are attractive HTL for perovskite solar cells due to their wide band gap, excellent energy band alignment relative to the perovskite layer, and ease of manufacturing [Citation21,Citation22]. For p-i-n type PSC structures, CuAlO2 has higher optical transparency in the visible band while maintaining excellent electrical conductivity [Citation23].
Femi Igbari et al. investigated the CuAlO2 hole interfacial layer and obtained a maximum of 14.52% PCE [Citation24]. Achilleas Savva et al. researched CuAlO2/Cu–O hole-selective contact and obtained 16.3% PCE with negligible hysteresis effect. They also found near 80% transparency for CuAlO2 in the visible wavelength range. Mona Shasti et al. simulated MAPbI3-based perovskite solar cells using CuAlO2 as HTM and obtained 19.82% PCE [Citation25]. Fanliang Bao et al. fabricated PSCs with CuAl(M)O2 HTL in CsPbBr3-based perovskite solar cells [Citation26]. They declared that the oxygen movement and structure in CuAl(Sc)O2 boosted electric charge flow, making solar cells using this material 10.13% efficient, compared to 6.5% with HTL-free device. Shayan Tariq Jan et al. investigated the n-i-p and p-i-n structures for MAGeI3 PSCs, using CuAlO2 as the HTL, and found that the p-i-n structure showing a 24.32% of efficiency, while the n-i-p structure provides a 14.82% PCE [Citation27].
The primary novelty of this study lies in the synthesis methodology employed, specifically, the utilization of green Aloe-vera extract to synthesize CuAlO2. In addition to providing a distinctive, environmentally friendly method, this novel approach also addresses the rising significance of sustainable practices in material synthesis. The p-i-n structure with Pb-free perovskite and inorganic charge transport layers (CTLs) was studied in this work. The objective of this work is to utilize green synthesized CuAlO2 as HTL in the PSC device structure in the configuration of FTO/CuAlO2/CH3NH3SnI3/WO3/Au. The materials used in the proposed device are environmentally benign, abundant, and inexpensive. Delafossite CuAlO2 as HTL and WO3 as ETL [Citation28–31] are both promising materials for dramatically improving device performance; specifically, inorganic CTLs enhance stability. By the sol–gel method, synthesized CuAlO2 has been investigated by XRD and FTIR studies, which verified the material properties. The optical properties of the prepared CuAlO2 were also determined. The device performance was then simulated in preparation for actual device fabrication. Variations in Absorber thickness, HTL and ETL thickness, carrier concentration, interfacial defect, series and shunt resistance, Mott–Schottky capacitance studies, and temperature effect have been investigated to optimize the device.
2. Methodology
This study was conducted in two parts: (i) experimental and (ii) numerical simulation. In the experimental part, synthesized CuAlO2 was analysed using XRD, UV-Vis, and FTIR techniques. In the second part, a perovskite solar cell (p-i-n) has been designed with characterized CuAlO2 as hole transport material, and its PV properties have been studied by the SCAPS-1D simulation programme.
2.1. Materials
CuAlO2 was synthesized by a green sol–gel process. Aloe vera-water extract, which acted as a green chelating/complexing agent, was used [Citation32]. Other chemicals Cu(NO3)2.3H2O, Al(NO3)3.9H2O were used as the sources of Cu and Al, respectively. All chemicals were AR grade, collected from Sigma-Aldrich, and used directly without further purification.
2.2. Collection of plant extract
Initially, the collected Aloe Vera stems were washed several times with DI water before being dried in normal conditions and sliced. Each 15 g Aloe Vera slice was placed in a glass beaker with 100 mL DI water and stirred for 1 h on a hot plate at 60°C. After the extraction was finished, the mixture was filtered, and the filtrate was stored in the refrigerator to be used as a chelating or complexing agent during the synthesis process.
2.3. Preparation of CuAlO2
Millimole equivalents of 0.241 g Cu(NO3)2.3H2O and 0.38 g Al(NO3)3.9H2O were weighed out and mixed into 100 mL Aloe Vera extract separately in two conical flasks while gently shaking to mix up homogeneously. In a 500 mL glass beaker, both coloured mixtures were warmed at 80°C with continuous stirring until the mixture was transferred into a clear solution. The solution was evaporated at 100–120°C until it became a light greenish gelatinous mass. The mass in gel form was dried completely in an oven at 150°C for 12 h. Finally, the dried powder material was calcined for 6 h at 500°C to yield the desired product. The entire synthesis process is depicted schematically in Figure .
2.4. Material characterization
Phase structure of the synthesized CuAlO2 was recorded by X-ray diffraction (XRD: Diffractometer; Bruker AXS D8 ADVANCE) equipped with Cu Kα radiation. The Fourier transmittance infrared (FTIR) spectroscopy is equipped with a Bruker Vertex-70 spectrometer, and a Hyperion 3000 IR microscope was used to observe the transmittance spectral response of the sample. To study the optical nature of the material, UV-Vis spectroscopy was carried out by a spectrophotometer (Perkin Elmer Lambda-35).
3. Results and discussion
3.1. XRD study
Figure illustrates the XRD outcomes of CuAlO2 prepared by the green synthesis route. The diffraction peaks of the film have been recognized as (006), (101), (102), (012), (104), (009), and (108) delafossite structures with the Rm space cluster. Measured crystallite size, dislocation densities, strain, and lattice parameters are 23.40 nm, 1.60 × 1011 cm−3, 4.79 × 10−3, and 3.51 Å, respectively. Figure (b) represents the elemental arrangement in the lattice of a unit cell. The green colour sphere is an Al atom, which is octahedrally connected with the deep ash-coloured sphere oxygen atoms and reddish sphere Cu ions are stacked inside by the layered of (Al2O6)−1 [Citation33].
3.2. Optical properties
The UV-Vis absorbance profile of the pure phase CuAlO2 is exposed in Figure (a). The direct Eg can be determined by using absorption onset (355 nm) as in the below equation.
(1)
(1) The direct optical Eg is assessed from the Tauc plot in Figure (b) around 3.5 eV. This Eg is reliable with Eg (3.5 eV) estimated for CuAlO2 thin film grown by pulsed laser deposition (PLD) method [Citation34].
3.3. FTIR study
The FTIR study provides important information about metal–oxygen stretching, ion sites, and the presence of functional groups in the sample [Citation35]. The spectra exhibit a broad peak at 604 cm−1, which covers the transmittance ranging frequency wave number from 550 cm−1 to 900 cm−1 (Figure ). The next three peaks are obtained at 1066, 1242, and 1404 cm−1, respectively. Another two close signals are found at around 2901, and 2987 cm−1 and the last peak appeared at 3665 cm−1. The broad peak at 604 cm−1 is the result of the convolution of several smaller peaks. This peak is assigned to the transmittance peaks of Cu-O, Al–O bond vibrations. Peaks in the 800–900 cm−1 range may be attributed to short Al–O stretching vibrations in distorted AlO6 octahedral CuAlO2 [Citation36]. Peak around 1000 cm−1 may be assigned to Al–O-C, or Cu-O–C [Citation37]. The peak appears at 2901 cm−1 due to C−H stretching and peaks at 2987 cm−1 wavenumber or above due to O-H bond stretching, which may have been incorporated from the atmosphere or phytochemicals used in the precursor [Citation38].
4. Device modelling for simulation
The 2nd part of the study has been presented in this section. The proposed device, in which synthesized CuAlO2 was considered as HTL, was studied using SCAPS-1D simulation software. From the experimental part, it was found that the CuAlO2 exhibited an energy bandgap of 3.5 eV, which is higher than that of some promising inorganic HTMs such as CuO2 (2.45 eV), CuSCN (3.40 eV), CuCrO2 (3.30 eV), SrCu2O2 (3.30 eV) [Citation39,Citation40]. As a higher energy bandgap of the window layer in PSCs can pass more light to the absorber layer by minimizing the charge leakage, charge separation, and charge transfer [Citation41], experimentally synthesized CuAlO2 would be considered a suitable HTL, through which solar radiation would travel to the perovskite absorber layer for the proposed p-i-n structured PSC. Table displays the reference data. Regarding holes and electrons, SCAPS-1D adheres to Poisson's calculation and the continuity equation. To get the simulated results realistic enough, SCAPS-1D considers the different types of defects, both in bulk and at the interfaces. Using the defect density values, the simulation provides cell performance parameters that match the experimental performances, confirming the validation of accepting those defect values [Citation42,Citation43]. In this simulation, a single energy distribution with standard lighting of 100 mW/cm2 (T = 300 K (K) or 27°C) has been used to provide the illumination (Figure ).
Figure 5. Schematic devise structure of FTO/CuAlO2/CH3NH3SnI3/WO3/Au solar cell with band alignment.

Table 1. Summarized data for simulation.
4.1. Band diagram
The photocurrent is caused by an excessive amount of minority carriers in the absorber layer. The process of drift and diffusion regulates the transport of holes and electrons from the absorber to the HTL and ETL. A mismatch in the lattice between two interfaces leads to the creation of interface defects, which in turn cause carrier recombination [Citation46]. Cliff and spike are the two types of band structures that may be found at the interface. The principal recombination process takes place at the interface when the band structures reveal cliff-like forms. Figure shows that a spike is observed in both interfaces, leading to a decrease in carrier recombination. The bending in the conduction band is due to the existence of a cliff structure at the interface. Band offset at the pn-junction must be positive (spike-like) in order to attain the optimal device performance [Citation47]. However, negative band offset with suitable band alignment can show better performance. This is attributable to the fact that the activation energy of recombination (Ea) at the absorber layer plays an important role in device performance [Citation48]. When the Ea value is increased, the degree of interface recombination diminishes. This band offset may reduce the amount of charge recombination by preventing injected electrons/holes from reaching the interface [Citation49]. The band gap (Eg) of the absorber layer was considered 1.3 eV. The conduction band offset (CBO) of −0.43 eV (χAbs − χETL) [Citation27] creates a cliff type barrier [Citation27,Citation50] that facilitates electron passage from the perovskite layer to the ETL, thereby preventing recombination. Similarly, the valence band offset (VBO) of −0.53 eV ((χAbs + Eg,Abs) − (χHTL + Eg,HTL)) [Citation25] also establishes a cliff-type barrier at the valence band edge, enabling holes to travel from the perovskite layer to the HTL, thereby mitigating excessive recombination. This barrier at the interfaces reduces the probability of carriers returning to the region where they originated and decreases the chance of recombination. While a larger band offset might typically be associated with increased carrier recombination, in this case, the alignment of energy levels is more favourable for charge transport, enhancing the overall device performance. This alignment could improve charge extraction, thereby reducing recombination rates and leading to better device efficiency.
4.2. Thickness optimization of HTL, ETL, and the Absorber layer
This section contains mainly simulated investigation on perovskite absorber layer thickness and their corresponding photovoltaic performances deploying WO3 (ETL) and CuAlO2 (HTL) as charge transporting layers. Besides, the effects of ETL and HTL thickness on the cell efficiency are also mentioned here. Maintaining other factors constant, CH3NH3SnI3 film thickness is scaled from 100 to 1000 nm, and photovoltaic outputs are shown in Figure . The variance of active layer thickness shows a very small influence on Voc (Figure (a)), but the Jsc (Figure (b)) has been intensely influenced since the Jsc reached 35.00 mAcm−2 from 15.00 mAcm−2 because of increased thickness. The reduction of Voc outside of 200 nm happens as recombination results from sluggish carrier movement. Positively, the thicker active layer is responsible for carrier generation as well as related recombination governing carrier lifetimes. Therefore, Jsc increases; as additional photons are captivated by the absorber layer when it is thicker. The promotion of photocarriers increased Jsc. An augmented Voc is apparent with cumulative depth till 300 nm. Outside these widths, Voc twitches lessen. The downshifting of Voc in respect to width because of incremental Jo is referred to as dark saturation current that is liable for higher carrier recombination. The reliance of Voc on Jsc and Jo follows as [Citation51].
(2)
(2) where K and T denote Boltzmann constant, temperature (Kelvin), correspondingly. Based on Equation (2), a short Jo is obligatory to attain greater Voc.
Figure 7. Effect of absorber layer thickness on cell performance, (a) Voc, Jsc vs thickness; (b), PCE, FF vs thickness; (c), QE (%) as a function of absorber thickness; (d) PCE for ETL, HTL and Without HTL Vs Thickness.

The FF outcomes in an unceasing decrement (Figure (b)) with cumulative enhanced thickness. Recombination incidences are considered for the typical model. The advanced PCE might be recognized through robust photon engagement along with long diffusion length (Ld) [Citation52]. Absorber thickness of a few hundred nm is satisfactory to engross supreme incident sunlight. All photogenerated carriers ought to gather, which are generated at minor spaces rather than scattering distance from CH3NH3SnI3/WO3 and CuAlO2/CH3NH3SnI3 interfaces. Variation of quantum efficiency (QE) graph for different values of absorber layer thickness is shown in Figure (c) representing that the thicker absorber layer confirms higher values of QE. Figure (d) shows the influence of ETL and HTL (with or without) thickness on the PCE of the PSC. The width difference of ETL, HTL, and HTL-free PSCs has been examined from 50 to 150 nm. The PCE remains nearly unaltered for thickness variation of ETL and HTL. The PCE decreases very insignificant values, specifically for higher HTL thickness. It is highly essential to have a decent pact among simulations and tentative studies to authenticate the correctness and applied understanding of PSC.
Therefore, it is expected that projected PV factors of the proposed structure would continue inside scope when leading to actual investigational demos. The presented performance of HTL-free PSC has to be examined by opposing HTL width in the same range. A PSC device without HTL displays the maximum 3% PCE at any thickness value smaller than that of a PSC with HTL. Therefore, it could be remarked that ETL and HTL are obligatory in device structure to attain high performance.
4.3. Study of the effect of carrier density of HTL (NA)
The simulations are performed at different NA in HTL to observe the doping influence. The NA concentration varied from 1 × 1014 cm−3 to 1 × 1020 cm−3. The effect of NA of CuAlO2 on Jsc and Voc of SC is presented in Figure .
The Jsc and Voc tend to increase at a very insignificant value and decline, as seen in Figure (a). The highest and the lowest Jsc are observed at 33.44 mA cm−2 and 33.42 mA cm−2, individually. After an NA value of 1 × 1017 cm−3, Jsc remains to drop by a small amount. Voc displays an inspiring stance of 0.966 V. Figure (b) indicates the gained PCE and FF. At lower NA (1 × 1018 cm−3) agreements, the highest FF and highest PCE are 78.1% and 25.25%, respectively. PCE increases by NA mainly due to Voc. Amplified doping stages at HTL increase the interfacial electric field, requiring distinct excitons after amplified device presentation [Citation53].
4.4. Study of the effect of interface defect density
Recombination at edges can be condensed to raise PSC output [Citation54]. To elude shunting loss, numerous approaches for interface modelling have lately been engaged keenly in attention [Citation55]. Figure embodies the impacts of boundary defects on PSC output, where defect concentrations are diverse from 1014 cm−3 to 1020 cm−3. The effect of defect properties at CuAlO2-MASnI3 (IL1) and MASnI3-WO3 (IL2) were considered to study. Figure (a) proves that Voc and Jsc for IL1 are almost constant till 1016 cm−3 and together decline by the additional upsurge of defect densities towards 1020 cm−3. Likewise, Figure (b) specifies no quantifiable variations in FF, though some insignificant hills and valleys appear from 1016 cm−3 to 1020 cm−3. FF suits the highest at defect density of 1018 cm−3 in IL1. The changes in series resistance due to increasing defect densities in PSC might be the reason for such variation [Citation56]. But PCE remains constant till 1016 cm−3, and PCE subsequently drops from 25.3% to 24.45% for further rises of the defect. The key reason for PCE decrease is the lessening of both Voc and Jsc after 1016 cm−3 in IL1 to some extent. Figure (c) displays that there are no variations in Jsc for defects in IL2. However, Voc slowly declines nearly by 30% with higher interface defect density. Figure (d) displays that FF is intensely enriched with a cumulative defect in IL2 till 1017 cm−3. The association between FF and collective IL2 defect densities could be clarified through ideality factor (n) responsible for the charge carrier transportation pathways from creation to the load. This marvel is related to semiconductor characteristics. The n straight affects the FF of PSC [Citation57]. The rise in FF is produced by lessening defects in IL2 powerfully distressing the PCE from 25.2% to 17.8%.
4.5. Study of the effect of series and shunt resistance on FF and PCE
Series and shunt resistance affect PV device performances significantly. The total resistance between terminals & the cell's right and rear contacts is series resistance. Series resistance is internal resistance, whereas shunt resistance is due to the reverse saturation current of the active junction [Citation58]. A portion of the device's total electrical resistance is contributed by the back contact, HTL, perovskite layer, ETL, and front contact (FTO). In order to investigate how the series and shunt resistance influences the performance of PSCs, we simulate the performance by changing the series resistance from 0 to 5 Ω cm2 and shunt resistance from 1000 to 5000 Ω cm2. The higher the series resistance, the greater the power losses. The power lost through the PSC can be expressed as Equation (3),
(3)
(3) Figure shows the impact of series and shunt resistance on the device's performance. Both series and shunt resistance greatly impact FF. FF is decreased from 78% to 64% and PCE is decreased from 25.5% to 20.5% with the increase of series resistance from 0 to 5 Ω cm2 (Figure (a)). Shunt paths deflect the current away from the load that was allocated for it, and as a result, they have a detrimental effect on the performance of the module, particularly at lower illumination levels. High-quality cells have greater shunt resistance and lower shunt current. Shunt current may cause cell heating in modules, harming the encapsulating layer. Shunt current is usually caused by defects in solar cells or cell interconnection problems. Ideal shunt resistance is considered to be infinite [Citation59]. In this simulation, FF is increased from 76.2% to 77.7% and PCE is increased from 24.6% to 25.13% with the increment of shunt resistance from 1000 to 5000 Ω cm2 (Figure (b)).
4.6. Study of the effect of temperature on cell performances
The stability of the device depends on the materials used in the cell, their properties (chemical inertness, thermal stability, non-hygroscopicity, etc.), defect-free manufacturing, encapsulation, etc. The absorber material (CH3NH3SnI3) used in our proposed device has outstanding temperature stability and a high defect tolerance limit. There are some issues with the chemical stability of this material because it tends to oxidize in an open environment. Adding anti-oxidant and encapsulation to the device can solve the problem. Chemically inert inorganic metal oxides WO3 and CuAlO2 are more stable than most organic ETLs or HTLs. Temperature stability was simulated from 300 to 400 K, and it was found that PCE is lowered only by 4.3% (Figure (b)) due to the lower trend of Voc (Figure (a)) and FF (Figure (b)) at a higher temperature. Temperature dependent Voc can be written as Equations (4) and (5) [Citation22];
(4)
(4)
(5)
(5) where Eg,0 is the band gap at T = 0 K, and a and b are constants. So, the bandgap decreases with increasing temperature, which impacts the Voc of the cell. With increasing temperature, Jsc remained constant. The series resistance of the solar cell is increased by the thermal scattering of electrons at high temperatures that lower the cell FF. Performance as a whole declined from a PCE of 25.3% to 19.8% with the increment of temperature 300 K to 400 K.
4.7. Mott–Schottky capacitance analysis
Temperature-dependent capacitance is seen in Figure . Low bias (< 0.4 V) does not affect capacitance, whereas high bias (> 0.4 V) does (Figure (a)). Temperature influences the dielectric characteristics of materials, increasing capacitance. Mott–Schottky investigation of temperature can be given in the following Equation (6) [Citation60],
(6)
(6) Capacitance, surface area, and space permittivity are denoted by C, S, and ϵ, respectively, while kB and T have their customary meanings, NA stands for acceptor volume, Vbi indicates flat band potential, and q stands for an elementary charge. Temperature-dependent C-V and Mot-Schottky graphs are shown in Figure (b). At the donor–acceptor interface, where Vbi is independent of Voc, recombination takes place. When Vbi goes below Voc, FF decreases. Tangent intersecting at the voltage axis in the Mot-Schottky graphs yields the Vbi. As T rises from 300 K to 400 K, the Vbi decreases from 0.78 V to 0.69 V, indicating greater rates of carrier recombination and an increase in series resistance with temperature. The proposed structure can maintain 84% of its efficiency at higher temperatures because of the high Vbi value.
4.8. Study on the effect of the interfacial buffer layer
Figure represents the impact of interfacial layer on device performances. Typically, PSC consists of four interfaces; for regular structure devices, these are TCO/ETL, ETL/perovskite, perovskite/HTL, and HTL/metal, and device for inverted structure; TCO/HTL, HTL/perovskite, perovskite/ETL, and ETL/metal. However, the two interfaces, ETL/perovskite, and HTL/perovskite, are the same for both devices except for the interfaces of TCO and metal electrodes [Citation61]. These two common interfaces play a very effective role in transporting photogenerated charge from the perovskite layer to the electrode through respective CTL. Several factors are involved in the interfaces to reduce device performance, such as state of defect density, band alignment, the position of band edges, etc. [Citation62]. In this study, IL1 (HTL/perovskite) does not have such a remarkable impact on device outcomes, but defects in IL2 (perovskite/ETL) have significant impacts that reduced the PCE by 32%, as indicated in Figure (d). The position of the conduction band edge of perovskite is at −4.11 eV, while that of ETL is at −4.38 eV. This energy gap could be one of the reasons for the efficiency losses [Citation63]. To compensate for the losses, an interfacial buffer layer (PCBM with the thickness of 20 nm) has been incorporated into the device structure and simulated, which enabled the regain of the PCE by 5.8%. Figure shows the J-V curve for presenting the impact of the interfacial buffer layer on device performances. The PCE of the modified device is 26.74%, and corresponding Voc, Jsc, and FF are 0.99 V, 33.43 mA cm−2, and 81.05%, respectively.
4.9. Comparative study
The simulation study has also been extended for some other prominent inorganic and organic HTMs in a similar device. The HTMs are NiO, CuI, Cu2O, CuSCN, and PEDOT: PSS and their corresponding J-V curves are presented in Figure . All devices produce almost the same Jsc (33.15–33.44 mA cm−2), exceptional for organic HTM, PEDOT: PSS (31.26 mA cm−2) is the lowest one. The NiO-based device gives the lowest PCE at 18.64% due to its low FF (65.64%) compared to others. Cu2O is a simple, promising semiconducting oxide that provides the 2nd highest PCE 24.23%, with Voc 0.96 V, Jsc 33.15 mA cm−2, and FF 76.20%. In this study, among all HTMs, the CuAlO2-based device shows the highest PCE 25.29% without a buffer layer, and with a buffer layer, it is 26.74%. A comparative results are presented in Table , in which the best simulation results from this study considering CuAlO2 as HTM are compared with the other studies found from different literature.
Table 2. A comparison between this work and a related study published.
5. Conclusion
In the 1st part of the study, p-type CuAlO2 has been prepared by the green synthesis process and characterized by XRD, FTIR, and UV-Vis spectroscopy. The spectroscopic data are used to calculate the bandgap (Eg = 3.5 eV) of delafossite CuAlO2 material by the Tauc plot method. In the 2nd part, a SCAPS-1D simulation study has been carried out on Pb-free perovskite solar cells using synthesized CuAlO2 as HTL and WO3 as ETL. In this simulation, experimentally found Eg of CuAlO2 was used, which matches with the previously used value in different studies, confirming the feasible use of green synthesized CuAlO2 as a proper HTL in p-i-n structured PSC. Besides, this simulation confirmed the proper matching of CuAlO2 as HTL in a lead-free perovskite-based solar cell. To the best of our knowledge, use of green synthesized CuAlO2 as HTL in a eco-friendly PSC development was not analysed theoretically before. Furthermore, the device performances have been optimized with changing layer thickness, internal and interface defects, carrier density, shunt, and series resistance. The effects of temperature and buffer layer have also been evaluated. The performance of CuAlO2 as HTL in the proposed device has been compared to a similar structured device with different HTLs such as NiO, Cu2O, CuI, CuCNS, and PEDOT: PSS. The investigation represents that the CuAlO2-based optimized device exhibits the highest PCE 26.74%, with relative Voc, Jsc, and FF of 0.99 V, 33.43 mA cm−2, and 81.05%, respectively. The results of the overall study identified in-depth insights into internal factors that encourage the fabrication of the real device.
Acknowledgements
This work is funded by the Deputyship of Research & Innovation, Ministry of Education in Saudi Arabia, through project number(959/1443). In addition, the authors would like to express their appreciation for the support provided by the Islamic University of Madinah. The authors would also like to express their gratitude for the financial assistance provided by the Dana Impact Research Award from the University Kebangsaan Malaysia (DIP 2021-026). Finally, the authors are grateful to Dr Marc Burgelman of the University of Gent in Belgium for supplying the SCAPS-1D simulation programme.
Disclosure statement
No potential conflict of interest was reported by the author(s).
References
- Hossain MK, Ishraque Toki GF, Samajdar DP, et al. Deep insights into the coupled optoelectronic and photovoltaic analysis of lead-free CsSnI3 perovskite-based solar cell using DFT calculations and SCAPS-1D simulations. ACS Omega. 2023;8(25):22466–22485.
- Hossain MK, Bhattarai S, Arnab AA, et al. Harnessing the potential of CsPbBr3-based perovskite solar cells using efficient charge transport materials and global optimization. RSC Adv. 2023;13(30):21044–21062. doi:10.1039/D3RA02485G
- Hossain MK, Toki GFI, Kuddus A, et al. An extensive study on multiple ETL and HTL layers to design and simulation of high-performance lead-free CsSnCl3-based perovskite solar cells. Sci Rep. 2023;13(1):2521. doi:10.1038/s41598-023-28506-2
- Hossain MK, Toki GFI, Kuddus A, et al. Optimization of the architecture of lead-free CsSnCl3-perovskite solar cells for enhancement of efficiency: a combination of SCAPS-1D and wxAMPS study. Mater Chem Phys. 2023;308:128281. doi:10.1016/j.matchemphys.2023.128281
- Haque MM, Mahjabin S, Khan S, et al. Study on the interface defects of eco-friendly perovskite solar cells. Sol Energy. 2022;247:96–108. doi:10.1016/j.solener.2022.10.024
- Ahmed S, Akhtaruzzaman M, Zulhafizhazuan W, et al. Theoretical verification of using the Ga-doped ZnO as a charge transport layer in an inorganic perovskite solar cell. Jpn J Appl Phys. 2023;62(9):0092001. doi:10.35848/1347-4065/aced74
- Das B, Liu Z, Aguilera I, et al. Defect tolerant device geometries for lead-halide perovskites. Mater Adv. 2021;2(11):3655–3670. doi:10.1039/D0MA00902D
- Mahmud Hasan AK, Raifuku I, Amin N, et al. Air-stable perovskite photovoltaic cells with low temperature deposited NiOx as an efficient hole-transporting material. Opt Mater Express. 2020;10(8):1801–1816. doi:10.1364/OME.391321
- Cao Q, Li Y, Zhang H, et al. Efficient and stable inverted perovskite solar cells with very high fill factors via incorporation of star-shaped polymer. Sci Adv. 2021;7(28):eabg0633.
- Min H, Lee DY, Kim J, et al. Perovskite solar cells with atomically coherent interlayers on SnO2 electrodes. Nature. 2021;598(7881):444–450. doi:10.1038/s41586-021-03964-8
- Islam MA, Sarkar DK, Shahinuzzaman M, et al. Green synthesis of lead sulphide nanoparticles for high-efficiency perovskite solar cell applications. Nanomaterials. 2022;12(11):1933. Available from: https://www.mdpi.com/2079-4991/12/11/1933
- Singh NK, Agarwal A. Numerical investigation of electron/hole transport layer for enhancement of ecofriendly Tin-Ge based perovskite solar cell. Energy Sources Part A. 2023;45(1):3087–3106. doi:10.1080/15567036.2023.2192182
- Wan Z, Lai H, Ren S, et al. Interfacial engineering in lead-free tin-based perovskite solar cells. J Energy Chem. 2021;57:147–168. doi:10.1016/j.jechem.2020.08.053
- Cao J, Yan F. Recent progress in tin-based perovskite solar cells. Energy Environ Sci. 2021;14(3):1286–1325. doi:10.1039/D0EE04007J
- Wang M, Wang W, Ma B, et al. Lead-Free perovskite materials for solar cells. Nano-Micro Lett. 2021;13(1):62. doi:10.1007/s40820-020-00578-z
- Nishimura K, Kamarudin MA, Hirotani D, et al. Lead-free tin-halide perovskite solar cells with 13% efficiency. Nano Energy. 2020;74:104858. doi:10.1016/j.nanoen.2020.104858
- Jiang X, Wang F, Wei Q, et al. Ultra-high open-circuit voltage of tin perovskite solar cells via an electron transporting layer design. Nat Commun. 2020;11(1):1–7. doi:10.1038/s41467-019-13993-7
- Mottakin M, Sobayel K, Sarkar D, et al. Design and modelling of eco-friendly CH3NH3SnI3-based perovskite solar cells with suitable transport layers. Energies. 2021;14(21):7200. doi:10.3390/en14217200
- Singh NK, Agarwal A. Performance assessment of sustainable highly efficient CsSn0.5Ge0.5I3/FASnI3 based Perovskite solar cell: a numerical modelling approach. Opt Mater (Amst). 2023;139:113822. doi:10.1016/j.optmat.2023.113822
- Li S, Cao Y-L, Li W-H, et al. A brief review of hole transporting materials commonly used in perovskite solar cells. Rare Met. 2021;40(10):2712–2729. doi:10.1007/s12598-020-01691-z
- Dunlap-Shohl WA, Daunis TB, Wang X, et al. Room-temperature fabrication of a delafossite CuCrO2hole transport layer for perovskite solar cells. J Mater Chem A. 2018;6(2):469–477. doi:10.1039/C7TA09494A
- Sarkar DK, Mahmud Hasan AK, Mottakin M, et al. Lead free efficient perovskite solar cell device optimization and defect study using Mg doped CuCrO2 as HTL and WO3 as ETL. Sol Energy. 2022;243:215–224. doi:https://doi.org/10.1016/j.solener.2022.07.013.
- Kawazoe H, Yasukawa M, Hyodo H, et al. P-type electrical conduction in transparent thin films of CuAlO2. Nature. 1997;389:939–942. doi:10.1038/40087
- Igbari F, Li M, Hu Y, et al. A room-temperature CuAlO2hole interfacial layer for efficient and stable planar perovskite solar cells. J Mater Chem A. 2016;4(4):1326–1335. doi:10.1039/C5TA07957H
- Shasti M, Mortezaali A. Numerical study of Cu2O, SrCu2O2, and CuAlO2 as hole-transport materials for application in perovskite solar cells. Physica Status Solidi A. 2019;216(18):1900337.
- Bao F, Sui H, Gong Z, et al. Enhanced interstitial oxygen-enabled efficient CuAl(M)O2hole extractors for air-stable all-inorganic perovskite solar cells. ACS Sustain Chem Eng. 2023;11(14):5665–5673. doi:10.1021/acssuschemeng.3c00149
- Jan ST, Noman M. Analyzing the effect of planar and inverted structure architecture on the properties of MAGeI3perovskite solar cells. Energy Technol. 2023;11:2300564. doi:10.1002/ente.202300564
- Mahjabin S, et al. Boosting perovskite solar cell stability through a sputtered Mo-doped tungsten oxide (WOx). Electron Transport Layer. Energy Fuels. 2023;37(24):19860–19869.
- Mahjabin S, Haque M, Sobayel K, et al. Investigation of morphological, optical, and dielectric properties of RF sputtered WOx thin films for optoelectronic applications. Nanomaterials. 2022;12(19):3467. doi:10.3390/nano12193467
- Mahjabin S, Hossain MI, Haque MM, et al. Sputtered WOx thin film as the electron transport layer for efficient perovskite solar cells. Appl Phys A. 2022;128(4):358. doi:10.1007/s00339-022-05500-5
- Mahjabin S, Mahfuzul Haque M, Khan S, et al. Effects of oxygen concentration variation on the structural and optical properties of reactive sputtered WOx thin film. Sol Energy. 2021;222:202–211. doi:10.1016/j.solener.2021.05.031
- Mahy JG, Lambert SD.. Heterogeneous photocatalysis: a solution for a greener earth. Catalysts. 2022;12:686.
- Nakanishi A, Katayama-Yoshida H, Ishikawa T, et al. Chemical trend of superconducting critical temperatures in hole-doped CuBO2, CuAlO2, CuGaO2, and CuInO2. J Phys Soc Jpn. 2016;85(9):0094711. doi:10.7566/JPSJ.85.094711
- Kawazoe H, Yasukawa M, Hyodo H, et al. P-type electrical conduction in transparent thin films of CuAlO2. Nature. 1997;389(6654):939–942. doi:10.1038/40087
- Benreguia N, Barnabé A, Trari M. Sol–gel synthesis and characterization of the delafossite CuAlO2. J Solgel Sci Technol. 2015;75(3):670–679. doi:10.1007/s10971-015-3737-x
- Saha B, Thapa R, Chattopadhyay K. A novel route for the low temperature synthesis of p-type transparent semiconducting CuAlO2. Mater Lett. 2009;63(3-4):394–396. doi:10.1016/j.matlet.2008.10.048
- Ferraro JR.. Infrared spectra of inorganic and coordination compounds (Nakamoto, Kazuo). J. Chem. Educ. 1963;40(9):501.
- Prakash T, Padma Prasad K, Ramasamy S, et al. Optical and electrical properties of mechanochemically synthesized nanocrystalline delafossite CuAlO2. J Nanosci Nanotechnol. 2008;8(8):4273–4278. doi:10.1166/jnn.2008.AN23
- Abnavi H, Maram DK, Abnavi A. Performance analysis of several electron/hole transport layers in thin film MAPbI3-based perovskite solar cells: a simulation study. Opt Mater (Amst). 2021;118:111258. doi:10.1016/j.optmat.2021.111258
- Alkhammash HI, Haque M. Device modelling and performance analysis of chalcogenide perovskite-based solar cell with diverse hole transport materials and back contact metals. Jpn J Appl Phys. 2023;62(1):0012006. doi:10.35848/1347-4065/acb11b
- Shin SS, Lee SJ, Seok SI. Exploring wide bandgap metal oxides for perovskite solar cells. Apl Mater. 2019;7(2):022401. doi:10.1063/1.5055607.
- Patel M, Ray A. Enhancement of output performance of Cu2ZnSnS4 thin film solar cells—a numerical simulation approach and comparison to experiments. Phys B. 2012;407(21):4391–4397. doi:10.1016/j.physb.2012.07.042
- Karthick S, Velumani S, Bouclé J. Experimental and SCAPS simulated formamidinium perovskite solar cells: a comparison of device performance. Sol Energy. 2020;205:349–357. doi:10.1016/j.solener.2020.05.041
- Shasti M, Mortezaali A. Numerical study of Cu2O, SrCu2O2, and CuAlO2 as hole-transport materials for application in Perovskite solar cells. Physica Status Solidi A. 2019;216(18):1900337. doi:https://doi.org/10.1002/pssa.201900337.
- Hao F, Stoumpos CC, Cao DH, et al. Lead-free solid-state organic–inorganic halide perovskite solar cells. Nat Photonics. 2014;8(6):489–494. doi:10.1038/nphoton.2014.82
- Kumar M, Askari SA, Ram SK, et al. Investigation of all-oxide thin-film solar cell with p-SnOx as absorber layer. IEEE Trans Electron Devices. 2022;69(3):1115–1122. doi:10.1109/TED.2022.3143077
- Ghorbani E. On efficiency of earth-abundant chalcogenide photovoltaic materials buffered with CdS: the limiting effect of band alignment. J Physics Energy. 2020;2(2):0025002. doi:10.1088/2515-7655/ab6942
- Yu X, Zou X, Cheng J, et al. Numerical simulation analysis of effect of energy band alignment and functional layer thickness on the performance for perovskite solar cells with Cd1-xZnxS electron transport layer. Mater Res Express. 2020;7(10):105906. doi:10.1088/2053-1591/abbf12
- Ding C, Zhang Y, Liu F, et al. Effect of the conduction band offset on interfacial recombination behavior of the planar perovskite solar cells. Nano Energy. 2018;53:17–26. doi:10.1016/j.nanoen.2018.08.031
- Khattak YH, Baig F, Toura H, et al. CZTSe kesterite as an alternative hole transport layer for MASnI3 perovskite solar cells. J Electron Mater. 2019;48(9):5723–5733. doi:10.1007/s11664-019-07374-5
- Katz EA, Faiman D, Tuladhar SM, et al. Temperature dependence for the photovoltaic device parameters of polymer-fullerene solar cells under operating conditions. J Appl Phys. 2001;90(10):5343–5350. doi:10.1063/1.1412270
- Bai Z, Chen S-C, Lin S-S, et al. Review in optoelectronic properties of p-type CuCrO2 transparent conductive films. Surf Interfaces. 2021;22:100824. doi:10.1016/j.surfin.2020.100824
- Kharangarh P, Misra D, Georgiou G, et al. Characterization of space charge layer deep defects in n+-CdS/p-CdTe solar cells by temperature dependent capacitance spectroscopy. J Appl Phys. 2013;113(14):144504. doi:10.1063/1.4800830
- Wolff CM, Caprioglio P, Stolterfoht M, et al. Nonradiative recombination in perovskite solar cells, 2019.
- Ghobadi A. Effect of interface defects on high efficient perovskite solar cells.
- Zhou X, Han J. Design and simulation of C2N based solar cell by SCAPS-1D software. Mater Res Express. 2020;7(12):126303. doi:10.1088/2053-1591/abcdd6
- Vasić A, Stojanović M, Osmokrović P, et al. The influence of ideality factor on fill factor and efficiency of solar cells. Mater Sci Forum. 2000;352:241–246. doi:10.4028/www.scientific.net/MSF.352.241
- Jayan KD, Sebastian V, Kurian J. Simulation and optimization studies on CsPbI3 based inorganic perovskite solar cells. Sol Energy. 2021;221:99–108. doi:10.1016/j.solener.2021.04.030
- Dhass AD, Natarajan E, Ponnusamy L. Influence of shunt resistance on the performance of solar photovoltaic cell. 2012 International Conference on Emerging Trends in Electrical Engineering and Energy Management (ICETEEEM); 2012 Dec 13–15. p. 382–386. doi:10.1109/ICETEEEM.2012.6494522
- Gelderman K, Lee L, Donne SW. Flat-band potential of a semiconductor: using the Mott–Schottky equation. J Chem Educ. 2007;84(4):685. doi:10.1021/ed084p685
- Cho A-N, Park N-G. Impact of interfacial layers in perovskite solar cells. ChemSusChem. 2017;10(19):3687–3704. doi:10.1002/cssc.201701095
- Zhang F, Ma W, Guo H, et al. Interfacial oxygen vacancies as a potential cause of hysteresis in perovskite solar cells. Chem Mater. 2016;28(3):802–812. doi:10.1021/acs.chemmater.5b04019
- Meng F, Liu K, Dai S, et al. A perylene diimide based polymer: a dual function interfacial material for efficient perovskite solar cells. Mater Chem Front. 2017;1(6):1079–1086. doi:10.1039/C6QM00309E
- Ismail M, Noman M, Tariq Jan S, et al. Boosting efficiency of eco-friendly perovskite solar cell through optimization of novel charge transport layers. R Soc Open Sci. 2023;10(6):230331. doi:10.1098/rsos.230331
- Savva A, Papadas IT, Tsikritzis D, et al. Inverted perovskite photovoltaics using flame spray pyrolysis solution based CuAlO2/Cu–O hole-selective contact. ACS Appl Energy Mater. 2019;2(3):2276–2287. doi:10.1021/acsaem.9b00070
- Igbari F, Li M, Hu Y, et al. A room-temperature CuAlO2hole interfacial layer for efficient and stable planar perovskite solar cells. J Mater Chem A. 2016;4(4):1326–1335. doi:10.1039/C5TA07957H