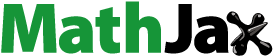
Abstract
This study investigated the radiation shielding performance of the xBaO-(30-x)TeO2-35Bi2O3-35B2O3 glass system, where x = 5, 10, 15, 20 and 25, using Phy-X/PSD software and MCNPX Monte Carlo code. Key radiation shielding indicators, including attenuation coefficients, half value layer (HVL), tenth value layer (TVL), effective atomic number (Zeff), mean free path (MFP), and build-up factors, were measured within the energy range of 0.015–15 MeV. Further, the study assessed the glasses' capacity to block fast neutrons, using the fast neutron removal cross-section (ΣR) as a metric. The results showed that gamma shielding effectiveness follows the order: BaTBiB-1 < BaTBiB-2 < BaTBiB-3 < BaTBiB-4 < BaTBiB-5 at all photon energies. Additionally, the ΣR values for BaTBiB-1 to BaTBiB-5 glasses were 0.10179, 0.10263, 0.10362, 0.10581, and 0.10623 cm−1, respectively. Therefore, the findings demonstrate that including BaO enhances the gamma and neutron shielding characteristics of tellurite glasses, making them valuable for radiation-related applications.
1. Introduction
The spectrum of ionizing radiation includes a diversity of emissions, such as charged particles, neutrons, and gamma/X-rays, each emanating from a variety of natural and anthropogenic sources and bearing an array of energies with varying levels of associated risks [Citation1, Citation2]. Specifically, gamma radiation, which is distinguished by its lack of charge and mass, carries high-energy photons capable of traversing extended distances, thereby granting it the penetrative power to bypass various materials [Citation3]. This form of radiation originates from both ubiquitous natural sources, including cosmic rays, radiation from within the human body, and the Earth’s crust, as well as from synthetic origins, such as medical imaging technologies, nuclear weaponry, and energy production in nuclear facilities [Citation4–6]. Ionizing radiation can be utilized in various fields, including medical applications, industry, and research. For example, medical applications rely on a considerable volume of X and gamma-ray machines daily, while industries employ radiation for purposes like crack detection, irradiation, and security screening [Citation7–9]. Gamma radiation exposure can be harmful to living organisms, as it has the potential to cause severe damage to biological tissues and DNA. This damage can result in genetic mutations, an elevated risk of cancer, and adverse implications for both the health of individuals and the ecological system [Citation10, Citation11]. It’s worth mentioning that the implementation of effective shielding materials is crucial in mitigating the detrimental impact of gamma radiation. Therefore, to mitigate these detrimental effects and ensure the safety of the surrounding environment and humans, the use of radiation shielding materials is highly recommended.
Lead (Pb), while traditionally used as a radiation shielding material, is gradually being phased out due to significant concerns over its weight, toxicity, and environmental impact. Therefore, in the pursuit of Pb-free alternatives, various materials like polymers, concrete, ceramics, and alloys have been developed and suggested as effective radiation shielding options [Citation12–14]. While these materials offer acceptable radiation protection efficiency and are cost-effectiveness, they have various drawbacks. These drawbacks include the formation of cracks, water permeability, and the inability to transmit the visible light [Citation15]. Fortunately, glasses stand out as potential and competitive alternatives due to their outstanding characteristics, including inherent density, high optical clarity, recyclability, and versatility in shape and size [Citation16–19]. As a result, they have found applications in various fields such as electronics and optics [Citation20, Citation21]. Tellurium oxide (TeO2) has attracted considerable interest among oxide glasses, including phosphate, borate, and silicate glasses, owing to its remarkable physical and chemical characteristics. These glasses exhibit a high thermal expansion coefficient, a high index of refraction, a low glass transition temperature, a wide range of dielectric constants, nonlinear optical properties, a low melting temperature, and superior optical transmission in the infrared region [Citation22–24]. Consequently, TeO2 has been extensively employed in numerous photonic applications [Citation25–27]. The addition of glass modifiers such as ZnO and BaO can improve the TeO2 glass matrix and address certain limitations, such as reducing the manufacturing temperature and preventing glass clustering and crystallization [Citation28–31]. Furthermore, the incorporation of BaO into TeO2 glasses has been identified to enhance their capabilities in shielding against radiation. In a previous study [Citation32], a series of TeO2-Li2O-BaO glasses with varying BaO concentrations were fabricated and tested for their optical, structural, and radiation shielding characteristics. Accordingly, the increasing incorporation of BaO led to a reduction in the optical bandgap, while simultaneously improving both the glass transition temperature (Tg) and the abilities of radiation shielding. Another study conducted by Boonin et al. [Citation30] examined the impact of BaO content on radiation shielding in glassy materials composed of TeO–ZnO–BaO. The investigation encompassed experimental and simulation approaches, specifically analysing gamma ray energies within the range of 223.02–662 keV. The research determined that glass with a composition of 25 mol% BaO demonstrated optimal effectiveness for gamma shielding purposes. Moreover, an investigation conducted by Srinivas et al. [Citation33] delved into the shielding properties of the BaO-TeO2-B2O3-Cr2O3 glass system. Their findings highlighted that the radiation shielding behaviour exhibits enhancements with the incorporation of larger quantities of barium oxide. The impact of BaO was further explored in a distinct glass system (SiO2–B2O3–BaO–CaO–ZnO–MgO), revealing its noteworthy contribution to enhanced shielding characteristics [Citation34]. In addition, Vani et al. [Citation35] also observed a similar positive effect of BaO on the radiation properties of fluorotellurite glasses, further validating the beneficial influence of BaO as a modifier in enhancing the radiation shielding capabilities of glass systems. Given the significance of tellurite glasses in the realm of radiation shielding, the primary goal of this study is to evaluate their efficacy in attenuating both gamma and neutron radiation. The evaluation covers a broad spectrum of gamma-ray energies, ranging from 0.015 to 15 MeV, utilizing the Phy-X/PSD software [Citation36]. Furthermore, the study involves corroborating the mass attenuation coefficients of the evaluated samples through the MCNPX Monte Carlo simulation code. The novelty of this study lies in its investigation of the previously unexplored correlation between the incorporation of BaO at different concentrations (5, 10, 15, 20, and 25 mol%) and the consequent shielding capabilities against gamma ray and neutron particles in tellurite glass systems. Consequently, this research not only expands the potential applications of these glasses but also provides valuable insights for the effective utilization of tellurite glasses in radiation shielding, particularly within medical, industrial, and research settings.
2. Materials and methods
2.1. Glass characterization
This study’s main aim was to analyse the shielding capabilities against gamma and neutron radiation of previously prepared tellurite glass samples using the melt quenching technique, as described by [Citation37]. These samples, designated as xBaO-(30-x)TeO2-35Bi2O3-35B2O3, have varied compositions, ranging from 5 to 25 mol % BaO. They are identified as BaTBiB-1 (5.73 g/cm3), BaTBiB-2 (5.81 g/cm3), BaTBiB-3 (5.90 g/cm3), BaTBiB-4 (6.06 g/cm3), and BaTBiB-5 (6.12 g/cm3). Table presents these samples, detailing their elemental mole fractions, densities, and specific codes. Figure illustrates the density variations in these glass samples in relation to the increasing BaO content. The observations revealed that as the concentration of BaO increased, there was a noticeable augmentation in the mass and elemental composition of the glasses, consequently resulting in a significant rise in their densities. This led to a substantial density disparity of 0.39 g/cm3 between the samples with the lowest and highest levels of BaO enhancement.
Figure 1. The relationship between the densities of glass (g/cm³) and the increasing contribution of BaO (%mole) in its chemical structure.

Table 1. Samples code, elemental mole fraction and density of xBaO-(30-x)TeO2-35Bi2O3-35B2O3 glasses.
2.2. Theoretical basis
As gamma photons pass through a medium, they undergo various interactions, most notably the photoelectric effect and Compton scattering. In the photoelectric effect, the medium’s atoms completely absorb the photons. On the other hand, Compton scattering leads to a decrease in photon energy because of interactions with electrons [Citation38]. Shielding materials, crucial for reducing ionizing radiation risks, are designed to efficiently absorb both scattered and propagated photons. These materials are essential in limiting undesired human interaction with gamma rays. The evaluation of a material’s ability to attenuate photons involves assessing several parameters. The upcoming sections will delve into photon attenuation coefficients and their associated equations. Although full derivations are found in other texts, an essential concept to understand is the Lambert–Beer law [Citation39]. This law dictates that the intensity of a monochromatic beam (initially I0) diminishes exponentially as it travels through a material with a certain thickness (x) and a linear radiation attenuation coefficient (LAC, μ). The mathematical representation of this law is as follows:
(1)
(1) The mass attenuation coefficient (MAC) serves as a vital indicator, evaluating the likelihood of photon-matter interaction by considering the density of the absorptive material; it is obtained by dividing the linear radiation attenuation coefficient (LAC) by the material’s density. It provides valuable information about the characteristics of photon-matter interactions and how they vary with the material’s density. The formula for this calculation is as follows [Citation40, Citation41].
(2)
(2) In the given context, wi denotes the weight fraction of the ith constituent element, and ρ represents the sample’s density. The sample’s ability to attenuate radiation is influenced by several factors, including photon energy, atomic number, material thickness, and density, among others [Citation42]. The increased atomic number (Z) of an absorbing material greatly increases the probability of interactions between gamma ray photons and the atoms of the substance. This may be ascribed to the correlation between higher atomic numbers and an increased quantity of electrons available for interaction. When gamma ray photons interact with these atoms, the likelihood of photon absorption or scattering is increased. This results in a reduction in the transmission of photons through the material, hence increasing the amount of attenuation [Citation38]. The efficacy of a substance in providing protection against gamma ray photons mostly relies on its LAC and MAC values, with the MAC, in particular, playing a crucial role in calculating other attenuation parameters to assess the efficacy of chosen samples as shielding materials [Citation13]. These parameters consist of the half value layer (HVL) and the tenth value layer (TVL). These parameters, half-thickness and one-tenth thickness, denote the average thickness of the absorber needed to attenuate a half and a tenth of the incident photon intensity, respectively. Significantly, lower HVL and TVL values imply a higher level of effectiveness in radiation shielding. The formulae for determining the HVL and TVL are as follows [Citation40, Citation41].
(3)
(3)
(4)
(4) Another radiation shielding parameter is the mean free path (MFP), which is defined as the average distance travelled by photons between two consecutive interactions and is inversely proportional to μ as [Citation40,Citation41]:
(5)
(5) Materials characterized by high density typically have a shorter mean free path (MFP), making them particularly effective as barriers against gamma rays. The shorter the MFP, the more effective the material is at attenuating radiation. Another important parameter in evaluating a material’s shielding capability is the effective atomic number (Zeff). This number represents the relative number of electrons in the attenuating material that participate in photon-atom interactions. It serves as a composite metric that effectively summarizes the overall electron density and interaction potential of a material, which are critical factors in determining how well a material can attenuate gamma photons. Zeff can be computed utilizing specific equations, which take into account the proportionate contribution of each constituent element in the material to the overall photon-atom interaction processes. Thus, Zeff allows for a more nuanced understanding of a material’s shielding characteristics, particularly in terms of its electron interaction capacity, and can be written as [Citation43]:
(6)
(6) Where: Ai: the atomic weight, Zi: the atomic number, fi: the mole fraction of each constituent element.
The Effective Buildup Factor (EBF) and Effective Absorption Buildup Factor (EABF) are pivotal for comprehending the cumulative impact of scattered radiation, which influences the overall detected radiation. EBF estimates the ratio of all detected gamma contributions (primary and secondary) to the contribution of undetected gammas (primary radiation). EABF considers energy deposition by primary and secondary radiation in comparison to energy deposition by primary radiation alone. The estimation of the buildup factors for the investigated glasses employs the G-P fitting methodology, which involves three steps [Citation40, Citation44, Citation45]. Firstly, the equivalent atomic numbers (Zeq) of the samples were calculated using logarithmic interpolation method, as in equation (7):
(7)
(7) Where R1 and R2 represent the (μm)Compton/(μm)Total ratios of the adjacent elements with atomic numbers Z1 and Z2, respectively. Secondly, the calculation of G-P fitting parameters for the glasses involves a similar logarithmic interpolation procedure in Zeq, as follows:
(8)
(8) At a given energy, the G-P fitting parameters, represented as P1 and P2, correspond to the atomic numbers Z1 and Z2, respectively. Finally, the G-P fitting parameters (b, c, a, Xk and d) were used to estimate the buildup factors using the following formula:
(9)
(9) Where,
(10)
(10) Where X represents the source-detector distance (x ≤ 40 MFP), b represents the EBF value at 1 mfp, K (E, X) signifies the dose multiplication factor, and b, c, a, XK, and d are computed G-P fitting parameters in the photon energy range of 0.015–15 MeV.
Therefore, this method is integral for understanding how radiation intensifies within a material due to photon scattering and absorption processes.
Neutrons, uncharged subatomic particles, interact with mediums through a variety of mechanisms including neutron capture, nuclear fission, and both elastic and inelastic scattering, as well as through nuclear spallation processes. These interactions are crucial for understanding how materials behave under neutron bombardment. A key metric in this context is the fast neutron removal cross section (ΣR or FNRCS). This measure evaluates a material’s efficacy in attenuating neutrons. A high ΣR value is indicative of a material’s robust capability to impede neutron passage. The ΣR can be conceptualized as a quantitative indicator of a material’s effectiveness in reducing the intensity and penetration of fast neutrons, which is vital for materials used in neutron shielding applications. The definition and calculation of ΣR involve considering the likelihood of various neutron-material interactions and quantifying the material’s capacity to absorb or scatter fast neutrons, thereby reducing their presence in a given environment [Citation46]. For a compound or homogeneous mixture, ΣR can be determined by employing the ΣR/ρ values of the constituent elements. The ΣR value can be computed using the following formulas:
(11)
(11)
(12)
(12) Where
represents the mass removal cross-section, wi is the weight percentage, and
corresponds to the partial density of the ith constituent.
2.3. MCNPX simulations for mass attenuation coefficients
Monte Carlo simulations were conducted using the MCNPX (version 2.7.0) general-purpose code, which is designed for complex neutron, photon, and electron transport. The simulation environment was calibrated to reflect an accurate representation of the radiation interaction within the materials studied, specifically barium-doped tellurite glasses of various compositions. The simulation setup, as depicted in Figure , comprised a modelled detector and a collimation system to ensure a directed beam of radiation towards the glass sample. The geometry was defined without energy cut-off to capture the full spectrum of potential interactions. Glass samples were labelled according to their barium oxide content, ranging from BaTBiB-1 to BaTBiB-5. The materials used in the simulation included the barium-doped tellurite glasses, a lead block to represent a standard shielding material for comparison, and the source of radiation. The glass samples were defined with compositions as per the glass codes assigned. The F4 tally mesh was employed within the modelled detector to measure the flux averaged over the cell volume, which provided data on the radiation absorbed and allowed for the calculation of mass attenuation coefficients. The obtained simulation results were examined and juxtaposed with the theoretical data derived from the Phy-X/PSD software for comprehensive analysis and comparison. Table presents the simulation outcomes alongside the Phy-X/PSD results and the associated deviations. The comparative analysis demonstrated good harmony between the simulated data and the theoretical model, confirming the reliability of the simulation setup.
Figure 2. 2D MCNPX simulation setup of mass attenuation coefficient calculations obtained from MCNPX Visual Editor.

Table 2. Mass attenuation coefficients (cm2/g) of BaTBiB-1 to BaTBiB-5 glasses obtained through Phy-X and MCNPX Monte Carlo simulation code.
3. Results and discussion
In the current study, the shielding parameters were evaluated for five different compositions of tellurite glasses with various amounts of BaO (%mol) using Phy-X simulation software. The following subsections present a thorough examination and discussion of the findings obtained in this study.
3.1. Mass and linear attenuation coefficients
The mass attenuation coefficient (MAC), measured in cm2/g, is a crucial metric for evaluating the absorption properties of shielding materials, especially for gamma radiation. A higher MAC value is desirable for enhanced shielding efficacy. Figure in the paper illustrates the changes in MAC values against photon energy for glasses consisting of different proportions of BaO, TeO2, Bi2O3, and B2O3, as specified in Table . The data shows that the maximum values of MAC for all the considered glasses analysed occur at lower energies (E < 500 keV). As the energy of photons rises, the MAC values exhibit a consistent decrease. Notably, there is a slight increase in MAC values at around 100 keV, which aligns with the K absorption edge of bismuth (90.53 keV). Between 0.5 and 5 MeV, the MAC values decrease at a slower rate, whereas at higher energies, up to 15 MeV, they decrease further and reach a practically constant level. The diverse patterns seen in Figure may be elucidated by using the principles of basic radiation physics. The gamma photons’ interaction with the glass samples is greatly affected by two main factors: the material’s atomic number (Z) and the photon energy. Therefore, at different energy levels, there are distinct mechanisms through which photons interact with matter. These mechanisms include photoelectric absorption (PE), Compton scattering (CS), and pair production (PP). Each of these processes predominates at specific energy ranges, with photoelectric absorption being prevalent at low energies, Compton scattering at intermediate energies, and pair production at high energies [Citation47]. In the low-energy region, all considered glasses exhibit significant mass attenuation coefficient (MAC) values, where potential energy (PE) is the major factor. The high value in PE is a result of the negative correlation with photon energy (1/E3.5) and the positive correlation with atomic number (Z4). Among the investigated glass samples, the BaTBiB-5 sample stands out due to its composition, which includes a significant number of elements with high atomic numbers. As a result, this glass sample demonstrates the highest values for the mass attenuation coefficient (MAC) when compared to the other samples. As the energy of photons rises, the MAC values decline exponentially and finally reach a stable level. This tendency is related to the cross-section of the CS mechanism, which is directly proportional to (1/E) and (Z). Within the high-energy range, the PP process, which exhibits a cross-section that is directly proportional to Z2 [Citation48], results in a marginal augmentation of MAC values. The research also involves a comparison of the MAC values of the glasses, which were computed using Phy-X/PSD software, with the results obtained from MCNPX simulation. This comparison is shown in Table . Validating the efficiency and dependability of the shielding materials under research is of utmost importance in this comparison. The findings produced from both methodologies demonstrate a significant level of closeness, with an average percentage variance of 3.14. The deviation % between the two programs has been determined using the subsequent formula:
The linear attenuation coefficient (LAC) serves as a fundamental and valuable parameter for assessing the efficacy of glasses in providing shielding against gamma radiation. The LAC values of the studied glass samples followed a similar trend to the mass attenuation coefficients. Figure demonstrates that BaTBiB-5 again possessed the highest LAC throughout the energy range under investigation. At lower energy levels, the effective attenuation of the glass structure was facilitated by the dense arrangement of elements with significant atomic numbers. As the energy levels shifted to the mid-range, BaTBiB-5 maintained a significant attenuation capability, although the distinctions between the samples became less noticeable. At higher energies, the variations in LAC between the different samples were less prominent, but BaTBiB-5 still maintained its lead, albeit with a narrower margin.
3.2. Half and tenth value layers (HVL, TVL)
The half-value layer (HVL) is an essential measure used to assess how effectively glasses provide shielding against radiation. It serves as a crucial indicator of the thickness needed to reduce the initial photon count by half. It is necessary to mention that a low HVL value in a material indicates improved shielding abilities due to the increased probability of photon-material interaction [Citation49]. Figure in the study presents the HVL values for the BaTBiB glass samples, providing a basis for both qualitative and quantitative analysis with several important findings. Firstly, the HVL trends across the glass samples are consistent. This uniformity in behaviour is significant as it suggests a predictable pattern of photon attenuation across different compositions of the same type of glass. Secondly, the HVL values are observed to be lower at lower photon energies, increasing progressively with the rise in energy levels, spanning a range from 0.001 to 3.14 cm. This variation is crucial as it indicates the glasses’ changing efficiency in shielding against photons of different energy levels. Thirdly, the research highlights that the HVL values of the BaTBiB glasses exhibit independence from their composition when low photon energies are considered. This result implies that, at these lower energy levels, the specific makeup of the tellurite glasses does not significantly influence their photon attenuation capability, as they all exhibit nearly identical HVL values. Nevertheless, in the moderate and high-energy ranges, a discernible change occurs as the HVL values demonstrate a distinct correlation with the chemical composition of the glasses. This finding is particularly relevant as it highlights the importance of composition in determining the effectiveness of glass as a shielding material against higher-energy photons. Such insights are essential for the development and optimization of radiation shielding materials, especially in contexts where specific energy ranges of photons are of concern. Specifically, BaTBiB-1 glass demonstrates the highest HVL values, while BaTBiB-5 glass exhibits the lowest HVL values. Figure presents a comparison of the HVL values between BaTBiB glass samples and commonly available shielding glass samples namely RS-253 G18 (ρ = 2.53 g/cm3), RS-360 (ρ = 3.6 g/cm3) and RS-520 (ρ = 5.2 g/cm3), as well as various types of concretes, namely ordinary (ρ = 2.3 g/cm3), hematite–serpentine (ρ = 2.5 g/cm3), ilmenite–limonite (ρ = 2.9 g/cm3) and basalt–magnetite (ρ = 3.05 g/cm3), ilmenite (ρ = 3.5 g/cm3), steel-scrap (ρ = 4 g/cm3) and steel-magnetite (ρ = 5.11 g/cm3) concretes. The comparison reveals that at all energies, the HVL thicknesses of all selected glasses (BaTBiB-1–BaTBiB-5) are lower than those of the commercial samples. Thus, these results indicate that the chosen BaTBiB glasses exhibit superior photon shielding performance compared to the commercially investigated materials. Furthermore, the evaluation of a glass’s attenuation ability can also be determined by its tenth value layer (TVL) value. It is widely acknowledged that materials with lower TVL values require less thickness to effectively block gamma radiation photons. Figure showcases the TVL measurements for the BaTBiB glasses, which exhibit similar trends to those observed in the HVL measurements illustrated in Figure . The TVL shows an increase with higher incident photon energy and a decline as the mol% of BaO content (or the sample’s density) increases. For example, in the case of BaTBiB-1 (density = 5.73 g/cm3), the TVLs range from 5.98 cm to 8.62 cm at 1 and 15 MeV, respectively. Similarly, for BaTBiB-5 (density = 6.12 g/cm3), the TVLs range from 5.55 cm to 7.97 cm at the same energy levels. Of particular importance is the observation that the BaTBiB samples consistently exhibited their maximum TVL values at 5 MeV.
Figure 5. Half value layer (HVL) thickness of the studied glass samples as a function of photon energy (MeV).

3.3. Mean free path
In the study, the mean free path (MFP) was analysed to understand how gamma photons are attenuated in the prepared glass samples. Figure showcases the relationship between MFP and photon energy for glasses with different BaO concentrations. A key observation from the graph is that the MFP increases with rising photon energy. This trend signifies that the glass samples are more penetrable by photons with higher energy. The reason behind this is that the probability of photons interacting with the glass material undergoes a substantial decrease as the energy levels rise. Consequently, the glasses demonstrate greater effectiveness in attenuating photons of lower energy while allowing a more pronounced transmission of photons with higher energy. Further, Figure highlights that among the prepared glasses, BaTBiB-5 exhibits the greatest MFP, while BaTBiB-1 has the smallest. This variation suggests that increasing the BaO content in the glass composition results in a reduction of the MFP, enhancing the glass’s shielding ability. To evaluate the practical effectiveness of these glasses, the study compares their MFP values with those of standard shielding materials as shown in Figure . Interestingly, the graph shows that all the tested glasses, including the BaTBiB varieties, have lower MFP values compared to standard shielding glasses and concrete materials. This result is significant as it demonstrates the superior shielding capabilities of the BaTBiB glasses compared to conventional materials. The observed results present promising prospects for potential application and practical use of BaTBiB glasses as transparent materials for radiation shielding. The MFP curve also suggests that the presence of BaO within the glass composition not only enhances the attenuation properties of the BaO–TeO2–Bi2O3–B2O3 glasses but also contributes to their suitability for practical shielding applications. This insight is invaluable for the development of advanced and highly efficient shielding materials, particularly in fields where transparency and effectiveness in attenuating a range of photon energies are required.
3.4. Effective atomic number (Zeff)
The effective atomic number (Zeff) is a crucial parameter for assessing the attenuation capabilities of a glass system against radiation. Higher Zeff values in a glass composition signify an enhanced ability to attenuate or block radiation. Zeff, derived from linear attenuation coefficient (LAC) values, is widely used to analyse how the composition of a material affects its photon attenuation characteristics. Figure in the study illustrates consistent patterns of Zeff of all investigated glasses. The most notable aspect of these trends is that Zeff values reach their maximum at lower energy levels. This peak is primarily due to the significant influence of the Z4-dependent photoelectric absorption process. Additionally, there’s a noticeable small peak around 100 keV, which corresponds to the k-absorption edge of Bi. After the photoelectric region, a distinct region resembling a valley emerges between 0.2 and 3 MeV, where Compton scattering takes precedence as the primary interaction, resulting in the lowest Zeff values. Beyond 3 MeV, there is a pronounced rise in Zeff, primarily due to the increasing dominance of the Z2-dependent pair production process. Furthermore, Figure showcases a notable augmentation in Zeff values across all energy levels as a result of incorporating BaO into the glass composition. As an illustration, when considering an energy level of 0.1 MeV, the Zeff values for BaTBiB-1 through BaTBiB-5 progressively increase, with BaTBiB-5 displaying the highest Zeff value, indicating its superior ability to attenuate incident gamma photons compared to the other samples. This gradual increase across the BaTBiB series underscores the role of BaO in enhancing the glasses’ ability to attenuate photons. In summary, these findings emphasize the influence of BaO on the effectiveness of radiation shielding of the BaTBiB samples, with BaTBiB-5 emerging as the most effective in terms of attenuating gamma photons.
3.5. Energy absorption and exposure buildup factors (EABF and EBF)
In the study, a thorough assessment of the radiation protection performances of the glass samples was conducted by calculating EBF and EABF parameters. Several key factors, such as the effective atomic number of the medium, the energy of gamma rays, and the penetration depth, significantly impact the buildup factor. Tables , , , , list the equivalent atomic numbers (Zeq) and GP fitting parameters of the EBF and EABF for the five BaTBiB glasses, covering a wide energy spectrum from 0.015 to 15 MeV and penetration distances ranging from 0.5 to 40 mfp. Both EBF and EABF are crucial for understanding the cumulative effect of scattered radiation, which can add to the dose beyond the primary beam’s impact. Figures and in the study indicate that at lower energies (≤0.1 MeV), both EABFs and EBFs display minimal values. The primary reason for this pattern can be explained by the dominant influence of the photoelectric effect at these energy levels. As a result, the majority of photons are fully absorbed, leading to a decrease in build-up values. Around 0.1 MeV, there is a noticeable increase in both parameters for all glass compositions. This rise is linked to the K-absorption edges of Bi (99.53 keV), influencing the variations in EBF and EABF. As photon energy increases, there is a gradual and slight uptick in both EBF and EABF values. This increase is associated with the multiple scattering events, predominantly through the Compton scattering process, up to 5 MeV. Beyond this energy, a significant rise in both EBF and EABF values is observed for all penetration depths, ascribed to PP process in this energy region. This process results in a higher generation of secondary photons, especially notable with the increasing depth of penetration. An important observation is that the EBF and EABF values for all samples trend upwards with increasing penetration depths at a given photon energy. This pattern suggests an enhanced probability of photon absorption as the depth of interaction within the medium increases. Of particular note is that the BaTBiB-5 glass sample, which contains the highest BaO content of 25 mol%, consistently exhibits the most minimal EBF and EABF values among all mean free path (mfp) values. Figure specifically highlights this pattern at a penetration depth of 5 mfp. This finding underscores that higher concentrations of BaO in glass compositions can significantly boost radiation protection efficiency, even at higher mfp values where photon build-up is more significant. These results are critical in demonstrating the potential of BaO-enriched glass compositions in enhancing radiation shielding, especially in scenarios involving deep penetration and high photon energies. Such insights are invaluable for the development of effective radiation protection materials in various applications.
Table 3. G-P fitting coefficients for the BaTBiB-1 glass sample, corresponding to the EBF and EABF parameters.
Table 4. G-P fitting coefficients for the BaTBiB-2 glass sample, corresponding to the EBF and EABF parameters.
Table 5. G-P fitting coefficients for the BaTBiB-3 glass sample, corresponding to the EBF and EABF parameters.
Table 6. G-P fitting coefficients for the BaTBiB-4 glass sample, corresponding to the EBF and EABF parameters.
Table 7. G-P fitting coefficients for the BaTBiB-5 glass sample, corresponding to the EBF and EABF parameters.
Figure 11. Variation of exposure buildup factors (EBF) as a function of increasing photon energy at different mean free path (mfp) values.

3.6. Fast neutron removal cross-section (ΣR)
The fast neutron removal cross-section (ΣR), measured in cm−1, is a vital metric for evaluating a material’s effectiveness in attenuating fast neutrons, typically found in fission energy scenarios. This parameter measures the likelihood of a primary interaction leading to the elimination of an incoming neutron, essentially gauging the material’s proficiency in blocking or reducing the intensity of a neutron beam. In the study, Figure presents the ΣR values for the BaTBiB series of glasses. These values are 0.10179, 0.10263, 0.10362, 0.10581, and 0.10623 cm−1, respectively. Notably, the BaTBiB-5 sample, which contains the highest proportion of BaO, records the highest ΣR value within the series. This observation is significant as it indicates that the presence of BaO in the glass composition substantially enhances its ability to provide effective neutron shielding. The higher ΣR value of BaTBiB-5 suggests that it is more capable of attenuating fast neutrons compared to the other samples in the series. This enhancement in neutron shielding capability can be attributed to the specific properties of BaO, which contribute to a more effective interaction with fast neutrons, thereby increasing the probability of their removal from the neutron flux. This finding is particularly relevant in contexts where neutron shielding is crucial, such as in nuclear reactors or certain medical applications. The ability of the BaTBiB-5 glass to provide enhanced protection against fast neutrons, as indicated by its higher ΣR value, marks it as a potentially valuable material for such applications, where effective neutron attenuation is a key requirement.
This study has shown that superior shielding features were achieved with the highest amount of BaO. These results align with previous studies, which have also observed similar outcomes when there is an increased incorporation of heavy metals in the shielding structures [Citation50–58].
4. Conclusion
The study analysed gamma-ray and neutron shielding parameters for a series of tellurite glasses with compositions xBaO-(30-x)TeO2-35Bi2O3-35B2O3, where the BaO content varied from 5 to 25 mol%. This evaluation was conducted using the Phy-X/PSD software and covered a broad energy spectrum from 0.015 to 15 MeV. The investigation included a detailed assessment of several key parameters: MAC, LAC, HVL, TVL, MFP, Zeff, EBF, EABF and FNRCS. This study has yielded several crucial findings, which are summarized as follows:
The MAC and LAC values reached their peak at the lowest energy level, with BaTBiB-5 exhibiting the highest values among all the studied glasses.
Conversely, the values for HVL, TVL and MFP showed an inverse relationship with BaO content, following the sequence from BaTBiB-1 (highest) to BaTBiB-5 (lowest).
A comparative analysis of HVL and MFP values between the BaTBiB glass samples and commonly used shielding materials (RS-253 G18, RS-360, RS-520, ordinary, hematite-serpentine, ilmenite-limonite, basalt-magnetite, ilmenite, steel-scrap, and steel-magnetite concretes) highlighted the superior shielding capabilities of the studied tellurite glasses.
Furthermore, Zeff exhibited an increase with the progressive increment of BaO content within the studied samples, attributed to the variations in atomic numbers and atomic weights of the constituent elements.
The G-P fitting method was employed to calculate the EBF and EABF values within the energy range of 0.015–15 MeV up to 40 MFP. Notably, among the glasses studied, BaTBiB-5 glass displayed the lowest EABF and EBF values, demonstrating its superior performance compared to the other glasses.
In terms of neutron shielding, the lowest ΣR value was recorded for the BaTBiB-1 glass (0. 10179 cm−1), whereas the highest ΣR was observed in the BaTBiB-5 glass (0.10623 cm−1).
In light of the findings of this comprehensive study, it can be argued that the BaTBiB-5 glass sample (25BaO-5TeO2-35Bi2O3-35B2O3) with the highest BaO content, exhibits superior attenuation characteristics against gamma radiation and neutron particles as well. This makes it a promising candidate for applications requiring efficient radiation shielding. In addition, this insight is valuable for the development and selection of materials for radiation shielding, particularly in applications where controlling exposure to various energy levels of gamma radiation is critical. The findings of the present study should be regarded as preliminary, underscoring the critical need for subsequent experimental investigations. Furthermore, it is crucial to conduct additional research on the utilized tellurite glass system, incorporating diverse doping elements. This will contribute to expanding our knowledge about this glass system, providing a deeper understanding of its properties and behaviour.
Disclosure statement
No potential conflict of interest was reported by the author(s).
References
- Issa SAM, Ali AM, Tekin HO, et al. Enhancement of nuclear radiation shielding and mechanical properties of YBiBO3 glasses using La2O3. Nucl Eng Technol. 2020;52:1297–1303. doi:10.1016/j.net.2019.11.017
- Alrowaili ZA, Alwadai N, Eke C, et al. Influence of BaO/ZnCl2 content on gamma transmission properties of the optical TeO2-based glass system. Optik (Stuttg). 2022;270:170054. doi:10.1016/j.ijleo.2022.170054
- Sayyed MI, Akman F, Geçibesler IH, et al. Measurement of mass attenuation coefficients, effective atomic numbers, and electron densities for different parts of medicinal aromatic plants in low-energy region. Nucl Sci Tech. 2018;29:1–10. doi:10.1007/s41365-018-0475-0
- Issa SAM, Ali AM, Susoy G, et al. Mechanical, physical and gamma ray shielding properties of xPbO-(50-x) MoO3–50V2O5 (25≤ x≤ 45 mol%) glass system. Ceram Int. 2020; 46:20251–20263.
- Kaur P, Singh KJ, Thakur S, et al. Investigation of bismuth borate glass system modified with barium for structural and gamma-ray shielding properties. Spectrochim Acta A Mol Biomol Spectrosc. 2019;206:367–377. doi:10.1016/j.saa.2018.08.038
- Abouhaswa AS, Zakaly HMH, Issa SAM, et al. Synthesis, physical, optical, mechanical, and radiation attenuation properties of TiO2–Na2O–Bi2O3–B2O3 glasses. Ceram Int. 2021;47:185–204. doi:10.1016/j.ceramint.2020.08.122
- Şengul A, Akgüngör K, Akkurt I. Gamma-ray shielding properties of some dosimetric materials. J Aust Ceram Soc. 2023;59:117–126. doi:10.1007/s41779-022-00817-z
- ALMisned G, Günoğlu K, Özkavak HV, et al. An investigation on gamma-ray and neutron attenuation properties of multi-layered Al/B4C composite. Mater Today Commun. 2023;36:106813. doi:10.1016/j.mtcomm.2023.106813
- Sayyed MI, Akyildirim H, Al-Buriahi MS, et al. Oxyfluoro-tellurite-zinc glasses and the nuclear-shielding ability under the substitution of AlF 3 by ZnO. Appl Phys A. 2020;126:1–12. doi:10.1007/s00339-019-3265-6
- Zakariya NI, Kahn MTE. Benefits and biological effects of ionizing radiation, Sch Acad J Biosci. 2014;2:583–591.
- Desouky O, Ding N, Zhou G. Targeted and non-targeted effects of ionizing radiation. J Radiat Res Appl Sci. 2015;8:247–254.
- More CV, Alsayed Z, Badawi MS, et al. Polymeric composite materials for radiation shielding: a review. Environ Chem Lett. 2021;19:2057–2090. doi:10.1007/s10311-021-01189-9
- Tekin HO, Cavli B, Altunsoy EE, et al. An investigation on radiation protection and shielding properties of 16 slice computed tomography (CT) facilities. Int J Comput Exp Sci Eng. 2018;4:37–40. doi:10.22399/ijcesen.408231
- Singh J, Singh H, Sharma J, et al. Fusible alloys: a potential candidate for gamma rays shield design. Progr Nucl Energy. 2018;106:387–395. doi:10.1016/j.pnucene.2018.04.002
- Babeer AM, Sayyed MI, Morshidy HY, et al. High transparency of PbO–BaO–Fe2O3–SrO–B2O3 glasses with improved radiation shielding properties. Opt Mater. 2023;145:114387. doi:10.1016/j.optmat.2023.114387
- Al-Hadeethi Y, Sayyed MI. Effect of Gd2O3 on the radiation shielding characteristics of Sb2O3–PbO–B2O3–Gd2O3 glass system. Ceram Int. 2020;46:13768–13773. doi:10.1016/j.ceramint.2020.02.166
- El-Taher A, Ali AM, Saddeek YB, et al. Gamma ray shielding and structural properties of iron alkali alumino-phosphate glasses modified by PbO. Radiat Phys Chem. 2019;165:108403. doi:10.1016/j.radphyschem.2019.108403
- Issa SAM, Sayyed MI, Mostafa AMA, et al. Investigation of mechanical and radiation shielding features of heavy metal oxide based phosphate glasses for gamma radiation attenuation applications. J Mater Sci Mater Electron. 2019;30:12140–12151. doi:10.1007/s10854-019-01572-x
- Waly E-SA, Al-Qous GS, Bourham MA. Shielding properties of glasses with different heavy elements additives for radiation shielding in the energy range 15–300 keV. Radiat Phys Chem. 2018;150:120–124. doi:10.1016/j.radphyschem.2018.04.029
- Tekin HO, Issa SAM, Mahmoud KA-A, et al. Nuclear radiation shielding competences of barium-reinforced borosilicate glasses. Emerg Mater Res. 2020;9:1131–1144.
- Sayyed MI, Ali AA, Tekin HO, et al. Investigation of gamma-ray shielding properties of bismuth borotellurite glasses using MCNPX code and XCOM program. Appl Phys A. 2019;125:1–7. doi:10.1007/s00339-019-2739-x
- Rammah YS, Kilic G, El-Mallawany R, et al. Investigation of optical, physical, and gamma-ray shielding features of novel vanadyl boro-phosphate glasses. J Non Cryst Solids. 2020;533:119905. doi:10.1016/j.jnoncrysol.2020.119905
- Sidkey MA, El Mallawany RA, Abousehly AA, et al. Relaxation of longitudinal ultrasonic waves in some tellurite glasses. Mater Chem Phys. 2002;74:222–229. doi:10.1016/S0254-0584(01)00466-7
- Dong M, Elbashir BO, Sayyed MI. Enhancement of gamma ray shielding properties by PbO partial replacement of WO3 in ternary 60TeO2–(40-x) WO3–xPbO glass system. Chalcogenide Lett. 2017;14:113–118.
- Tekin HO, Kassab LRP, Issa SAM, et al. Structural and physical characterization study on synthesized tellurite (TeO2) and germanate (GeO2) glass shields using XRD, Raman spectroscopy, FLUKA and PHITS. Opt Mater (Amst). 2020;110:110533. doi:10.1016/j.optmat.2020.110533
- Jha A, Richards B, Jose G, et al. Rare-earth ion doped TeO2 and GeO2 glasses as laser materials. Prog Mater Sci. 2012;57:1426–1491. doi:10.1016/j.pmatsci.2012.04.003
- Ersundu MÇ, Ersundu AE, Sayyed MI, et al. Evaluation of physical, structural properties and shielding parameters for K2O–WO3–TeO2 glasses for gamma ray shielding applications. J Alloys Compd. 2017;714:278–286. doi:10.1016/j.jallcom.2017.04.223
- Biswas J, Jana S. Visible luminescence and energy migration mechanism of Sm3+ in phospho-tellurite glasses by co-activating with Tb3+ ions for solid state lighting device applications. Phys B Condens Matter. 2023;657:414812. doi:10.1016/j.physb.2023.414812
- Lu Y, Cai M, Cao R, et al. Ho3+ doped germanate-tellurite glass sensitized by Er3+ and Yb3+ for efficient 2.0 µm laser material. Mater Res Bull. 2016;84:124–131. doi:10.1016/j.materresbull.2016.07.017
- Boonin K, Yasaka P, Limkitjaroenporn P, et al. Effect of BaO on lead free zinc barium tellurite glass for radiation shielding materials in nuclear application. J Non Cryst Solids. 2020;550:120386. doi:10.1016/j.jnoncrysol.2020.120386
- Gao S, Liu X, Kang S, et al. 2–3 µm emission and fluorescent decaying behavior in Ho3+-doped tellurium germanate glass. Opt Mater (Amst). 2016;53:44–47. doi:10.1016/j.optmat.2015.12.045
- Alzahrani JS, Muniz RF, Alrowaili ZA, et al. A synergistic effect of heavy metal oxides to enhance the physical, optical, and radiation-absorption properties of TeO2-Li2O-BaO glasses. Optik (Stuttg). 2022;261:169189. doi:10.1016/j.ijleo.2022.169189
- Srinivas B, Bhogi A, Naresh P, et al. Fabrication, optical and radiation shielding properties of BaO-TeO2-B2O3-Cr2O3 glass system. Optik (Stuttg). 2022;258:168877. doi:10.1016/j.ijleo.2022.168877
- Rammah YS, Tekin HO, Sriwunkum C, et al. Investigations on borate glasses within SBC-Bx system for gamma-ray shielding applications. Nucl Eng Technol. 2021;53:282–293. doi:10.1016/j.net.2020.06.034
- Vani P, Vinitha G, Sayyed MI, et al. Investigation on structural, optical, thermal and gamma photon shielding properties of zinc and barium doped fluorotellurite glasses. J Non Cryst Solids. 2019;511:194–200. doi:10.1016/j.jnoncrysol.2019.02.005
- Şakar E, Özpolat ÖF, Alım B, et al. PhyPhy-X/PSD: development of a user friendly online software for calculation of parameters relevant to radiation shielding and dosimetry. Radiat Phys Chem. 2020;166:108496. doi:10.1016/j.radphyschem.2019.108496
- Srinivas B, Hameed A, Chary MN, et al. Physical, optical and FT-IR studies of bismuth-boro-tellurite glasses containing BaO as modifier. in: IOP Conf Ser Mater Sci Eng, IOP Publishing. 2018; p. 12022.
- Podgorsak EB. Interaction of photons with matter, compendium to radiation physics for medical physicists: 300 problems and solutions; 2014; p. 387–514.
- Swinehart DF. The beer-Lambert law. J Chem Educ. 1962;39:333. doi:10.1021/ed039p333
- Sayyed MI, Tekin HO, Agar O. Gamma photon and neutron attenuation properties of MgO–BaO–B2O3–TeO2–Cr2O3 glasses: the role of TeO2. Radiat Phys Chem. 2019;163:58–66. doi:10.1016/j.radphyschem.2019.05.012
- Ali AM, Rammah YS, Sayyed MI, et al. The impact of lead oxide on the optical and gamma shielding properties of barium borate glasses. Appl Phys A. 2020;126:1–9.
- Mansouri E, Mesbahi A, Malekzadeh R, et al. Shielding characteristics of nanocomposites for protection against X-and gamma rays in medical applications: effect of particle size, photon energy and nano-particle concentration. Radiat Environ Biophys. 2020;59:583–600. doi:10.1007/s00411-020-00865-8
- More CV, Lokhande RM, Pawar PP. Effective atomic number and electron density of amino acids within the energy range of 0.122–1.330 MeV. Radiat Phys Chem. 2016;125:14–20. doi:10.1016/j.radphyschem.2016.02.024
- Issa SAM, Ahmad M, Tekin HO, et al. Effect of Bi2O3 content on mechanical and nuclear radiation shielding properties of Bi2O3-MoO3-B2O3-SiO2-Na2O-Fe2O3 glass system. Results Phys. 2019;13:102165. doi:10.1016/j.rinp.2019.102165
- Lokhande RM, More CV, Surung BS, et al. Determination of attenuation parameters and energy absorption build-up factor of amine group materials. Radiat Phys Chem. 2017;141:292–299. doi:10.1016/j.radphyschem.2017.08.004
- El-Khayatt AM. Calculation of fast neutron removal cross-sections for some compounds and materials. Ann Nucl Energy. 2010;37:218–222. doi:10.1016/j.anucene.2009.10.022
- More CV, Alavian H, Pawar PP. Evaluation of gamma ray and neutron attenuation capability of thermoplastic polymers. Appl Radiat Isot. 2021;176:109884. doi:10.1016/j.apradiso.2021.109884
- Mahmoud KA, Sayyed MI, Tashlykov OL. Gamma ray shielding characteristics and exposure buildup factor for some natural rocks using MCNP-5 code. Nucl Eng Technol. 2019;51:1835–1841. doi:10.1016/j.net.2019.05.013
- More CV, Alavian H, Pawar PP. Evaluation of gamma-ray attenuation characteristics of some thermoplastic polymers: experimental, WinXCom and MCNPX studies. J Non Cryst Solids. 2020;546:120277. doi:10.1016/j.jnoncrysol.2020.120277
- Vignesh S, Winowlin Jappes JT, Nagaveena S, et al. Development of lightweight polymer laminates for radiation shielding and electronics applications. Int J Polym Sci. 2022;2022. doi:10.1155/2022/5252528
- More CV, Pawar PP, Badawi MS, et al. Thabet, Extensive theoretical study of gamma-ray shielding parameters using epoxy resin-metal chloride mixtures. Nucl Technol Radiat Prot. 2020;35:138–149. doi:10.2298/NTRP2002138M
- Kilicoglu O, More CV, Kara U, et al. Investigation of the effect of cement type on nuclear shield performance of heavy concrete. Radiat Phys Chem. 2023;209:110954. doi:10.1016/j.radphyschem.2023.110954
- More CV, Akman F, Dilsiz K, et al. Estimation of neutron and gamma-ray attenuation characteristics of some ferrites: Geant4, FLUKA and WinXCom studies. Appl Radiat Isot. 2023;197:110803. doi:10.1016/j.apradiso.2023.110803
- Vignesh S, Jappes JTW, Nagaveena S, et al. Preparation of novel in-situ layered B4C and PbO reinforced solution casted layered polymer composites (SCLPC) for augmenting the gamma irradiation shielding capability. Vacuum. 2023;207:111583. doi:10.1016/j.vacuum.2022.111583
- El-Qahtani ZMH, Shaaban ER, Soraya MM. Attenuation characteristics of high-energy radiation on As-Se-Sn chalcogenide glassy alloy. Chalcogenide Lett. 2021;18:311–326.
- Ezzeldien M, Salama MA, Hasaneen MF, et al. Multicomponent Ge-As-Te-Pb chalcogenide glasses for radiations shielding applications. Chalcogenide Lett. 2022;19. doi:10.15251/CL.2022.1912.939
- Alkallas FH, Ben Gouider Trabelsi A, Elaissi S, et al. Investigation of the gamma photon shielding in Se–Te–Ag chalcogenide glasses using the Phy-X/PSD software. Cogent Eng. 2022;9:2116829. doi:10.1080/23311916.2022.2116829
- Soraya MM, Ahmed FBM, Mahasen MM. Enhancing the physical, optical and shielding properties for ternary Sb2O3–B2O3–K2O glasses. J Mater Sci Mater Electron. 2022;33:22077–22091. doi:10.1007/s10854-022-08971-7