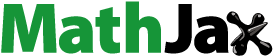
Abstract
The present work focuses on investigating magnetohydrodynamic (MHD) natural convection in a complex I-shaped enclosure with corrugated walls, filled with nanofluid and porous media layers. This study analyzes various parameters' effects on heat transfer and fluid flow. The enclosure's left and right walls maintain low temperatures, while the other walls are insulated. The inner corrugated cylinder experiences high temperatures. The study examines the impact of Rayleigh number (Ra), Hartman number (Ha), nanofluid volume fraction (ϕ), Darcy number (Da), MHD inclination angle (γ), position of the corrugated cylinder (δ) and number of undulations (N) on isotherms, streamlines, velocity profiles and average Nusselt number variations. The results show that higher Ra values increase the average Nusselt number while increasing Da enhances it by 65%. Magnetic source inclination greatly affects heat transfer, with Nusselt at γ = 90° being 15.34 compared to 10.234 at γ = 0°. The study concludes that optimal heat transfer occurs at γ = 90°, Ra = 106, Da = 10−3, Ha = 30 and N = 2.
Nomenclature
F.E.M., | = | the finite element method |
HVAC | = | Heating, Ventilation, and Air Conditioning |
MHD | = | Magnetohydrodynamics |
S | = | Minimum value equal to zero and maximum equal to 1 |
C1ε, C2ε | = | Model constant |
K | = | Thermal conductivity |
Nu | = | Local convection Nusselt number |
α | = | Thermal diffusivity, m2 /s |
ε | = | Turbulent dissipation rate |
υ | = | Kinematic viscosity of the fluid, m2 /s |
µ | = | Dynamic viscosity, Ns/m2 |
ρ | = | Density, kg/m3 |
Φ | = | Nanoparticle volume concentration |
Cp | = | Specific heat at constant pressure (kJ/kg. K) |
E | = | Position of the trapezoidal heater |
Da | = | Darcy number |
g | = | Gravitational acceleration (m/s2) |
Gr | = | Grashof number |
Ha | = | Hartmann number |
k | = | Thermal conductivity (W/m. K) |
P | = | Dimensionnels pressure |
p | = | Pressure (Pa) |
Pr | = | Prandtl number |
Ra | = | Rayleigh number |
T | = | Temperature (K) |
Nu | = | Local Nusselt number on the hot sides |
N | = | number of corrugations |
No | = | Number of hot bodies |
U | = | Dimensionless velocity component in x-direction |
V | = | Dimensionless velocity component in the y-direction |
V | = | Dimensionless coordinate in the horizontal direction |
α | = | Thermal diffusivity (m2/s) |
β | = | Volumetric coefficient of thermal expansion (K−1) |
γ | = | MHD angle |
ε | = | Porosity |
θ | = | Dimensionless temperature (T-Tc/ΔT) |
σ | = | Electrical conductivity |
Ψ | = | Dimensionless stream function |
1. Introduction
Magnetohydrodynamic (MHD) natural convective heat transfer in different shapes of enclosers is considered the main important field of study resulting from its numerous applications such as in engineering science and technology, such as cooling systems, heat exchangers, electronic equipment, nuclear reactors, geothermal engineering, and home ventilation, etc. Additionally, nanotechnology has offered a contemporary method of enhancing the fluid's heat conductivity. [Citation1]. The effect of MHD natural convection heat transfer exhibited at geometrical configurations of the enclosure. Enclosures of diverse shapes and dimensions are vital in minimizing thermal energy losses and optimizing energy production. These shapes include square, rectangular, circular, annular, triangular, trapezoidal, rhombus, and elliptical [Citation2–4]. In contrast to the previously described shapes, an additional category of enclosure geometry is called wavy geometry, characterized by a higher level of complexity. The enclosure is comprised of undulating shapes. A wavy-walled enclosure is a containment structure characterized by walls exhibiting a sinusoidal or undulating form. The undulating configuration of the walls has the potential to induce supplementary turbulence within the fluid, consequently influencing the flow dynamics. Moreover, undulating walls have resulted in a notable decrease in energy consumption and improved heat transfer efficiency within thermal systems. Various applications use water, oil, and ethylene glycol as conventional working fluids. However, a second issue arises because of these fluids. The thermophysical properties of these traditional fluids are the primary source of the problem. These fluids have a low thermal conductivity, a significant barrier to enhancing and accelerating the rate of heat transfer. So, these results have motivated others to find a suitable solution to enhance heat transfer; nanofluid appears as an active solution for large applications that depend on heat transfer enhancement. Nanofluid, known as the addition of tiny solid nanoparticles, has high thermal conductivity, such as Zn, Al, Ag, etc., to a basic fluid which may be distilled water or alcohol, glycol, and another basic fluid. The resulting fluid has very good thermophysical properties, which makes it better at moving heat. However, there are more studies on convective flow in porous media due to the action of thermal buoyancy alone [Citation5–8]. Using a finite volume scheme, the square encloser and nanofluid as a working liquid were studied first by Khanafer et al. [Citation9]. The findings were significant because it can be demonstrated that raising the Garshof number and the nanofluids’ volume fraction increases the rate of heat transfer.
Numerous researchers have investigated and reported examining nanofluids in a magnetic field enclosure. Abu-Nada and Chamkha [Citation10] utilized various models to determine the impact of the dynamic viscosity of nanofluids and thermal conductivity on the values of Nu number. Many parameters have been studied to show the effect of it on heat transfer efficiency such as Rayleigh number, aspect ratio, and concentration of nanofluid. The results suggest that the Nu number is highly affected by the dynamic viscosity than the thermal conductivity values. The authors conducted Nusselt number exhibited superior performance when the aspect ratio was characterized by a low dimensionless value, as opposed to a high geometric aspect ratio. The study conducted by Rashad et al. [Citation11] investigated the effects of various factors on a triangular enclosure filled with Cu-Al2O3. These factors included the location of the heat source, Rayleigh number, internal heat generation, Hartmann numbers, and nanofluid concentration. The researchers utilized a central finite difference scheme in their analysis. The findings show the using of hybrid nanofluids has a slight effect on heat transfer compared to conventional nanofluids. The numerical study using FEM to analyze the heat transfer by natural convection occurring within an elliptical enclosure containing a nanofluid has been conducted by Abdulkadhim [Citation12]. The enclosure also featured a circular body that was heated. The study examined the impact of the nanofluid's charge and the Rayleigh and Hartmann numbers on the inner circular cylinder's horizontal position. The findings suggest that the Rayleigh number increased with increasing the nanofluids volume fraction, while the Hartmann number decreased with it. So, this gives enhancement of heat transfer by convection.
Hatami [Citation13] employed FEM to investigate the heat transfer by convection phenomena between surfaces. The present study investigates the behaviour of an inner cylinder within a nanofluid enclosure with a wavy texture. This study examined the impact of the hot inner cylinder's dimensions and the undulating profile's height and configuration. It was determined that an increase in the internal body size results in a corresponding increase in the Nusselt number. Nonetheless, it has been observed that a critical value of dimensionless exists in this regard. This critical value marks a point beyond which a decrease in the Nusselt number is expected. The effect of MHD by using Fe3O2–water as nanofluid on natural convection in an annular encloser equipped with an inner hot cylinder was studied by Sheikholeslami et al. [Citation14]. The effect of different parameters studied such as nanoparticle ϕ, Ra, Ha, and γ. The findings demonstrated that an increase in Ra number enhanced the Nu. On the other hand, Nu decreases with increasing value Ha. This paragraph highlights the identification of the wavy enclosure filled with substances such as nanofluids, pure fluids, porous medium, etc. have more effect on the natural convection.
Abdelmalek [Citation15] conducted a numerical analysis to show the effects of various arrangements of wavy inner bodies submerged by a square enclosure full of nanofluid, utilizing the CVFEM method. Their findings indicate that increased undulations result in a higher heat transfer rate and a weaker fluid flow.
Oztop et al. [Citation16] employed the F.V. to present the impact of heat source volumetric and wave amplitudes on the rate of heat transfer within a wavy enclosure. Ma et al. [Citation17] investigated four separate cases of thermal arrangements of internal bodies in an I-shaped enclosure that is filled with nanofluid. The researchers examined the effect of Raylie number, Hartman number, and the MHD inclination angle on fluid transfer. Many scientists have examined how convection heat transfer becomes in cavities with different shapes, like circles, squares, and triangles. Kim et al [Citation18] used the F.V.M to solve the convection heat transfer in transient conditions between circular cylinders inside a square enclosure at different heights and Ra. Hussain and Hussein [Citation19] showed the effect of natural convection and fluid flow by putting a solid cylinder inside a rectangular box containing air. J. Lee [Citation20] shows the effect of the position of a heated cylinder inside a square enclosure mounted at low temperatures on natural convection. The results show that convection heat transfer is affected by the position and these results are validated with the results of Choi, C. et al. [Citation21] under transient conditions. Massoudi et al. [Citation22] investigated numerically the effect using nanofluid consisted Nanotube (CNT), 50% water, and 50% Ethylene Glycol with a magnetic field on the performance of heat sinks with different shapes of fins at a range of Ra (103 ≤ Ra ≤ 106), and Ha. (0 ≤ Ha ≤ 30) and the results proved that the efficiency of convection cooling liquid is supported by increasing Rayleigh number. In another research, Massoudi et al. [Citation23] studied numerically the MHD of MWCNT/water nanofluid within T- cavity. The data reveals that convective heat reduced with Lorentz powers. Natural convection heat transfer in the enclosure as W-shaped presented by Dhia et al. [Citation24] at different aspect ratios. Ag/Al2O3 hybrid nanofluid saturated with porous medium with uniform heat generation. The results showed that the performance of convection heat transfer can be ameliorated by the addition of hybrid nanoparticles, also, it increased with increasing of Rayleigh and Darcy numbers. Alshare, Aiman, et al [Citation25] investigated the effect of a magnetic field on mixed convection in a corrugated cavity filled with nanofluid at a volume fraction (ϕ) ranging (0-0.08%). Several undulation numbers (N), are considered. The results reveal that heat transfer has been augmented, also, the Nusselt number increases with increasing number of undulations, and ϕ. The effect of a magnetic field on mixed convection heat transfer in the lid-driven at the top of a wavy enclosure filled by hybrid nanofluid (Fe3O4–MWCNT/water), has been studied by Maneengam et al. [Citation26]. The effect of the angle of magnetic on thermal performance is significant, where thermal performance is improved by the magnetic forces when it is in a perpendicular direction. Also, the results show that the number of undulations on the two-sided wavy wall enclosure can enhance the heat transfer rate. Lid-driven container filled by hybrid nanofluid of Al2O3-Cu/water at a 4% volume fraction studied by Maneengam et al. [Citation27]. Also, the effect of different shapes of obstacles on thermal activity was indicated, the results show that the Nusselt number increased to 15.54% at triangle obstacles. Biswas et al. [Citation28] investigated numerically the effect of MHD on free convection in an oblique enclosure filled with porous media saturated with Cu–Al2O3/water hybrid nanofluid with different volume fraction of nanoparticles, Da, Ra and Ha numbers. The findings indicated an increase in the undulation amplitude enhances the heat transfer. Mandal et al [Citation29] studying numerically the effect of MHD on heat transfer in complex enclosure filled by hybrid nanofluid Al2O3-Cu-H2O at different dimensionless parameters, (Ra), (Ha), (Da), and undulation amplitude (λ). The results show the increasing of the undulation's amplitude led to an increase the heating surface area. Mondal et al. [Citation30] showed that the partial magnetic fields with cavity inclination have a significant impact on the heat transport mechanism.
According to the above studies, the Inner hot pipe configurations refer to the placement, shape, and arrangement of the hot cylinder within the wavy-walled enclosure can affect the temperature distribution within the chamber and, consequently, the fluid flow. In the present study, the MHD TGC in a novel-shaped, wavily walled enclosure with convergent from the top and above sides with a corrugated hot cylinder will likely involve numerical simulations using the finite element technique for a multiple dimensionless parameters, such as Rayleigh number , Hartman number
, and nanofluid volume fraction
, Darcy number
, MHD inclination angle
, position of the corrugated cylinder
with respect to x-axis as shown in Figure and number undulations of corrugated cylinder
. The simulations can investigate the heat transfer and fluid flow characteristics beside to optimize the enclosure design and desirable cylinder configuration for specific applications. The applications of such selected geometry are on the heat exchanger industry as there is I-shaped enclosure that it stands for the heat exchanger. Additionally, we make the top and bottom of the enclosure to had a trapezoidal shape to study such a proposed design and locate the vortices within the enclosure. The major novelty is on examination of the MHD natural convection in such a complex shape of enclosure which is look like I-shaped with corrugated walls. Additionally, the bottom and top give the shape of trapezoidal enclosure with corrugated surfaces connected the both trapezoidal enclosure which increases the novelty points along with the inner corrugated porous cylinders.
2. Description and mathematical modelling
Figure is an illustration of the computational domain with its dimensions in (mm). The geometry involves corrugated cylinders inside a vertical enclosure with wavy walls at the right and left, while the bottom and top give the shape of the trapezoidal enclosure. The bottom and top walls of the enclosure are insulated, while the right and left are maintained at a cold temperature. In this study, assumed that there is one corrugated cylinder is heated and will be moved upward in a vertical direction. So, the effect location of heated corrugated cylinder on heat transfer enhancement will be study at three cases to show the suitable location of it. This cylinder will be porous, while the enclosure will be filled with a nanofluid. The Effect of the MHD inclination angle (γ) in the present work will be calculated at three values which are (00, 450 and 900). The enclosure filled by Al2O3/H2O. Table . includes the thermophysical properties of the pure fluid and the Al203/water nanofluid.
Table 1. Thermophysical properties of Nanoparticle and basic fluid at T = 250 C [Citation32].
The corrugated cylinder has been sketched by a parametric curve, which has the following equations: [Citation31]
(1)
(1)
(2)
(2) A is the amplitude, R is the radius of the outer circle and equal to 10 mm, N is the number of corrugations between 2 and 8. Also, the sinusoidal vertical walls have been sketched by using a parametric curve by using equations (3-4). [Citation6]
(3)
(3)
(4)
(4) A: amplitude of vertical wall equal to 0.08 and N: number of corrugations in vertical wall equal to 3. and S has been set with zero for a minimum value and set to 1 as the maximum value. The wavy enclosure is filled with an Al2O3/water nanofluid. The porous media in the other three cylinders is saturated with the same nanofluid. Under stable state conditions, the following main assumption is taken into consideration:
1- Fluid flow is assumed to be incompressible,
2- Laminar flow and Newtonian,
3- With no internal heat generation due to Darcy’s viscous dissipation,
4- Radiation effect is insignificant,
5- Nanoparticle is spherical and uniform in size.
6- Thermal and physical properties are constant.
7- Thermal equilibrium between the nanofluid and the porous media is considered.
8- It is presumed that the thermophysical parameters remain constant, except for the density which is influenced by temperature and handled using the Boussinesq approximation.
9- The Darcy-Brinkmann model is employed to simulate porous media,
3. Governing equation
The equations that govern the fluid flow and heat transfer can be written as follows [Citation33].
Continuity Equation
(5)
(5)
– Momentum Equation
(6)
(6)
– momentum Equation
(7)
(7) Energy Equation
(8)
(8) The non-dimensional governing equations were derived using dimensionless parameters. [Citation6]
(9)
(9) The nondimensional of the above equations can be written as follows:
Continuity equation:
(10)
(10) Momentum Equation
– momentum Equation
(11)
(11) Energy Equation
(12)
(12) The main coefficients that appear in non-dimensional governing equations are listed in Table
Table 2. Coefficients included in non-dimensional G.E.
In the following, the equations describing the nanofluid's thermophysical properties have been inserted [Citation34, Citation35]:
(13)
(13)
(14)
(14)
(15)
(15)
(16)
(16)
The following equations are inserted to determine the effectiveness of the thermal conductivity, keff, and the thermal diffusivity, :[Citation36]
(17)
(17) The nanofluid’s ability to conduct electricity can be guessed from [Citation36]
(18)
(18) The contours of streamlines have been depicted below;
(19)
(19) Following is the dimensionless formula for the stream function;
(20)
(20) Boundary conditions
Boundaries were used to simulate the inner hot corrugated cylinder as follows.
(21)
(21) The B.C.S. at the cold wavy wall sides are:
(22)
(22) The B.C.S. at the above and down walls of the encloser, which are insulated, are:
(23)
(23) To check the thermal behaviour Nusselt number must be calculated at the hot wall. The following equation is used to calculate average and local Nu: [Citation36]
(24)
(24)
4. Numerical analysis
The governing equations’ dimensionless form as shown in equations (10-12) with applying the boundary conditions shown in equations (21-23) are solved numerically by using COMSOL Multiphysics software based on FEM. The laminar flow (spf) was analyzed by solving continuity and momentum equations, as well as the heat transfer mode was analyzed by solving the energy equation Equation(12)(12)
(12) .
4.1. Mesh generation and dependency
The consuming time and accuracy of numerical results dependently on the number of elements where the appropriate number of elements must be determined. So, in the present work, the shape of mesh had been chosen as triangular type due to its simplicity and ability to cover all of the enclosure quickly as seen in Figure . On the other hand, the accuracy of results must be checked by choosing the dependent number of mesh that give an accurate result. Table shows effective of a number of elements on the Nusselt number which was chosen as a parameter to check the solution. It can be seen with the increases of a number of elements, the accuracy of Nusselt number had not affected. Hence there is no need to increases the number of elements. So, meshing with extra-fine grid used in the present work at residual error is set up to 10−6.
Table 3. Sensitivity of Grid generation on the dimensionless value of Nusselt number.
4.2. Validation of numerical solutions
The accuracy of software can be verification by validation the results of present work with significant researchers in terms of Nusselt number, stream lines etc. Table show the validation of average Nusselt number results of the present work with Malekpour et al. [Citation37] for a range of Ra number in I -shaped encloser filled by CuO – water nanofluid.
Table 4. Validation of Nuavg at φ = 0.04, Ha = 80 of the current study with Malekpour et al.[Citation37].
Also, another validation with number of researchers has been done for values of Ra number as shown in Figure . It can be seen from this figure and Table there is a good agreement between results.
Another validation has been verified in terms of streamlines for different porous layer thickness at Ra = 106, Da = 10−3, ϕ = 0.05. of present work with numerical results of Hussain and Rahomey [Citation38] as shown in Figure .
Figure 4. Validation in terms of streamlines of CFD results for present work and Hussain and Rahomey [Citation38].
![Figure 4. Validation in terms of streamlines of CFD results for present work and Hussain and Rahomey [Citation38].](/cms/asset/6bb2db3e-f461-4f6f-9218-65b5f20889bd/tusc_a_2335685_f0004_oc.jpg)
On another side models of both thermal conductivity and the dynamic viscosity properties that used in the present work validated among the experimental data in Figure (a) and (b). These validations perform a significant trust of the accuracy of the present numerical code.
Figure 5. (a) validation the dynamic viscosity ratio with experimental of Ho et al. [Citation39] (b) validation of thermal conductivity ratio with Chon et al. [Citation40].
![Figure 5. (a) validation the dynamic viscosity ratio with experimental of Ho et al. [Citation39] (b) validation of thermal conductivity ratio with Chon et al. [Citation40].](/cms/asset/95441d7d-af7a-472b-804b-a8b06838465f/tusc_a_2335685_f0005_oc.jpg)
5. Results and discussion
The following variables are presented and analyzed in this study: Rayleigh number (Ra), Hartman number (Ha), Darcy number (Da), and medium porosity (ε) for all results is kept at (0.4), and thermal conductivity ratio (knf / keff) 0.8776. Furthermore, the wavy porous cylinder's size, shape, position, and number of undulations of the corrugated cylinder also changed to study the effect of these parameters.
5.1. Effect of Rayleigh’s number
Figure shows the isothermal lines in enclosure at Ha = 60, Da = 10−3 and the corrugated cylinder at lower (δ = 0.3) at various Ra number. It can be shown from this figure that the increase in the Ra number leads to enhanced natural convection. As the Raleigh number increases, the convective heat transfer increase due to buoyancy-driven flows becomes more pronounced. Also, this figure showed that there are vortices near the corrugated cylinder in region that fully with nanofluid, while in the porous region there is no circulations this attributed that strength of fluid flow becomes lower in this region. Hence, with the increasing Ra number the isothermal lines exhibit stronger convective currents lead to increase the heat transfer rates which improved thermal performance of the system. This can be attributed to the buoyancy forces induce stronger fluid motion, causing temperature gradients to be steeper in certain regions.
The effect of the Ra number on stream function and streamlines has been shown in Figure . This figure shows that increasing the Ra number from 104 to 106 improves the maximum values of the stream function due to increase the magnitude of velocity. The increasing of velocity resulted from the enhancement that resulted in buoyancy force which can be generated by the variations of density, especially in the area close to the corrugated heated cylinder and cold walls. This can result in enhanced flow instabilities, such as forming eddies, vortices, and recirculation zones, as shown in case (c). The streamlines become curved, twisted, and irregular due to the intensified convective motion caused by higher buoyancy effects. The stream lines become more complex and exhibit rough shapes. This behaviour also relates to the strong coupling between the buoyancy-driven flow and the porous -nano-fluid mediums.
5.2. Effect of Hartman number
The Hartmann number is a dimensionless parameter that characterizes the ratio of magnetic forces to forces resulting from the viscosity resistance in a magnetohydrodynamic (MHD) flow. It is typically associated with conducting fluids in a magnetic field. Figure shows the effect of the Hartman number on the isothermal counter and stream function, which ranges from (0 to 60) at Ra = 106, and Da = 10−3. As stronger magnetic forces leads to increasing Ha number, suppressing the system's convective heat transfer. The magnetic field tends to suppress the fluid motion, reducing the formation of convective currents and impeding heat transfer from hot to cold regions. This figure shows the development of MHD Boundary Layers near the walls of corrugated cylinders with the Hartmann number increasing. The magnetic field's presence significantly influences the heat transfer within the boundary layers. The grown isothermal lines have been noticed in case (c). This can be attributed to two main reasons; firstly, the magnetic field interacts with temperature gradients, resulting in thermomagnetic convection, leading to localized the stream flow and improved natural convection heat transfer in the regions near the hot surface and spread towered above the enclosure. Secondly, a magnetic field can induce Joule heating in the conducting fluid. This is caused by the electrical current generated by the magnetic field and fluid interaction.
The effect of the Hartmann number on streamlines and stream functions is shown in Figure . It can be noticed from this figure that the increases in Ha number lead to a reduction in maximum value of stream function due to the magnetic field, which becomes stronger and exerts greater influence on the fluid. Also, the suppression of fluid motion takes place, and it can be noticed that there is a reduction of flow velocities and turbulence damping. The streamlines become smoother and more constrained due to the magnetic forces acting against the fluid motion. At Ha = 0, large vorticity appears, while increasing the Ha led to the formation of eddies, vortices, and recirculation zones. The magnetic field and fluid flow interplay can result in dynamic and irregular streamlines. In all figures, the behaviour of streamlines or isothermal lines becomes symmetric. This is attributed to the hot wall at the centre of the enclosure while the other side is cold.
5.3. Effect of inclination angle
The inclination angle of the magnetic field effect on isothermal lines is shown in Figure ; the values of the (γ) are taken equal to (0, 45, 90 deg) at Ha = 60, Da = 0.001, and Ra = 60. The orientation of the magnetic source at different angles (0°, 45°, 90°) in a system involving nanofluid-porous flow inside a corrugated cylinder can lead to varying effects on the isothermal lines. The interaction between the magnetic field, fluid flow, and porous medium results in different flow patterns and temperature distributions for each orientation. Case (a) represents the magnetic source is oriented at 0° (parallel to the fluid flow direction); it can be noticed from this figure that there is a minimal impact on the distribution of isothermal lines, where the max isothermal lines appear above the hot cylinder as parallel layers. When the magnetic source is oriented at 45° to the fluid flow direction case (b), it can notice additional complexity to the thermal behaviour. The altered streamline patterns can influence the convective heat transfer characteristics, changing the positioning and distribution of isothermal lines. Case (c) shows the effect of a magnetic source on isothermal lines when it is orientated at 90° (perpendicular to the fluid flow direction). The perpendicular magnetic field orientation can substantially impact the distribution of isothermal lines. The findings show that hotlines that have red colour toward the above layers of the heated cylinder at an angle of the magnetic field equal to zero degree (γ = 00), while penetrating the layer on the right at (γ = 450). The hotlines toward to left when the angle of the magnetic is equal to 900. In case(c), the enclosure coverage by the isothermal lines and distributed symmetrically on both sides of I- the wavy encloser. The altered streamline patterns induced by the Lorentz force can modify the convective heat transfer characteristics and significantly influence the positioning and distribution of isothermal lines.
Figure 10. The isothermal counter varies the magnetic source’s inclination angle (Ha = 60, Ra = 106, Da = 10−3, and δ = 0.3).

Figure 11. The stream function counter varies the magnetic source’s inclination angle (Ha = 60, Ra = 106, Da = 10−3 and δ = 0.3).

The influence of orientation of magnetic source on streamlines shows in Figure . It is noticed from this figure the max values of the stream function appear at (γ = 900). Case (a) shows that the magnetic field lines and fluid flow direction are aligned, resulting in minimal interaction between the magnetic field and the flow. Case (b) shows that the magnetic source is oriented at 45° to the fluid flow direction, which means the magnetic field lines and fluid flow direction are now at an angle. In this case, a significant deflection or distortion in the streamlines leads to altered flow patterns, which can be attributed to the Lorentz force that acts perpendicular to the magnetic field and fluid flow directions. When the magnetic source is oriented at 90° leads to a significant interaction between the magnetic field and the flow. Small eddies appear at 00 angle, and these eddies become larger and less number at (γ = 900).
5.4. Effect of Darcy’s number
The influence of changing the Da number on both isothermals has been indicated in the Figure . It can be seen from this figure the increasing Da leads to heat transfer enhancement where heat conduction through the porous media will mainly influence the temperature distribution and isothermal lines. The temperature gradients will follow the characteristics of the conduction process rather than the fluid flow patterns. It can be seen from case(a) that the region near the hot source has a higher isothermal line and decreased at Da = 10−5. Also, the high isothermal lines reached the top edges of the encloser while this reversed at low Da, where the lower values of the isothermal line appear at the leading edges of the encloser.
Figure shows the effect of Da on-stream functions; in the case of low Darcy numbers, viscous forces dominate the flow behaviour. The fluid flow in the porous media will be characterized by slow and highly damped flow patterns. The stream functions will reflect this behaviour, indicating a smooth and relatively slow fluid movement through the porous media. It is noticed that the max value of the stream function appears at high Da.
5.5. Effect of locations
The Effect of the heat source’s position has been shown in Figure at Ha = 60, Ra = 106, Da = 10−3. It can be seen from this figure that the isothermal lines fill the enclosure when the hot cylinder is at δ1 = 0.3. When the hot source is at δ2 = 0.8, it can be noticed that the isothermal lines don’t cover all the area in the encloser where it covers the entire area from δ2 = 0.8 to upper. Also, this trend reversed in case (c), where these isothermal lines cover the size above the hot cylinder at δ3 = 1.3 and effect on lower site, as shown in the blue line. This can be attributed to the convection transfer from a hot source to a cold start and due to the buoyancy force of fluid.
The influence of the hot source's location has been shown in Figure , Whereas the max stream function occurs in case a and less value in case (c). Hence, the influence of location is quite significant as it diminishes the fluid's flow intensity. Case (a) exhibits superior resistance to fluid flow in the internally heated corrugated pipe, followed by case (b), while case (c) demonstrates the lowest resistance.
5.6. Heat transfer enhancement
To check the heat transfer, the Nusselt number must be calculated because it indicates the heat transfer enhancements. The present section illustrates the effects of different parameters on heat transfer, for example, the volume fraction of nanoparticles, the position of the heated cylinder, Ha number, Ra number, Da, the inclination of MHD angle, and undulations number. The effect of hot inner corrugated cylinder localization at different volume concentrations of nanoparticles, Ha = 60, Ra = 106, and γ = 900 has been shown in Figure . It can be seen from this figure that the hot corrugated cylinder at a lower position (δ1=0.3) has a high average mean Nusselt number compared to other locations. This can be attributed to the Nusselt number is the function of temperature gradient and effective thermal conductivity concerning thermal conductivity to basic fluid. Also, it can be seen from this figure that the Nusselt number is decreased with increasing volume concertation of nanoparticles, which offers a different behaviour. In contrast, other studies show that the increase in volume concertation leads to a rise in Nu. This implies that the enhancement in thermal conductivity resulting from increasing the volume fraction of nanofluid “φ” surpasses the influence of inertia and viscous forces. In contrast, the Maxwell model exhibits distinct behaviour characterized by the detrimental effect of the parameter φ on (Nu). The observed adverse effect, namely the decrease in Nu with an increase in φ, indicates that at high Ra = 106, the combined influence of inertia and viscous forces outweighs the impact of thermal conductivity. As mentioned earlier, when the Rayleigh number (Ra) is less than this value, an increase in the parameter φ results in an increase in the Nusselt number (Nu).
Figure 16. Values of Nu interfaces with ϕ for different locations of the hot cylinder at Ha = 60, Ra = 106, and γ = 900.

The effect of the inclination angle of the magnetic field (MHD) on the Nusselt number is shown in the Figure . The increasing inclination angle to γ = 900 led to maximum heat transfer, while the heat transfer is less at both ranges, less than 900 and greater than 900. This also showed when the effect of inclination of MHD angle on isothermal lines explained where the lines of isothermal distribution are distributed symmetrically on all enclosers and coverage it.
Figure 17. Nu interfaces with γ, on the first cylinder at δ = 0.3, at different volume fractions Ra = 106 and Ha = 60, Da = 10−3.

The effect of Hartman on heat transfer is shown in Figure ; as shown in the previous section, the increase of Hartman leads to a decrease in the max stream function and less distribution to isothermal lines; here, the rise of Ha leads to a reduction in Nu.
Figure 18. Nu interfaces with Ha on the first cylinder at δ = 0.3, at a different angle of inclination, Ra = 106 and ϕ = 0.02, Da = 10−3.

On the other hand, increasing Ra and Da leads to high heat transfer, as indicated in Figures and , respectively. It can be inferred from these figures that increasing Ra; Da numbers enhance natural convection. As the Raleigh number increases, the convective heat transfer due to buoyancy-driven flows becomes more pronounced. Increasing of Rayleigh number leads to enhancing the fluid flow strength and this reflects on enhancement of heat transfer as the heat transfer at low Rayleigh number is takes place due to conductive mode while with increment of Rayleigh number into
the convection mode will be dominant. Additionally, increasing the Darcy number leads to increases the porosity of the porous medium leading to more nanofluid to penetrate through the entire domain transferring more heat. The isothermal lines exhibit stronger convective currents, increasing heat transfer rates.
Figure 19. Nu interfaces with volume fraction on the first cylinder at δ = 0.3, at different Ra, Ha = 60 and Da = 10−3,γ=p/2.

The Effect of the number of undulations on the first hot corrugated cylinder (δ = 0.3), Figure shows this Effect where the conditions are fixed at (Ra = 106, Ha = 30, Da = 10−3, ϕ=0.06, γ = 00), according to results obtained that increasing of several undulations lead to a slight decrease in Nusselt number.
Figure 21. Nu varies with the number of undulations of the hot corrugated cylinder at δ = 0.3 (Ra = 106, Ha = 30, Da = 10−3, ϕ=0.06, γ = 00).

The Effect of several undulations of the hot cylinder on the max stream function has been shown in Figure at (Ra = 106, Ha = 30, Da = 10−3, ϕ=0.06, γ = 45°). This Figure shows that the increasing undulations number of the corrugated cylinder from 2 to 4 led to a slight decrease in the maximum value of stream function, and then increased and reached 35.18 at N = 8; this can be attributed to the rise in the vorticities that formed about the enclosure.
Conclusions
MHD natural convection has been examinate in enclosure like I-wavy shaped with trapezoidal sides from top and bottom filled by AL2O3/ water nanofluid. The enclosure has corrugated cylinder from porous medium. In the present study the location (δ) of this cylinder is changed with respect to lower side of enclosure. The effects of many dimensionless parameters have been studied such as Ra, Ha, Da numbers, and angle of inclination of magnetic source, volume fraction of nanoparticle. The main conclusions obtained are as follows:
The using nanofluid AL2O3/ water nanofluid can increase the intensity of flow this can attributed to enhance the buoyancy force.
The performance of heat transfer by convection inside enclosure increased by increasing both of Da numbers, where hydrodynamic flow becomes stronger near heated wall this lead to increase Nusselt number from 8 to 18.67 with increasing of Da from 10−5–10−3.
The convection heat transfer inside the enclosure is reduced by the increase in the Hartmann number.
The variation of the magnetic field inclination angle has a distinct impact on the rate of heat transmission. It has been observed that as the angle increases from 0° to 90°, the Nusselt number decreases. The results indicate that the Nusselt number is equal to 15.34 at γ = 900, but at 0° it is 10.234.
Increasing of Rayleigh number leads to enhancing the fluid flow strength and this reflects on enhancement of heat transfer as the heat transfer at low Rayleigh number
is takes place due to conductive mode while with increment of Rayleigh number into
the convection mode will be dominant.
The increasing of Ra number lead to increase number of circulation in flow and this lead to enhance the heat transfer.
It can be seen that the decreasing number of undulations of inner corrugated cylinder reveal to best heat transfer. It can be seen that Nu = 15.9 at N = 2 and reaches 10.59 at = 8. That means the enclosure with smooth walls can enhance the heat transfer as compared to the internally heated corrugated cylinder.
Applying a magnetic field vertically enhances the strength of fluid flow.
There is a noticeable effect of the location of the hot corrugated cylinder on the natural convection heat transfer. The result proved that the inner cylinder at a low position led to high heat transfer compared to other sites.
The number of waves of the internal corrugated cylinder controls the circulation of heat transfer and fluid flow inside the enclosure
Acknowledgement
The authors extend their appreciation to the Deanship of Scientific Research at King Khalid University for funding this work through large group Research Project under grant number RGP. 2/7/44.
Disclosure statement
No potential conflict of interest was reported by the author(s).
Additional information
Funding
References
- Tayebi T, Chamkha AJ. Effects of various configurations of an inserted corrugated conductive cylinder on MHD natural convection in a hybrid nanofluid-filled square domain. J Therm Anal Calorim. 2021;143:1399–1411.
- Dogonchi AS, Armaghani T, Chamkha AJ, et al. Natural convection analysis in a cavity with an inclined elliptical heater subject to shape factor of nanoparticles and magnetic field. Arab J Sci Eng. 2019;44:7919–7931.
- El Mansouri A, Hasnaoui M, Amahmid A, et al. Numerical analysis of conjugate convection-conduction heat transfer in an air-filled cavity with a rhombus conducting block subjected to subdivision: Cooperating and opposing roles. Int J Heat Mass Transfer. 2020;150:119375.
- Parvin S, Roy NC, Saha LK. Magnetohydrodynamic natural convection of a hybrid nanofluid from a sinusoidal wavy cylinder placed in a curve-shaped cavity. AIP Adv. 2021;11(8).
- Mandal DK, Biswas N, Manna NK, et al. Thermo-fluidic transport process in a novel M-shaped cavity packed with non-Darcian porous medium and hybrid nanofluid: application of artificial neural network (ANN). Phys Fluids. 2022;34(3).
- Abdulkadhim A, Abed IM, Said NM. Computational investigation of magnetohydrodynamics convective heat transfer in I-shaped wavy enclosure considering various shapes of inner bodies filled with nanofluid–porous layers. Braz J Chem Eng. 2023;40(2):427–447.
- Biswas N, Mondal MK, Mandal DK, et al. A narrative loom of hybrid nanofluid-filled wavy walled tilted porous enclosure imposing a partially active magnetic field. Int J Mech Sci. 2022;217:107028.
- Hamza NH, Abdulrazzaq NM, Theeb MA, et al. The influence of magnetic field on entropy generation in a wavy cavity equipped with internal heated plate using Darcy-Brinkman-Forchheimer model. Int J Therm. 2023: 100463.
- Khanafer K, Vafai K, Lightstone M. Buoyancy-driven heat transfer enhancement in a two-dimensional enclosure utilizing nanofluids. Int J Heat Mass Transfer. 2003;46(19):3639–3653.
- Abu-Nada E, Chamkha AJ. Effect of nanofluid variable properties on natural convection in enclosures filled with a CuO–EG–water nanofluid. Int J Therm Sci. 2010;49(12):2339–2352.
- Rashad AM, Chamkha AJ, Ismael MA, et al. Magnetohydrodynamics natural convection in a triangular cavity filled with a Cu-Al2O3/water hybrid nanofluid with localized heating from below and internal heat generation. J Heat Transfer. 2018;140(7):072502.
- Abdulkadhim A. On simulation of the natural convection heat transfer between circular cylinder and an elliptical enclosure filled with nanofluid [part I: the effect of MHD and internal heat generation/absorption]. Math Model Eng Prob. 2019;6(4):599–610.
- Hatami M. Nanoparticles migration around the heated cylinder during the RSM optimization of a wavy-wall enclosure. Adv Powder Technol. 2017;28(3):890–899.
- Sheikholeslami M, Hayat T, Alsaedi A. On simulation of nanofluid radiation and natural convection in an enclosure with elliptical cylinders. Int J Heat Mass Transfer. 2017;115:981–991. doi:10.1016/j.ijheatmasstransfer.2017.07.119
- Abdelmalek Z, Tayebi T, Dogonchi AS, et al. Role of various configurations of a wavy circular heater on convective heat transfer within an enclosure filled with nanofluid. Int Commun Heat Mass Transfer. 2020;113:104525.
- Oztop HF, Abu-Nada E, Varol Y, et al. Natural convection in wavy enclosures with volumetric heat sources. Int J Therm Sci. 2011;50(4):502–514.
- Ma Y, Mohebbi R, Rashidi MM, et al. Nanoliquid thermal convection in I-shaped multiple-pipe heat exchanger under magnetic field influence. Physica A. 2020;550:124028.
- Kim BS, et al. A numerical study of natural convection in a square enclosure with a corrugated cylinderat different vertical locations. Int J Heat Mass Transfer. 2008;51(7-8):1888–1906.
- Hussein AK, Hussain SH. Heatline visualization of natural convection heat transfer in an inclined wavy cavities filled with nanofluids and subjected to a discrete isoflux heating from its left sidewall. Alexandria Eng J. 2016;55(1):169–186.
- Lee JM, Ha MY, Yoon HS. Natural convection in a square enclosure with a circular cylinder at different horizontal and diagonal locations. Int J Heat Mass Transfer. 2010;53(25-26):5905–5919.
- Choi C, Jeong S, Ha MY, et al. Effect of a circular cylinder’s location on natural convection in a rhombus enclosure. Int J Heat Mass Transfer. 2014;77:60–73.
- Massoudi MD, Ben Hamida MB. Enhancement of MHD radiative CNT-50% water+ 50% ethylene glycol nanoliquid performance in cooling an electronic heat sink featuring wavy fins. Waves Random Complex Media. 2022;32:1–26.
- Massoudi MD, Hamida MBB, Almeshaal MA, et al. The influence of multiple fins arrangement cases on heat sink efficiency of MHD MWCNT-water nanofluid within tilted T-shaped cavity packed with trapezoidal fins considering thermal emission impact. Int Commun Heat Mass Transfer. 2021;126:105468.
- Dhia Massoudi M, Ben Hamida MB, Mohammed HA, et al. MHD heat transfer in W-shaped inclined cavity containing a porous medium saturated with Ag/Al2O3 hybrid nanofluid in the presence of uniform heat generation/absorption. Energies. 2020;13(13):3457.
- Alshare A, Abderrahmane A, Guedri K, et al. Hydrothermal and entropy investigation of nanofluid natural convection in a lid-driven cavity concentric with an elliptical cavity with a wavy boundary heated from below. Nanomaterials. 2022;12(9):1392.
- Maneengam A, Bouzennada T, Abderrahmane A, et al. Numerical study of lid-driven hybrid nanofluid flow in a corrugated porous cavity in the presence of magnetic field. Nanomaterials. 2022;12(14):2390.
- Maneengam A, Laidoudi H, Abderrahmane A, et al. Entropy generation in 2D lid-driven porous container with the presence of obstacles of different shapes and under the influences of Buoyancy and Lorentz forces. Nanomaterials. 2022;12(13):2206.
- Biswas N, Mandal DK, Manna NK, et al. Magnetohydrodynamic thermal characteristics of water-based hybrid nanofluid-filled non-Darcian porous wavy enclosure: effect of undulation. Int J Numer Methods Heat Fluid Flow. 2022;32(5):1742–1777.
- Mandal DK, Biswas N, Manna NK, et al. Magneto-hydrothermal performance of hybrid nanofluid flow through a non-Darcian porous complex wavy enclosure. Eur Phys J Spec Top. 2022;231(13-14):2695–2712.
- Mondal MK, Biswas N, Mandal DK, et al. Assessment of thermal performance of hybrid nanofluid flow in a tilted porous enclosure by imposing partial magnetic fields. Waves Random Complex Media. 2022;32:1–34.
- Tayebi T, Dahmane F, Jamshed W, et al. Double-diffusive magneto-natural convection of nanofluid in an enclosure equipped with a wavy porous cylinder in the local thermal non-equilibrium situation. Case Stud Therm Eng. 2023;43:102785.
- Sheremet MA, Oztop HF, Pop I, et al. MHD free convection in a wavy open porous tall cavity filled with nanofluids under an effect of corner heater. Int J Heat Mass Transfer. 2016;103:955–964.
- Sheikholeslami M, Gorji-Bandpy M, Ganji DD, et al. Natural convection of nanofluids in an enclosure between a circular and a sinusoidal cylinder in the presence of magnetic field. Int Commun Heat Mass Transfer. 2012;39(9):1435–1443.
- Mahmoudi AH, Pop I, Shahi M. Effect of magnetic field on natural convection in a triangular enclosure filled with nanofluid. Int J Therm Sci. 2012;59:126–140.
- Ali FH, Hamzah HK, Egab K, et al. Non-Newtonian nanofluid natural convection in a U-shaped cavity under magnetic field. Int J Mech Sci. 2020;186:105887.
- Dogonchi AS, Sadeghi M, Ghodrat M, et al. Natural convection and entropy generation of a nanoliquid in a crown wavy cavity: effect of thermo-physical parameters and cavity shape. Case Stud Therm Eng. 2021;27:101208.
- Malekpour A, Karimi N, Mehdizadeh A. Magnetohydrodynamics, natural convection, and entropy generation of CuO–water nanofluid in an I-shape enclosure – a numerical study. J Therm Sci Eng Appl. 2018;10(6):061016.
- Hussain SH, Rahomey MS. Comparison of natural convection around a circular cylinder with different geometries of cylinders inside a square enclosure filled with Ag-nanofluid superposed porous-nanofluid layers. J Heat Transfer. 2019;141(2):1–12.
- Ho CJ, Liu WK, Chang YS, et al. Natural convection heat transfer of alumina-water nanofluid in vertical square enclosures: An experimental study. Int J Therm Sci. 2010;49(8):1345–1353.
- Chon CH, Kihm KD, Lee SP, et al. Empirical correlation finding the role of temperature and particle size for nanofluid (Al2O3) thermal conductivity enhancement. Appl Phys Lett. 2005;87(15):1–4.
- Fusegi T, Hyun JM, Kuwahara K, et al. A numerical study of three-dimensional natural convection in a differentially heated cubical enclosure. Int J Heat Mass Transfer. 1991;34(6):1543–1557.
- de Vahl Davis G. Natural convection of air in a square cavity: a bench mark numerical solution. Int J Numer Methods Fluids. 1983;3(3):249–264.
- Barakos G, Mitsoulis E, Assimacopoulos DO. Natural convection flow in a square cavity revisited: laminar and turbulent models with wall functions. Int J Numer Methods Fluids. 1994;18(7):695–719.