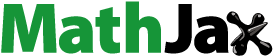
Abstract
Using a male ICRP voxel computational phantom, Monte Carlo simulations were performed to estimate the dose enhancement ratio (DER) in prostate radiation for 6 and 10 MV photon energy. A fully validated linear accelerator head was used, initially with a single beam to calculate the DER for different concentrations of nanogold mixed with prostate tissue. Each energy was tested with (FF) and without (FFF) a flattening filter. The DER was then calculated for a verified 10 MV treatment plan to mimic the real treatment scenario. The results showed that the DER increased with concentration, especially for FFF mode. In addition, the flattening filter-free enhancement ratio (FFFER) was investigated, from which it was found that a 6 MV FFF with a 40 mg/ml concentration enhanced the dose by a factor of 1.03 compared to 6 MV FF. For the entire plan, the difference between FFF and FF for both energies was approximately 2%.
1. Introduction
Due to the possibility of radiotoxicity to vital normal tissues surrounding tumours, prescribed doses in radiotherapy are limited. According to recent research, utilizing high-Z (high atomic number) nanoparticles as a radiosensitizer can increase the radiation dose to the tumour without increasing normal tissue exposure [Citation1–4]. Radiation dose enhancement of high-Z nanoparticles is an active area of research in radiotherapy [Citation5]. This is because high-Z nanoparticles accumulate more in tumours compared to normal tissue due to the elevated levels of glutathione present in malignant tissues [Citation6]. The primary nanoparticles being studied include iodine [Citation7], iron oxide (Fe2O3) [Citation8], platinum, gold, and silver [Citation9].
Among these nanoparticles, nanogold has shown promising results in terms of optimizing dosage deposition within the tumour site [Citation10–15]. Nanogold gained scientific interest because of its biocompatibility and low level of toxicity [Citation10,Citation16]. It was studied as a possible radiosensitizer for various tumour sites such as brain tumours, breast cancer, melanoma, colon cancer, cervical carcinoma, lymphoma, and prostate cancer [Citation17].
Several Monte Carlo (MC) studies have been carried out to investigate the radio sensitization effects of nanogold at different with kV or MV photon energies and for different forms of tumour [Citation3,Citation18–22]. In these MC studies for nanogold, a range of simulation configurations have been used such as using only single nanogold with different sizes, clusters of nanogold, or gold – water mixtures [Citation13,Citation23].
Most subsequent research with nanogold and low energy kV photons corroborated such promising results of dosage increase [Citation19,Citation24]. For brachytherapy also, researchers demonstrated good dose enhancement by employing nanogold, which is because brachytherapy involves the use of low photon energies of less than 0.5 MeV [Citation25–27]. Nanogold can boost the local radiation dosage deposited in tumour tissues with such low kV X-ray energies because the photoelectric effect is prominent for atoms with high atomic numbers irradiated with low X-ray energies [Citation28].
The literature on utilizing nanogold with MV photons indicates conflicting results between physical theoretical models and experiments. Experiments with MV photon have revealed radiosensitization of nanoparticles that are substantially greater than the increase in dose alone [Citation5,Citation17,Citation29,Citation30]. On the other hand, physical simulations revealed that KV energies had a larger dose enhancement ratio compared to MV energies. However, it has also been shown that photon beam quality have great impact on the photon interaction cross-section within the tumour if the nanogold was used as radiosensitizer [Citation31,Citation32]
It has recently been shown that removing the flattening filter (flattening filter free, FFF) from the linear accelerator can be useful in clinical practice [Citation33]. The main advantages are a significant increase in beam output and a reduction in head scatter as compared to flattening beams (flattening filter, FF) [Citation34]. Because the FFF beam has more low-energy photons in the kilovoltage range compared to FF beam [Citation35], it may be preferable to combine an FFF beam with nanogold to maximize the dose enhancement ratio [Citation20,Citation22].
In a 2017 MC study, Hwang et al. calculated the dose enhancement ratio (DER), which is the dose required with nanogold divided by the dose required without nanogold, for various nanoparticles such as gold, gadolinium iodine, and iron oxide with varying concentrations and diameters for 4, 6, 10, and 15 MV photon energies, as well as Co-60. The research was carried out on a human head phantom with a virtual tumour with a diameter of 5 cm which was defined in the centre of the brain parenchyma. This indicates that the phantom's depth is not excessive. The maximum concentration of nanogold with lower MV photon energy, such as 4 and 6 MV, with flattering filter offered the best dosage enhancement in their study [Citation18].
Martelli et al. [Citation20] studied dose enhancement for prostate radiation utilizing the EGSnrc Monte Carlo code to perform macroscopic Monte Carlo simulations. The research was carried out on a pelvic phantom with a prostate in the middle. The prostate material can be simply set to be water or nanoparticles mixed with water at various concentrations. Gold, platinum, iodine, silver, and iron oxide nanoparticles were used in the study at various concentrations, ranging from 3 to 40 mg/ml. Two clinical photon beams of 6 and 10 MV with and without a flattening filter were employed. It was found that 6 MV FFF with a higher concentration of nanoparticles led to a larger dose enhancement ratio [Citation20].
Spina et al. [Citation22] investigated the effect on dose enhancement of different nanogold concentrations for 6 and 10 MV FFF using Geant4 and EGSnrc-based BEAMnrc Monte Carlo algorithms. Their research used a heterogeneous phantom of a gold-water mixture and photon beams of 6 MV FF, 6 MV FFF, 10 MV FF, and 10 MV FFF with field sizes of 10 × 10 cm2. The dimensions of the phantoms were 40 × 40 × 40 cm3 with a voxel size equal to 1 × 1 × 1 cm3. The source-to-surface distance (SSD) was 100 cm. The dose enhancement ratio was estimated for several photon beams at various depths. The main findings revealed that the dose enhancement ratio was highest at shallow depth with a 6 MV FFF beam. This study highlighted the effect of the depth of the tumour on nanoparticle dose enhancement [Citation22].
In the current study, the effect of different concentrations of nanoparticles mixed with the prostate tissue of the ICRP reference computational male phantom on dose distribution will be investigated using the BEAMnrc/EGSnrc Monte Carlo code. This should lead to more realistic outcomes as the real prostate tissue composition will be used, which has not been investigated to date in the literature. Firstly, the DER of a single field with different photon energies, 6 and 10 MV, with and without flattening filter for different nanogold concentrations will be calculated and compared. The DER of 40 mg/ml of nanogold for a field-in-field prostate plan will then be calculated and compared for both energies.
2. Method
2.1 Monte Carlo beam model
Two validated Monte Carlo Elekta Synergy linac head models operating at photon energies of 6 and 10 MV were used in this study. The accelerator heads were modelled using the BEAMnrc/EGSnrc Monte Carlo code and validated by previous studies [Citation36,Citation37].
In the BEAMnrc code, directional bremsstrahlung splitting (DBS) was selected with a splitting number of 20,000 and a splitting field radius that varies according to the field size, as it should encompass the entire field. The rejection plane was defined as being 85 cm. In conjunction with DBS, bremsstrahlung cross-section enhancement (BCSE) was used where the enhancement constant and the enhancement power were set to 20 and 0, respectively. In addition, the range rejection technique was used, where 2 MeV was used as an ESAVE value. The cutoff energies of photons and electrons were set to 10 and 700 keV, respectively. In addition, these values were utilized as global defaults for the thresholds governing the production of secondary electrons and bremsstrahlung photons across all media. Therefore, all interactions below these energy thresholds were identified as soft collisions, resulting in the termination of particle histories and the localized deposition of all energy. All other simulation parameters were left at their default values.
2.2 ICRP phantom
In this study, an ICRP reference computational male phantom was used, which was created from real image data (Figure ) [Citation38]. The male voxel phantom is based on a whole-body clinical CT image set of a 38-year-old individual who suffered from leukemia. The computational phantom consists of 222 slices of 254 × 127 pixels with voxel volume of 2.137 × 2.137 × 8 mm3. It is made up of 141 segmented organs and 53 specified media.
The ICRP phantom was converted to the “egsphant” file format in order to be applicable with DOSXYZnrc user code. In addition, “pegs” files containing the cross-sections data for electron and photon interactions with respect to different media were generated. The atomic compositions of each media were created using the “EGS_gui” software based on information provided within the ICRP, 2009 report [Citation38]. Small modifications were applied in the DOSXYZnrc code to be able to use the ICRP “egsphant” phantom.
The same cutoff energies were used in the DOSXYZnrc code. Photon splitting, with splitting number of 35, was used. The EXACT and PRESTA-II algorithms were used as the boundary crossing and electron-step algorithm, respectively. The maximum fractional energy loss per step (ESTEPE) was 0.25 (25%). All other simulation parameters were left at their default values.
2.3 Single field comparison
The PEGS/EGSnrc code was used to generate the material data library of the nanogold particles for MC calculations. In this study, different concentrations of nanogold were mixed with the prostate tissue. These concentrations were 3, 7, 18, 30, and 40 mg/ml (by mass) and the mass densities were 1.0329, 1.0369, 1.0479, 1.0601 and 1.0705 g/cm3 respectively. These concentrations were chosen as stated by Hainfeld et al. [Citation10] and are based on small-animal experiments in nanoparticle-enhanced radiotherapy [Citation10].
The first part of this investigation was carried out using a single beam with a field size of 7.2 × 7.7 cm2. For each nanogold concentration, the single beam was simulated using two different energies, 6 and 10 MV, with and without flattening filter, in order to study the effects of energy and the presence of the flattening filter on the dose enhancement of nanogold. Consequently, the dose distribution was simulated, and the dose enhancement ratio (DER) was calculated using the formula below:
where
is the target dose with nanoparticle addition and
is the target dose without nanoparticle addition.
For each simulation, the dose distribution file (3ddose) was imported into MATLAB and the DER calculated and then compared. In addition, the effect on the dose enhancement when using FFF mode compared with FF mode was evaluated via the following formula:
where
is the DER with FFF at a dose point and
is the DER with FF at a dose point.
2.4 Field-in-Field plan comparison
Field-in-field plans with 6 and 10 MV photon energies were carried out and modelled using the DOSXYZnrc and BEAMnrc/Monte Carlo code, as shown Figure . The treatment plan settings from treatment planning software were converted to BEAMnrc input files. The modelled plan includes eight conformal fields: anterior (large open and small), left lateral (large 50° wedged, consisting of weighted open and 60° wedge, and small) and right lateral (large 50° wedged, consisting of weighted open and 60° wedge, and small). The prescribed dose was 74 Gy in 37 fractions over 7.4 weeks (2 Gy/fraction). All simulated beams were weighted and summed using MATLAB to obtain the final complete plan. These simulations were repeated when the prostate media was replaced with a nanogold-prostate tissue mixture at a concentration of 40 mg/ml. Then DERs were calculated for both plans and compared. Finally, the FFFER was calculated.
Figure 2. Transverse slice showing the prostate of the ICRP computational male phantom (left), and showing the complete treatment 10 MV prostate plan (right) (statistical uncertainty ≤1).

All the simulations were performed on a Linux Ubuntu PC with a 3.4 GHz AMD 9 5950X 16-processor CPU with hyperthreading and 32 Gb of RAM. Each simulation was split into 32 parallel jobs, distributing the simulation over 16 processors. A different number of histories for each simulation was used to achieve a statistical uncertainty of equal to or less than 1% at the region of interest.
3. Results
The current study on enhancing nanoparticle doses employs a computational phantom to replicate the real scenarios in radiotherapy treatment. By using the computational phantom alongside a full linear accelerator head, the investigation becomes extremely close to the real-world situation, and can thus be used to predict the benefit of using nanoparticles in radiotherapy.
3.1 Single field
Figures and show the DERs for different concentrations of nanogold for 6 and 10 MV, respectively, with and without flattening filter. As a result, the DER increases with nanogold concentration for both energies. The increase in absorbed dose, consequently DER, with rising concentration is attributed to the increased mass energy absorption coefficient for low-energy photons. For instance, at 300 keV photons, the mass energy absorption coefficient of prostate tissue increased by approximately 1.9% and 25% when mixed with nanogold concentrations of 3 and 40 mg/ml, respectively. The maximum DERs were found when a 40 mg/ml of nanogold was mixed with prostate tissue, which were 1.049, 1.078, 1.04, and 1.06 for 6 MV FF, 6 MV FFF, 10 MV FF, and 10 MV FFF, respectively. It can be clearly seen that the FFF beam showed a better dose enchantment and increases in DER by 2.8% and 1.9% when using photon beam energies of 6 and 10 MV, respectively. Therefore, the 6 MV FFF energy showed the optimal dose enhancement.
Figure 3. Dose enhancemnt ratio for different concnetraions of nanogold with 6 MV with (FF) and without (FFF) flattening filter (statistical uncertainty ).

Figure 4. Dose enhancemnt ratio for different concnetraions of nanogold with 10 MV with (FF) and without (FFF) flattening filter (statistical uncertainty ).

The current result is in agreement with previous studies [Citation3,Citation20,Citation22]. The reason behind this observation is due to the fact that the mean photon energy of 6 MV is lower than 10 MV, and consequently the probability of photoelectric interaction with material with high atomic number is greater, leading to increased dose deposition. In addition, removing the flattening filter would decrease the mean energy due to the increase in photon population with low energy, therefore increasing the dose enhancement.
Compared to Martelli’s 2020 study [Citation20], the results showed higher dose enhancement for the same energies with and without FFF. Even though the study was performed using a pelvic phantom with a prostate, it used water and nanogold-water mixtures. It was found that the optimal dose enhancement was achieved when 6 MV FFF was used. Smaller prostate size, smaller pelvic size, and a higher nanoparticle concentration also showed improved a dose enhancement ratio. For a 25 cm pelvic size and a concentration of 40 mg/ml, which give prostate depth of approximately 15 cm, the DERs were 1.09, 1.15, 1.04, and 1.08 for 6 MV FF, 6 MV FFF, 10 MV FF, and 10 MV FFF, respectively, as shown in Table [Citation20]. A study performed by Spina in 2022 [Citation22] also showed similar results to those achieved in this study for a 40 mg/ml concentration in the phantom for various depths in nanogold-water mixture media. The DERs at a 15 cm depth (which is comparable to the prostate depth in the ICRP phantom from the anterior) were 1.0624, 1.0972, 1.0316, and 1.0525 for 6MV FF, 6 MV FFF, 10 MV FF, and 10 MV FFF, respectively, as shown in Table [Citation22]. In these studies, the nanogold concentration was assumed to be evenly distributed within the tissue, similar to the current study.
Table 1. Comparison of the results of this study with previous studies.
In addition, the FFFER was calculated for each nanogold concentration with 6 and 10 MV, as shown in Figure . It is clear from this figure that the FFFER increases slightly with concentration. For 6 MV, the maximum FFFER was found to be 1.027 when a concentration of 40 mg/ml was used, while for 10 MV the maximum FFFER was 1.021. This indicates that removing the flattening filter increases the dose enhancement more significantly for 6 MV than for 10 MV, which is in agreement with previous studies [Citation22].
3.2. Investigation with complete 10 MV plan
Figure shows the entire treatment plan on the ICRP phantom with and without 40 mg/ml nanogold mixed with prostate tissue using 6 MV with FF. The DER for the complete 10 MV FF plan was 1.0362 and for 10 MV FFF was 1.055, as shown in Table . The slight decrease in the DER without flattening filter, for 10 MV, compared with the single field (1.0575) may be due to the different depths of the prostate relative to the different incident beam angles (as shown in Figure ). Spina et al. [Citation22] showed that DER decreases with depth [Citation22]. As a result, such a situation would affect the DER due to the differences in the attenuation of low-energy photons with different depths varies from 15 to 26 cm. The same observation was made when a 6 MV photon energy without flattening filter (1.077) was used. Consequently, the FFFER values were affected. However, even in a complete plan, the FFFER of the 6 MV photon beam was higher than that of the 10 MV.
Figure 6. The entire treatment plan (a) with and (b) without 40 mg/ml nanogold mixed with prostate tissue using 6 MV with FF.

Table 2. The DER for the entire plan for 6 and 10 MV with and without flattening filter with a 40 mg/ml nanogold concentration.
4. Conclusion
The current study has investigated the effects of nanoparticles with different concentrations mixed with the prostate tissue of an ICRP reference computational male phantom on dose distribution using the BEAMnrc/EGSnrc Monte Carlo code. This demonstrated more realistic outcomes as the real prostate tissue composition was used. Two different photon energies, 6 and 10 MV, were used for a single field and a complete plan. The greatest dose enhancement was found when a 40 mg/ml concentration of nanogold mixed with prostate tissue was irradiated via a 6 MV photon beam without a flattening filter. As a result, the tumour dose from a whole prostate radiotherapy treatment plan could be enhanced using nanogold particles whilst operating at a lower energy. The current approach of treating prostate cases could result in better outcomes with regard to patient treatment and help nanogold-enhanced radiotherapy.
Acknowledgment
The authors gratefully acknowledge the help from staff of the Department of Medical Physics and Clinical Engineering, SBUHB (Swansea Bay University Health Board) for their assistance in this study.
Disclosure statement
No potential conflict of interest was reported by the author(s).
References
- Herold DM, Das IJ, Stobbe CC, et al. Gold microspheres: a selective technique for producing biologically effective dose enhancement. Int J Radiat Biol. 2000 Jan 1;76(10):1357–1364. doi:10.1080/09553000050151637
- Regulla D, Schmid E, Friedland W, et al. Enhanced values of the RBE and H ratio for cytogenetic effects induced by secondary electrons from an X-irradiated gold surface. Radiat Res. 2002 Oct;158(4):505–515. doi:10.1667/0033-7587(2002)158[0505:EVOTRA]2.0.CO;2
- Robar JL, Martin MA, Riccio SA. Tumour dose enhancement using modified megavoltage photon beams and contrast media. Phys Med Biol. 2002 Jul;47(14):2433–2449. doi:10.1088/0031-9155/47/14/305
- Luchette M, Korideck H, Makrigiorgos M, et al. Radiation dose enhancement of gadolinium-based AGuIX nanoparticles on HeLa cells. Nanomedicine Nanotechnol Biol Med. 2014 Nov;10(8):1751–1755. doi:10.1016/j.nano.2014.06.004
- Rosa S, Connolly C, Schettino G, et al. Biological mechanisms of gold nanoparticle radiosensitization. Cancer Nanotechnol. 2017;8(1):2. doi:10.1186/s12645-017-0026-0
- Hepel M, Magdalena S.. Detection of oxidative stress biomarkers using functional gold nanoparticles. In: Matijević E, editor. Fine particles in medicine and pharmacy. Boston, MA: Springer; 2012. p. 241–281.
- Mansouri M, Shahbazi-Gahrouei D. A review on theranostic applications of iodine nanoparticles: recent findings and perspectives. Nanomedicine J. 2021 Sep 30;8:234–240.
- Hauser AK, Mitov MI, Daley EF, et al. Targeted iron oxide nanoparticles for the enhancement of radiation therapy. Biomaterials 2016 Oct;105:127–135. doi:10.1016/j.biomaterials.2016.07.032
- Yamada M, Foote M, Prow TW. Therapeutic gold, silver, and platinum nanoparticles. WIREs Nanomedicine Nanobiotechnology. 2015;7(3):428–445. doi:10.1002/wnan.1322
- Hainfeld JF, Slatkin DN, Smilowitz HM. The use of gold nanoparticles to enhance radiotherapy in mice. Phys Med Biol. 2004 Sep 21;49(18):N309–N315. doi:10.1088/0031-9155/49/18/N03
- Shukla R, Bansal V, Chaudhary M, et al. Biocompatibility of gold nanoparticles and their endocytotic fate inside the cellular compartment: a microscopic overview. Langmuir. 2005 Nov 1;21(23):10644–10654. doi:10.1021/la0513712
- Hainfeld JF, Dilmanian FA, Zhong Z, et al. Gold nanoparticles enhance the radiation therapy of a murine squamous cell carcinoma. Phys Med Biol. 2010 Jun 7;55(11):3045–3059. doi:10.1088/0031-9155/55/11/004
- Vlastou E, Diamantopoulos S, Efstathopoulos EP. Monte Carlo studies in gold nanoparticles enhanced radiotherapy: the impact of modelled parameters in dose enhancement. Phys Medica PM Int J Devoted Appl Phys Med Biol Off J Ital Assoc Biomed Phys AIFB. 2020 Dec;80:57–64.
- Chow JC. Chapter 2: Monte Carlo nanodosimetry in gold nanoparticle-enhanced radiotherapy. In: Chan MF, editor. Recent advancements and applications in dosimetry. New York, NY, USA: Nova Science Publishers; 2018. p. 1700–1738.
- Chow JC. Chapter 15: dose enhancement effect in radiotherapy: adding gold nanoparticle to tumour in cancer treatment. In: Ficai A, Grumezescu AM, editors. Nanostructures for cancer therapy; Amsterdam, The Netherlands: Elsevier; 2017. p. 383–403.
- Lasagna-Reeves C, Gonzalez-Romero D, Barria MA, et al. Bioaccumulation and toxicity of gold nanoparticles after repeated administration in mice. Biochem Biophys Res Commun. 2010 Mar 19;393(4):649–655. doi:10.1016/j.bbrc.2010.02.046
- Jain S, Coulter JA, Hounsell AR, et al. Cell-specific radiosensitization by gold nanoparticles at megavoltage radiation energies. Int J Radiat Oncol Biol Phys. 2011 Feb 1;79(2):531–539. doi:10.1016/j.ijrobp.2010.08.044
- Hwang C, Kim JM, Kim J. Influence of concentration, nanoparticle size, beam energy, and material on dose enhancement in radiation therapy. J Radiat Res (Tokyo). 2017 Jul;58(4):405–411. doi:10.1093/jrr/rrx009
- Sheeraz Z, Chow JCL. Evaluation of dose enhancement with gold nanoparticles in kilovoltage radiotherapy using the new EGS geometry library in Monte Carlo simulation. AIMS Biophys 2021;8(4):337–345. doi:10.3934/biophy.2021027
- Martelli S, Chow JCL. Dose enhancement for the flattening-filter-free and flattening-filter photon beams in nanoparticle-enhanced radiotherapy: a Monte Carlo phantom study. Nanomater Basel Switz. 2020 Mar 29;10(4):637. doi:10.3390/nano10040637
- Chow JCL. Depth dose enhancement on flattening-filter-free photon beam: a Monte Carlo study in nanoparticle-enhanced radiotherapy. Appl Sci. 2020 Jan;10(20):7052. doi:10.3390/app10207052
- Spina A, Chow JCL. Dosimetric impact on the flattening filter and addition of gold nanoparticles in radiotherapy: a Monte Carlo study on depth dose using the 6 and 10 MV FFF photon beams. Mater Basel Switz. 2022 Oct 15;15(20):7194.
- Zygmanski P, Sajo E. Nanoscale radiation transport and clinical beam modeling for gold nanoparticle dose enhanced radiotherapy (GNPT) using X-rays. Br J Radiol. 2016 Mar;89(1059):20150200. doi:10.1259/bjr.20150200
- Heuvel Van den F, Locquet JP, Nuyts S. Beam energy considerations for gold nano-particle enhanced radiation treatment. Phys Med Biol. 2010 Jul;55(16):4509. doi:10.1088/0031-9155/55/16/S06
- Bahreyni Toossi MT, Ghorbani M, Mehrpouyan M, et al. A Monte Carlo study on tissue dose enhancement in brachytherapy: a comparison between gadolinium and gold nanoparticles. Australas Phys Eng Sci Med. 2012 Jun 1;35(2):177–185. doi:10.1007/s13246-012-0143-3
- Ghorbani M, Pakravan D, Bakhshabadi M, et al. Dose enhancement in brachytherapy in the presence of gold nanoparticles: a Monte Carlo study on the size of gold nanoparticles and method of modelling. Nukleonika. 2012;57(3):401–406.
- Yoon M, Cho S, Jeong JH, et al. Monte Carlo simulation study on dose enhancement by gold nanoparticles in brachytherapy. J Korean Phys Soc. 2010 Jun 15;56(6):1754–1758. doi:10.3938/jkps.56.1754
- Butterworth KT, McMahon SJ, Currell FJ, et al. Physical basis and biological mechanisms of gold nanoparticle radiosensitization. Nanoscale 2012 Aug 21;4(16):4830–4838. doi:10.1039/c2nr31227a
- Yang C, Bromma K, Ciano-Oliveira D, et al. Gold nanoparticle mediated combined cancer therapy. Cancer Nanotechnol. 2018 Dec;9(1):4. doi:10.1186/s12645-018-0039-3
- Chithrani DB, Jelveh S, Jalali F, et al. Gold nanoparticles as radiation sensitizers in cancer therapy. Radiat Res. 2010 Jun;173(6):719–728. doi:10.1667/RR1984.1
- Tsiamas P, Liu B, Cifter F, et al. Impact of beam quality on megavoltage radiotherapy treatment techniques utilizing gold nanoparticles for dose enhancement. Phys Med Biol. 2013 Feb 7;58(3):451–464. doi:10.1088/0031-9155/58/3/451
- Berbeco RI, Detappe A, Tsiamas P, et al. Low Z target switching to increase tumor endothelial cell dose enhancement during gold nanoparticle-aided radiation therapy. Med Phys. 2016 Jan;43(1):436. doi:10.1118/1.4938410
- Xiao Y, Kry SF, Popple R, et al. Flattening filter-free accelerators: a report from the AAPM therapy emerging technology assessment work group. J Appl Clin Med Phys. 2015;16(3):12–29. doi:10.1120/jacmp.v16i3.5219
- Kragl G, Baier F, Lutz S, et al. Flattening filter free beams in SBRT and IMRT: dosimetric assessment of peripheral doses. Z Für Med Phys. 2011 May 1;21(2):91–101. doi:10.1016/j.zemedi.2010.07.003
- Georg D, Knöös T, McClean B. Current status and future perspective of flattening filter free photon beams. Med Phys. 2011 Mar;38(3):1280–1293. doi:10.1118/1.3554643
- Al-Saleh WM, Hugtenburg RP. Monte Carlo modelling of a 6 MV Elekta linear accelerator for in-field and out-of-field dosimetry. Radiat Phys Chem. 2023 Feb 1;203:110584. doi:10.1016/j.radphyschem.2022.110584
- Almatani T. Validation of a 10 MV photon beam Elekta Synergy linear accelerator using the BEAMnrc MC code. J King Saud Univ – Sci. 2021 Jun 1;33(4):101406. doi:10.1016/j.jksus.2021.101406
- ICRP. Adult reference computational phantoms. ICRP Publication 110. Ann. ICRP 39; 2009.