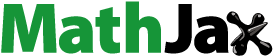
Abstract
A unique nanocomposite GO/AlCrO3/Mn3O4/SiO2/SnO2 synthesized through hydrothermal method serves as an electrode material for electrochemical supercapacitors. The material structural and optical characteristics were studied using various techniques. Interestingly, the average crystallite sizes were 16.88, 21.2, 20.81 nm for AlCrO3, GO, and GO@AlCrO3@SiO2@Mn3O4@SnO2 nanocomposite, respectively. The nanocomposite displayed 16 nm spherical grains due to particle aggregation on the graphene oxide surface causing structural deviations evident in the Raman spectrum slope around 1578 cm−1. FTIR spectra revealed distinctive bands at 2097 cm−1 and 1523 cm−1 indicating metal–oxygen bonds. UV-Vis spectra disclosed a band gap of 2.85 eV and a zeta potential of 27.9 mV signifying stability. The hybrid electrode exhibited remarkable electrochemical performance boasting a specific capacitance of 2495 Fg−1 for scan rate of 10 mVs−1. Given its robust performance under high current densities, enduring stability and energy efficiency the material holds significant promise as an energy storage material particularly for supercapacitors.
1. Introduction
Due to the surging demand for electronic devices, energy storage has become a crucial focus alongside energy conversion [Citation1]. With the need for alternative energy sources and higher energy consumption, improving energy storage technologies is vital given their dual optimization and high-power density. Supercapacitors, known for their impressive charge/discharge rates and long-lasting power have emerged as a significant solution [Citation2,Citation3]. These devices bridge the gap between rechargeable batteries and electrolytic capacitors due to their superior capacitance values. To achieve cost-effective high energy storage, environmentally friendly transition metal oxides with abundant availability and strong redox activity have been explored [Citation4]. In recent times efficient oxide spinel-based materials have been synthesized for the supercapacitor applications [Citation5–9]. The nano-flower and nano-plates like structures has also gained high attention for the supercapacitor applications [Citation10].
Utilizing two-dimensional (2D) structures, extensive electronic diversity have been achieved. In light of escalating ecological concerns and the need for clean water and efficient energy storage, advanced energy storage devices with high energy and power density and prolonged cycle life have gained worldwide significance. Particularly in 2D edge environments, massive materials exhibit distinctive physical and chemical properties tied to electron and phonon confinement effects [Citation11].
Graphene oxide characterized by its 2D nanostructure, exceptional electrical properties and large surface area has emerged as a prominent material in various applications. Its potential spans gas absorption, transistors, lithium-ion batteries, supercapacitors, lithium-air batteries, and drug delivery, garnering global attention. Introducing nanocrystals between layers of graphene oxide nanosheets enhances electrochemical characteristics making graphene oxide a compelling subject of research despite its relatively short history [Citation12,Citation13]. As a palpable semiconductor with substantial surface area, charge mobility, optical transparency, mechanical strength, thermal conductivity, and electrical conductivity graphene oxide holds promise [Citation14,Citation15] (Figure ).
ABO3-type perovskites, a class of compounds with diverse structures, have garnered interest [Citation16]. Investigating chemical interactions between aluminium and chromium nitrate has revealed reaction products formed at varying temperatures [Citation17,Citation18]. Amid extensive research on chromium-aluminium compounds and their physicochemical properties, interactions between chromium nitrates and aluminium have been explored [Citation19,Citation20]. Anticipating the need for sustainable energy, catalysts like tri-manganese tetroxide (Mn3O4) play roles in methane and carbon monoxide oxidation and nitrobenzene reduction. Manganese oxides serve in energy storage, catalysis, sensing, and electrodes [Citation21–23]. Mn3O4, an efficient and cost-effective catalyst, stands out [Citation24,Citation25]. SnO2 with its theoretical capacity of 782.0 mAhg−1 holds potential as a lithium-ion battery electrode material but its bulk counterparts face capacity degradation [Citation26,Citation27]. Nanostructured SnO2 materials dispersed on graphene nanosheets using various methods exhibit improved capacity and stability [Citation28]. Silicon dioxide nanoparticles valued for biomedical applications are functionalized due to their consistency and low toxicity [Citation29–31].
Addressing graphene oxide aggregation challenges AlCrO3, Mn3O4 and Na2SnO3.3H2O are introduced onto graphene oxide sheets forming a stable 3D conductive network and hydrogen bonding configuration [Citation32,Citation33]. Electrochemical investigations confirm the potential of the synthesized multinary composite as a high-performance electrode for supercapacitors [Citation34,Citation35]. While the GO/multinary nanocomposite shows promising performance in energy storage and water treatment detailed studies on chemical reactions between chromium carbonate and aluminium nitrate at elevated temperatures are lacking [Citation36–38]. The research introduces a novel multinary nanocomposite composed of graphene oxide (GO)/AlCrO3/SiO2/Mn3O4/SnO2 representing a distinctive combination that offers unprecedented advantages in the realm of energy storage. This unique composite structure harnesses the synergistic effects of multiple materials potentially enhancing electrochemical performance for energy storage systems such as increased capacity and cycling stability. The significance of this work lies in its ability to address specific challenges within the energy storage field and contribute to the advancement of energy storage technologies positioning it as a noteworthy addition to the ongoing research in this domain.
2. Preparation methods
2.1. Synthesis of aluminium perovskite
To initiate the synthesis, combine 3.7 grams of aluminium nitrate and 7 grams of chromium nitrate in 50 ml of ethanol. The aluminium and chromium nitrates dissolve in the ethanol solution. After a period of time, introduce an appropriate quantity of citric acid into the mixture while continuously stirring. Continue to heat and stir the solution consistently for around 2 h until a gel-like substance is formed. Subsequently, dry the gel at a temperature of 75 ˚C. Then, subject it to annealing for a duration of 6 h in an oven at varying temperatures ranging from 600 ˚C to 1100 ˚C. Once this process is complete, grind the resultant material using an agate mortar. Store the ground powder in sample bottles for subsequent characterization [Citation39].
2.2. Synthesis of trimanganese tetroxide
Commence the synthesis by combining 7 grams of chromium nitrate and 2.87 grams of manganese oxide within a beaker containing 50 ml of de-ionized water. Apply heat to the solution on a hot plate, maintaining a temperature range of 40–50 ˚C, and sustain continuous stirring for a duration of 20 min. Once homogeneity is achieved, gradually introduce 20 ml of ethanol into the mixture as a chelating agent. Subsequently, initiate the evapouration process by elevating the temperature to a range of 60–70 ˚C while consistently stirring until a gel with the desired brown hue forms. Proceed to desiccate the gel in a DHG-9202 oven at 105 ˚C for a period of 2 h. Following thorough desiccation, a brown powder is obtained. To further enhance the material, subject the Mn (NO3)2 powder to sintering in a VULCAN-D550 furnace at 800 ˚C for 3 h. Ultimately, utilize an agate motor to grind the sample into a finely powdered state [Citation40].
2.3. Synthesis of graphene oxide
Graphene oxide (GO) is typically produced from pure graphite powder using a modified version of Hummer’s approach [Citation41,Citation42].
2.4. Preparation of GO@AlCrO3 @SiO@ Mn3O4@SnO2 nanocomposite
Graphene Oxide (GO) is synthesized using natural graphite flakes following the methodology described in the literature. To create GO@AlCrO3@SiO2@Mn3O4@SnO2, the process involves introducing GO into a reduction procedure alongside AlCrO3 and diethylene glycol (DEG) [Citation43,Citation44]. Moving on, the next phase involves combining the prepared GO@AlCrO3@Mn3O4 composite with 40 mL of ethanol, 10 mL of water, and 5 mL of ammonia as per the as-prepared form (1 mL). Subsequently, 0.2 mL of tetraethyl orthosilicate (TEOS) is meticulously added drop by drop, and the reaction continues for a duration of 10 h under constant stirring. Following this, the dispersed GO@AlCrO3@SiO2@SnO2 composite is introduced into a solution comprising 60 mL of ethanol/water, with ethanol making up 37.5% by volume. In this solution, 0.2 g of sodium tin oxide (Na2SnO3·3H2O) and 0.36 g of urea are added while stirring. The amalgamated solution undergoes 10 min of continuous stirring before being transferred to a Teflon-lined stainless-steel autoclave. Subsequently, the autoclave is heated to a temperature of 200 °C and maintained at this temperature for a duration of 24 h. Upon completion of the reaction, the final product undergoes a sequence of procedures. It is subjected to four rounds of washing with ethanol and subsequently undergoes vacuum drying for 12 h at a temperature of 60 °C [Citation45].
2.5. Preparation of working electrode
1 milliliter of ethanol is employed to dissolve 0.25 mg of the nanocomposite material. The mixture is subsequently subjected to sonication for a duration of 20 min. The resulting solution is then delicately deposited onto a glassy carbon electrode using a micropipette. The electrode with the solution is allowed to air-dry for approximately 15 min. Following this, the prepared electrode is subjected to vacuum drying for a period of 20 min at room temperature. To act as a binder, a small droplet of Nafion solution is applied. The proportion of the binder in relation to the total mass of the active substance is 5%. After the vacuum drying process, the overall mass of the active ingredient on the electrode is 0.25 mg/cm2.
3. Instrumentation
For X-ray diffraction investigation, Brucker D-8 X-ray diffractometer equipped with a 40 VK X-ray source operating at a current of 40 mA and a step size of 0.02 was employed. Morphological and elemental analyses were conducted using the 20 kV TESCAN VEGA 3. Raman spectroscopy was performed by the high-resolution DONGWO OPTRON system from Korea, using 532 nm wavelength with a power output of 150 mW.
Cyclic voltammetry, galvanostatic charge–discharge, and electrochemical impedance spectroscopy within the frequency range of 0.1 Hz to 1 MHz were conducted using the Gamry Potentiostat Interface 1000. The reference, counter and working electrodes employed were silver chloride (AgCl), silver wire and modified glass carbon electrode (GCE) respectively. The electrolyte used was 1M KOH and the results were recorded using a DELL laptop. For the electrochemical calculation of the active surface area at a scan rate of 100 mV s−1 a standard redox solution was employed.
4. Results and discussion
4.1. XRD analysis
In Figure the X-ray diffraction patterns of the cleaned and dried samples are presented. Notably, the presence of oxygen-based functional groups contributes to a distinct peak at 2θ = 11.5° in GO, corresponding to an interlayer spacing of 0.94 nm between the GO layers (JCPDS NO.75-2078). Figure (a) displays diffraction peaks at 2θ values of 20.37°, 24.94°, 34.15°, 36.84°, 42.15°, 51.10°, 55.70°, 59.24°, 66.15°, 74.54°, and 78.23°. These peaks align well with the (200), (211), (220), (321), (330), (510), (521), (530), (600), and (611) space groups, indicating a strong correspondence to the hexagonal AlCrO3 with the p63/mmc space group (JCPDS card No.00-021-109). The diffraction pattern signifies the hexagonal structure of the material, with 2θ values consistent with those expected for AlCrO3. In Figure (b) the GO@AlCrO3@SiO2@Mn3O4@SnO2 nanocomposite demonstrates diffraction peaks at 2θ values of 26.28°, 27.65°, 30.05°, 31.60°, 35.44°, 36.62°, 37.95°, 41.04°, 44.35°, 53.80°, 56.08°, 62.3°, and 65.76°. These peaks are all indexed as cubic. Additionally, the absence of other discernible peaks indicates that the SiO2 in AlCrO3@SiO2 is in an amorphous state. The diamond-shaped peaks observed in GO@AlCrO3@SiO2@Mn3O4@SnO2 can be attributed to the (211), (200), and (220) reflections of hexagonal AlCrO3 nanocrystals. Meanwhile, the broader peak around 11.5° corresponds to GO. Peaks corresponding to SnO2 and SiO2 are less conspicuous due to their lower concentration and amorphous nature within the material [Citation46]. To explain this variance in lattice parameter values, we must look at the discrepancy in the size of ionic radii between the three metal ions, Mn + 2, Al + 3 and Si + 2 [Citation47]. If the spinel crystal lattice parameter is affected by a new phase of Al when it comes to the dielectric constant, AlCrO3 has a higher value than Mn3O4. Each sample is determined using Scherer equation, and the results are displayed in Table for each diffraction peak [Citation48]. According to this study, the crystallite sizes of multinary metal oxides were smaller than those of metal oxides [Citation49].
Table 1. Lattice parameters, particle, crystalline size and strain values of compound.
Figure depicts the results of a Williamson-Hall (WH) analysis of the full width at half maximum (FWHM) (β) of different Bragg peaks used to determine the influence of strain. The coordinates of the y-axis are and the x-axis is
. Each sample strain is calculated from the slope of the linear line fitted to the data, and each sample crystallite size is calculated from the intercept on the y-axis. Table displays the computed values of strain and crystallite size. Table shows the miller indices and peak lists for the parent and composite materials.
Table 2. Miller indices and peak lists of AlCrO3 and GO/AlCrO3/SiO2/Mn3O4/SnO2 nanocomposite.
4.2. SEM analysis
Figure (a) shows SEM image of graphene oxide as due to the presence of functional groups primarily oxygen. GO was found to be a two-dimensional material with a sheet-like lamellar structure and increased sheet thickness near the edges. Due to their firmly suspended morphology, GO sheets did not exhibit bending behaviour [Citation50]. The aluminium chromite nanoparticles maintained their nearly spherical morphology, while the graphene oxide (GO) nanoparticles were effectively incorporated onto the surface of the aluminium chromite resulting in the formation of a nanocomposite as depicted in Figure (b). The subsequent visualization of GO@AlCrO3@SiO2@Mn3O4@SnO2 reveals a complex, layered structure as illustrated in Figure (c). The image exhibits a relatively high aspect ratio suggesting that the quasi-spherical agglomerated morphology is attributed to the presence of approximately 127 spherical particles (depicted as lighter areas). Degradation of the GO layer became evident due to structural imperfections, aligning with findings from XRD measurements. Furthermore, the interplanar distance of 16 nm can be attributed to the (222) plane of α-MnO2 within the Mn3O4 crystals. The grain size evaluation using the Image J. program yielded dimensions of 22 nm for the graphene oxide, 7 nm for the aluminium chromite and 20 nm for composite. Additionally, the cumulative frequency and per-count histograms of the composite are illustrated in Figure for further characterizations.
4.3. FTIR spectroscopy
For each nanomaterial molecular bonding investigation, we plotted out its FTIR spectrum evaluation as shown in Figure . The FTIR spectra of GO give you an idea about absorption peaks at 766, 1253, 1570 cm−1 and 2093cm−1. In addition to sort of absorption bands, metal–oxygen stretching is responsible for both tetrahedral and octahedral sites [Citation51]. The angular distortion of water molecules absorbed is believed responsible for the band at 1253 cm−1. The C – H stretching vibrations cause a tiny bar at 2093cm−1 [Citation52,Citation53]. The band represents the bending vibrations of O-H groups at 1570 cm−1 [Citation54]. The bending sensations of OH groups were a symbol of bands at the same position, while the band signified the stretching feelings of OH groups at 2097cm−1. The perovskites lattice octahedral and tetrahedral aluminium or manganese ions are responsible for these two bands vibrations [Citation55]. Absorbed water molecules bend their vibrations at 1523 cm−1, which is the source of the band Al3+ O2 – in the octahedral sites and Mn2 + O2 – in the tetrahedral sites in synthesized Mn3O4 [Citation56]. This indicates that spinel Mn3O4 is present in the material. There is no change in the absorption band despite a secondary phase (Al), suggesting that Al2+ and Mn3+ occupy the tetra and octahedral sites respectively [Citation57]. Perovskite structure had an effect on wavenumber peculiar to these two bands, which lies between the ethics of ternary oxides analogous to Al and Mn ions in spinel structure about GO@AlCrO3@SiO2@Mn3O4@SnO2 [Citation58]. The vibration wavenumbers are somewhere between ternary metal oxides and multinary oxide samples. At the same time AlCrO3 (8.11) and Mn3O4 (8.19) have the highest lattice parameter values. All peaks shift due to structure change varying ionic radii of different metals and various cation distributions.
4.4. Energy dispersive spectroscopy
Figure showing EDS spectra of AlCrO3 with maximum intensity peak of aluminium accompanied by low intensity peaks of chromium and oxygen. Nanocomposite EDS spectra is visible in Figure with an impurity peak of sodium is visible in minor concentration might be due to beaker impurities existence [Citation13,Citation59]. AlCrO3 elements weight ratios are mentioned in Figure whereas nanocomposite elemental percentage can be seen in Figure respectively.
4.5. Raman spectroscopy
The Raman spectra spanning wavelengths from 1200 cm−1 to 1600 cm−1 are presented in Figure illustrating the Raman spectrum of GO (Figure (a)) alongside the Raman spectrum of the GO@AlCrO3@SiO2@Mn3O4@SnO2 nanocomposite (Figure (b)). Within the spectrum of GO, two distinct peaks are observed at 1352 and 1578 cm−1 corresponding to the D band and the G band of GO respectively. The Raman-active E2g mode at 1578 cm−1 confirms the presence of a graphite structure, while the pronounced D band suggests sp³ defects within the graphene sheet [Citation60]. The introduction of oxygen functionalities enhances the number of edge sites, subsequently amplifying the intensity of the D band in GO. The intensity ratio between the D and G bands (ID/IG) serves as a measure of structural deviations. This ratio increases from 0.83 for GO to 0.95 for the GO@AlCrO3@SiO2@Mn3O4@SnO2 nanocomposite. This elevation can be attributed to the hydrothermal process ability to restore the sp2 network upon reduction [Citation61]. In the case of the nanocomposite, the D band undergoes a red shift to 1351 cm−1, while the G band experiences a blue shift to 1589.90 cm−1 compared to GO. This phenomenon can be attributed to the presence of nanoparticles within the nanocomposite and the charge transfer between GO and SnO2 [Citation62,Citation63].
4.6. Ultra-Visible spectroscopy
For the nanocomposite GO@AlCrO3@SiO2@Mn3O4@SnO2 there are metallic absorption peaks at 260 and 630 nm but none can be identified [Citation64]. Another name given to absorption spectroscopy is UV-Visible spectroscopy related to UV (ultraviolet) light absorbance in nanomaterial as shown in Figure [Citation65]. No obvious absorption edge is shown by GO confirming graphene oxide metallic nature. In case of GO@AlCrO3@SiO2@Mn3O4@SiO2@SnO2 nanocomposite, absorption edges shifted to longer wavelengths. Bandgap energies of nanocomposite is determined by drawing plot between (αhʋ)1/2 versus hʋ as 2.85 eV. Obviously quantum size effect and bandgap narrowing are due to GO hybridization within nanocomposite [Citation66–68].
4.7. Zeta measurements
Surface charge stability investigations necessitate Zeta potential measurements. These measurements have revealed a significant relationship between the rate at which the negatively charged electrode attracts the positively charged electrode and a potential difference of approximately 100 volts [Citation69,Citation70]. Remarkably, the GO@AlCrO3@SiO2@Mn3O4@SnO2 nanocomposite demonstrates a remarkable capability to uphold a stable colloidal solution. This stability is achieved through the augmentation of electrostatic repulsion forces among the nanoparticles, as visually depicted in Figure .
4.8. Electrochemical measurements
The Gamry potential state interface 1000 instrument was utilized to perform the electrochemical analysis using an Ag/AgCl type reference electrode, also platinum wire using counter electrode and a glassy carbon electrode as the working electrode [Citation71]. Before fabricating using the powder-produced materials, the working electrode was always washed with alumina slurry and Ethanol. For GCE preparation 0.02 g of powdered electrode material was put on the surface, and 5% Nafion solution was added [Citation72]. It took 20 min in a 50 in an oven to dry the nano – electrode material after it was produced.
4.8.1. CV measurements
Cyclic voltammetry was employed to analyse the electrode potential in alkaline electrolytes using the modified working electrodes. As illustrated in Figure , cyclic voltammograms were captured using a GO@AlCrO3@SiO2@Mn3O4@SnO2 electrode immersed in 1M KOH solution with scan rates varying from 10 to 100 mVs−1. Notably, the nanocomposites exhibited capacitive behaviour, where the forward scan corresponded to anodic peaks (oxidation) and the reverse scan to cathodic peaks (reduction). This behaviour provided further evidence of surface redox processes occurring. The specific capacitance of the working electrodes (GO@AlCrO3@SiO2@Mn3O4@SnO2 nanocomposite) was determined using the equation where Cp represents specific capacitance in F/g, m signifies the electrode mass in mg (0.02 mg), and k is a constant. The estimated electrochemical capacitance parameters are summarized in Table . Notably, the capacitance exhibited an inverse relationship with the scan rate. At a scan rate of 20 mVs−1 in 1M KOH solution, a substantial specific capacitance of 2495 Fg−1 was recorded. However, the specific capacitance declined as the scan rate increased, since the penetration of electrolyte ions into the interior of the electrode material decreased, consequently reducing its charge storage capacity [Citation73]. Reduced scan rates allow electrolyte ions to use less surface area, leading to higher specific capacitance [Citation74]. Diffusion coefficient (D°) values for 1M KOH Pseudo capillary capacitance at the electrode inner surface can be explained by a diffusion seeing controlled process as shown by a linear type relationship between peak current (Ip) and the square root of scan rate (1/2) in a Randles–Sevcik plot (Figure ).
Table 3. Measurements of specific capacitance of GO@AlCrO3@SiO2@Mn3O4@SnO2 at various scan rates.
Capacitive energy storage applications are commonplace in the graphene oxide composites with heteroatom incorporation [Citation75–77]. The successful fabrication of nanomaterials has yielded an electrode material with a notably enlarged surface area, contributing to its robust electrochemical activity. This enhanced performance is attributed to the nanocomposite larger diffusion coefficient and reduced charge transfer resistance at the electrode–electrolyte interface, making it particularly proficient in basic solutions. Additionally, the heightened apparent rate constant underscores the enhanced utility of the synthesized capacitive material for on-site power applications in alkaline electrolytes. Furthermore, the nanocomposite outperforms certain previously reported capacitive materials by demonstrating substantially elevated capacitance values. This characteristic positions it as an effective medium for energy storage. Remarkably, the GO@AlCrO3@SiO2@Mn3O4@SnO2 nanocomposite exhibits significantly superior capacitance performance, even when operating with lower electrolyte concentrations.
4.8.2. Galvanostatic charge-discharge (GCD) measurements
Figure shows that the galvanostatic charge–discharge type analysis curve exhibits a non-linear behaviour during charging and discharging, which is indicative of the redox-active nature of the material and helps to explain the presence of oxidation and reduction peaks in the CV curve. Discharging times are reduced when current density increases [Citation78–80]. Maximum capacitance and prolonged discharge time are realized at the lowest current density form of special value due to the electrode long residence time for hydroxyl ion insertion into electrode-type material [Citation81]. Energy density and power density are two metrics where an inverse relationship can be shown when defining relations between them and
, correspondingly [Citation82]. Density of power increases from 1092 to 2495.5 W/kg; density of energy decreases from 67.388–48.611 Wh/kg. Nanocomposite electrode material is suggested as a promising electro-based active one for supercapacitor based devices [Citation83–85].
4.8.3. Electrochemical impedance spectroscopy
Modified electrode using glassy carbon electrode (GCE) electron transfer capacity analysed via EIS in both 1M KOH electrolytes by drawing Nyquist plot as shown in Figure . The prepared electrode EIS parameters are shown in Table whereas the electron transfer rate is evaluated using the formula In this equation C is electrolyte molar concentration, R is the universal gas constant, T is standard temperature, F is Faraday's constant, and Rct is charge transfer resistance [Citation86–88]. Charge transfer resistance (Rct) decreased value for 1M KOH confirms the maximum electron transfer rate (Kapp) resulting in maximum conductivity. It is worth noting that electrode modification affects Rct as it represents active material conductive behaviour [Citation89,Citation90]. The roughness parameter α value of the modified electrode ranges between 0.92-0.64, indicating fair roughness [Citation91]. The highest electron transfer rate constant value for 1M KOH is an indication of reaction facilitation [Citation92–94]. The compassion of the current work with the existing literature is depicted in Table .
Table 4. GO@AlCrO3@SiO2@Mn3O4@SnO2 nanocomposite electrode material parameters.
Table 5. Comparison of multinary composite with previous literature.
4. Conclusion
Exploring the potential advantages of a novel electrolyte formed through the amalgamation of distinct nanostructured components holds significant importance. The primary objective here is to comprehensively assess the capabilities and performance attributes of the inventive multinary nanocomposite based on GO@AlCrO3@Mn3O4@SiO2@SnO2 as an electrode material within an electrochemical supercapacitor. To achieve this goal, an extensive array of analytical techniques was employed encompassing XRD, SEM, EDS, Raman, UV-visible, FTIR, Zeta measurements, and cyclic voltammetry. Electrochemical impedance spectroscopy, the electrochemical active surface area, as well as power densities were also scrutinized. The FTIR spectra delineate noteworthy bands at 2097cm−1 and 1523 cm−1 signifying stretched vibrations indicative of the metal–oxygen interactions. EDs spectra affirm the presence of aluminium, silicon, tin, chromium and manganese in addition to oxygen. UV-Vis spectra shows the energy bandgap value of approximately 2.85 eV. The material stability is confirmed by a zeta potential value of 27.9 mV. The findings demonstrate remarkable power density ranging from 1710 to 2495.5 W/kg and energy density spanning from 67.38–48.61 Wh/kg. These outcomes underscore the nanocomposite-based material pronounced performance, particularly at heightened current densities displaying commendable stability and impressive energy efficiency. As a result, this material holds significant promise for utilization as an energy storage medium especially within the domain of supercapacitors.
Acknowledgement
We are thankful to National Institute of Lasers and Optronics (NILOP) College PIEAS, NILORE, Islamabad for provision of characterization facilities. This work was funded by the Researchers Supporting Project Number (RSP2024R243) King Saud University, Riyadh, Saudi Arabia.
Disclosure statement
No potential conflict of interest was reported by the author(s).
Additional information
Funding
References
- Ansari MZ, Ansari SA, Kim SH. Fundamentals and recent progress of Sn-based electrode materials for supercapacitors: A comprehensive review. J Energ Storage. 2022;53:105187. doi:10.1016/j.est.2022.105187
- Ansari MZ, Nandi DK, Janicek P, et al. Low-temperature atomic layer deposition of highly conformal tin nitride thin films for energy storage devices. ACS Appl Mater Interfaces. 2019;11(46):43608–43621. doi:10.1021/acsami.9b15790
- Parveen N, Ansari SA, Ansari MZ, et al. Manganese oxide as an effective electrode material for energy storage: A review. Environ Chem Lett. 2022;1:1–27. doi:10.1007/s10311-021-01316-6
- Sun W, Lipka SM, Yang F. Activated carbon derived from hemp and Its Use in electrochemical capacitors. InElectrochemical Society Meeting Abstracts 228 2015 7 9, 587–587. The Electrochemical Society, Inc. doi:10.1149/MA2015-02/9/587
- Du X, Ren X, Xu C, et al. Recent advances on the manganese cobalt oxides as electrode materials for supercapacitor applications: A comprehensive review. J Energ Storage. 2023;68:107672. doi:10.1016/j.est.2023.107672
- Ren X, Bao E, Liu X, et al. Advanced hybrid supercapacitors assembled with beta-Co(OH)2 microflowers and microclews as high-performance cathode materials. Colloids Surf, A. 2023;667:131391.doi:10.1016/j.colsurfa.2023.131391
- Sun J, Xu C, Chen H. A review on the synthesis of CuCo2O4-based electrode materials and their applications in supercapacitors. J Materiom. 2021;7(1):98–126. doi:10.1016/j.jmat.2020.07.013
- Bao E, Ren X, Wu R, et al. Porous MgCo2O4 nanoflakes serve as electrode materials for hybrid supercapacitors with excellent performance. J Colloid Interface Sci. 2022;625:925–935. doi:10.1016/j.jcis.2022.06.098
- Sun J, Xu C, Chen H. A review on the synthesis of CuCo2O4-based electrode materials and their applications in supercapacitors. J Materiom. 2021;7(1):98–126. doi:10.1016/j.jmat.2020.07.013
- Bao E, Sun J, Liu Y, et al. Facile preparation of SnS2 nanoflowers and nanoplates for the application of high-performance hybrid supercapacitors. Int J Hydrogen Energy. 2022;47(92):39204–39214. doi:10.1016/j.ijhydene.2022.09.081
- Gogotsi Y, Simon P. True performance metrics in electrochemical energy storage. science. 2011;334(6058):917–918. doi:10.1126/science.1213003
- Fernando JF. Reduction of reactive power waste of inductive electrical appliances using power factor correction. Vidyodaya J Sci. 2021;21(1):7–12. doi:10.31357/vjs.v24i01.4960
- Shafique R, Mahmood A, Batool K, et al. Graphene oxide/nickel chromite nanocomposite: optimized synthesis, structural and optical properties. ECS J Solid State Sci Technol. 2021;10(10):101005. doi:10.1149/2162-8777/ac2911
- Slater MD, Kim D, Lee E, et al. Sodium-ion batteries. Adv Funct Mater. 2013;23(8):947–958. doi:10.1002/adfm.201200691
- Zhang Z, Mu S, Zhang B, et al. A novel synthesis of carbon nanotubes directly from an indecomposable solid carbon source for electrochemical applications. J Mater Chem A. 2016;4(6):2137–2146. doi:10.1039/C5TA09631F
- Xu C, Wang X, Zhu J. Graphene−metal particle nanocomposites. J Phys Chem C. 2008;112(50):19841–5. doi:10.1021/jp807989b
- Reddy AL, Shaijumon MM, Gowda SR, et al. Coaxial MnO2/carbon nanotube array electrodes for high-performance lithium batteries. Nano Lett 2009;9(3):1002–1006. doi:10.1021/nl803081j
- Kolts JH, Delzer GA. Enhanced ethylene and ethane production with free-radical cracking catalysts. Science. 1986;232(4751):744–746. doi:10.1126/science.232.4751.744
- Fouda MF, Amin RS, Selim MM. Thermal and spectroscopic characterization of reaction products of al-nitrate-Cr-nitrate interaction at various temperatures. Thermochim Acta. 1989;141:277–291. doi:10.1016/0040-6031(89)87063-7
- Fouda MF, Amin RS, Selim MM. Thermal and spectroscopic characterization of reaction products of Al-nitrate-Cr-carbonate interaction at different temperatures. React Solids. 1990;8(1-2):21–28. doi:10.1016/0168-7336(90)80004-4
- Heinemann H, Carberry JJ, editors. Catalysis reviews: science and engineering. New York: M. Dekker; 1974.
- Thackeray MM, David WI, Bruce PG, et al. Lithium insertion into manganese spinels. Mater Res Bull. 1983;18(4):461–472. doi:10.1016/0025-5408(83)90138-1
- Pankov VV, Fournier T, Pernet M, et al. Superconductors prepared by the diffusion couples technique. Mater Res Bull. 1993;28(1):9–17. doi:10.1016/0025-5408(93)90003-V
- Piligkos S, Rajaraman G, Soler M, et al. Studies of an enneanuclear manganese single-molecule magnet. J Am Chem Soc 2005;127(15):5572–5580. doi:10.1021/ja042302x
- Yoshikai N, Zhang SL, Yamagata KI, et al. Mechanistic study of the manganese-catalyzed [2 + 2 + 2] annulation of 1,3-dicarbonyl compounds and terminal alkynes. J Am Chem Soc 2009;131(11):4099–4109. doi:10.1021/ja809202y
- Ding S, Luan D, Boey FY, et al. Sno2 nanosheets grown on graphene sheets with enhanced lithium storage properties. Chem Commun. 2011;47(25):7155–7157. doi:10.1039/c1cc11968k
- Huang X, Zhou X, Zhou L, et al. A facile One-step solvothermal synthesis of SnO2/graphene nanocomposite and Its application as an anode material for lithium-Ion batteries. ChemPhysChem. 2011;12(2):278–281. doi:https://doi.org/10.1002/cphc.201000376.
- Liu XG, Geng DY, Meng H, et al. Microwave absorption properties of FCC-Co/Al2O3 and FCC-Co/Y2O3 nanocapsules. Solid State Commun. 2009;149(1-2):64–67. doi:10.1016/j.ssc.2008.10.015
- Jang Y, Choi JJ, Kwon YM, et al. 3D (3-dimensional) porous silver nonwoven mats prepared with cellulosic templates and spray equipment for use as supercapacitor current collectors. Energy. 2015;93:1303–1307. doi:10.1016/j.energy.2015.10.013
- Rakhi RB, Lekshmi ML. Reduced graphene oxide based ternary nanocomposite cathodes for high-performance aqueous asymmetric supercapacitors. Electrochim Acta. 2017;231:539–548. doi:10.1016/j.electacta.2017.02.095
- Yanik MO, Yigit EA, Akansu YE, et al. Magnetic conductive polymer-graphene nanocomposites based supercapacitors for energy storage. Energy. 2017;138:883–889. doi:10.1016/j.energy.2017.07.022
- Ai W, Zhou W, Du Z, et al. Benzoxazole and benzimidazole heterocycle-grafted graphene for high-performance supercapacitor electrodes. J Mater Chem. 2012;22(44):23439–23446. doi:10.1039/c2jm35234f
- Zhang L, Jamal R, Zhao Q, et al. A highly efficient flexible dye-sensitized solar cell based on nickel sulfide/platinum/titanium counter electrode. Nanoscale Res Lett. 2015;10:1–9. doi:10.1186/1556-276X-10-1
- Navrotsky A. Energetics and crystal chemical systematics among ilmenite, lithium niobate, and perovskite structures. Chem Mater. 1998;10(10):2787–2793. doi:10.1021/cm9801901
- Luo J, Jang HD, Sun T, et al. Compression and aggregation-resistant particles of crumpled soft sheets. ACS Nano. 2011;5(11):8943–8949. doi:10.1021/nn203115u
- Wang WN, Jiang Y, Biswas P. Evaporation-induced crumpling of graphene oxide nanosheets in aerosolized droplets: confinement force relationship. J Phys Chem Lett. 2012;3(21):3228–3233. doi:10.1021/jz3015869
- Chen Y, Guo F, Jachak A, et al. Aerosol synthesis of cargo-filled graphene nanosacks. Nano Lett 2012;12(4):1996–2002. doi:10.1021/nl2045952
- Chen Y, Guo F, Qiu Y, et al. Encapsulation of particle ensembles in graphene nanosacks as a new route to multifunctional materials. Acs Nano. 2013;7(5):3744–3753. doi:10.1021/nn3055913
- Chadli I, Omari M, Abu Dalo M, et al. Preparation by sol–gel method and characterization of Zn-doped LaCrO3 perovskite. J Solgel Sci Technol. 2016;80:598–605. doi:10.1007/s10971-016-4170-5
- Wang G, Ma Z, Fan Y, et al. Preparation of size-selective Mn3O4hexagonal nanoplates with superior electrochemical properties for pseudocapacitors. Phys Chem Chem Phys. 2015;17(35):23017–23025. doi:10.1039/C5CP03366G
- Shafique R, Rani M, Mahmood A, et al. Copper chromite/graphene oxide nanocomposite for capacitive energy storage and electrochemical applications. Int J Environ Sci Technol. 2022;19(8):7517–7526. doi:10.1007/s13762-021-03616-4
- Zhang H, Peng C, Yang J, et al. Uniform ultrasmall graphene oxide nanosheets with Low cytotoxicity and high cellular uptake. ACS Appl. Mater. Interfac. 2013;5(5):1761–1767. doi:10.1021/am303005j
- Sun D, Tan Z, Tian X, et al. Graphene: A promising candidate for charge regulation in high-performance lithium-ion batteries. Nano Res. 2021;14(12):4370–4385. doi:10.1007/s12274-021-3405-0
- He H, Gao C. Supraparamagnetic, conductive, and processable multifunctional graphene nanosheets coated with high-density Fe3O4nanoparticles. ACS Appl Mater Interfaces. 2010;2(11):3201–3210. doi:10.1021/am100673g
- Liu J, Cheng J, Che R, et al. Double-Shelled yolk–shell microspheres with Fe3O4cores and SnO2double shells as high-performance microwave absorbers. J Phys Chem C. 2013;117(1):489–495. doi:10.1021/jp310898z
- Cao MS, Yang J, Song WL, et al. Ferroferric oxide/multiwalled carbon nanotube vs polyaniline/ferroferric oxide/multiwalled carbon nanotube multiheterostructures for highly effective microwave absorption. ACS Appl Mater Interfaces. 2012;4(12):6949–6956. doi:10.1021/am3021069
- Zhu M, Meng D, Wang C, et al. Facile fabrication of hierarchically porous CuFe2O4nanospheres with enhanced capacitance property. ACS Appl Mater Interfaces. 2013;5(13):6030–6037. doi:10.1021/am4007353
- Pendashteh A, Rahmanifar MS, Kaner RB, et al. Facile synthesis of nanostructured CuCo2O4 as a novel electrode material for high-rate supercapacitors. Chem Commun. 2014;50(16):1972–1975. doi:10.1039/c3cc48773c
- Adams DR, Meyers SA, Beidas RS. The relationship between financial strain, perceived stress, psychological symptoms, and academic and social integration in undergraduate students. J Am Coll Health. 2016;64(5):362–370. doi:10.1080/07448481.2016.1154559
- Debataraja A, Muchtar AR, Septiani NL, et al. High performance carbon monoxide sensor based on nano composite of SnO2-graphene. IEEE Sens J. 2017;17(24):8297–8305. doi:10.1109/JSEN.2017.2764088
- Lefez B, Nkeng P, Lopitaux J, et al. Characterization of cobaltite spinels by reflectance spectroscopy. Mater Res Bull. 1996;31(10):1263–1267. doi:10.1016/0025-5408(96)00122-5
- Guan C, Xia X, Meng N, et al. Hollow core–shell nanostructure supercapacitor electrodes: gap matters. Energy Environ Sci. 2012;5(10):9085–9090. doi:10.1039/c2ee22815g
- Pan GX, Xia XH, Cao F, et al. Template-free synthesis of hierarchical porous Co 3 O 4 microspheres and their application for electrochemical energy storage. Electrochim Acta. 2015;173:385–392. doi:10.1016/j.electacta.2015.05.078
- Patake VD, Lokhande CD, Joo OS. Electrodeposited ruthenium oxide thin films for supercapacitor: effect of surface treatments. Appl Surf Sci. 2009;255(7):4192–4196. doi:10.1016/j.apsusc.2008.11.005
- Aghazadeh M. Electrochemical preparation and properties of nanostructured Co3O4 as supercapacitor material. J Appl Electrochem. 2012;42:89–94. doi:10.1007/s10800-011-0375-z
- Li Q, Zeng L, Wang J, et al. Magnetic mesoporous organic−inorganic NiCo2O4hybrid nanomaterials for electrochemical immunosensors. ACS Appl Mater Interfaces. 2011;3(4):1366–1373. doi:10.1021/am200228k
- Hwang SG, Ryu SH, Yun SR, et al. Behavior of NiO–MnO2/MWCNT composites for use in a supercapacitor. Mater Chem Phys. 2011;130(1-2):507–512. doi:10.1016/j.matchemphys.2011.07.022
- Verma S, Joshi HM, Jagadale T, et al. Nearly monodispersed multifunctional NiCo2O4spinel nanoparticles: magnetism, infrared transparency, and radiofrequency absorption. J Phys Chem C. 2008;112(39):15106–15112. doi:10.1021/jp804923t
- Batool K, Rani M, Younus A, et al. Nanosized magnesium doped copper chromites spinel particles synthesis and characterization. ECS J Solid State Sci Technol. 2020;9(12):126005. doi:10.1149/2162-8777/abce00
- Wang T, Liu Z, Lu M, et al. Graphene–Fe3O4 nanohybrids: synthesis and excellent electromagnetic absorption properties. J Appl Phys. 2013;113(2):024314–024314. doi:10.1063/1.4774243.
- Liu PB, Huang Y, Sun X. Excellent electromagnetic absorption properties of poly(3,4-ethylenedioxythiophene)-reduced graphene oxide–Co3O4composites prepared by a hydrothermal method. ACS Appl Mater Interfaces. 2013;5(23):12355–12360. doi:10.1021/am404561c
- Yu H, Wang T, Wen B, et al. Graphene/polyaniline nanorod arrays: synthesis and excellent electromagnetic absorption properties. J Mater Chem. 2012;22(40):21679–21685. doi:10.1039/c2jm34273a
- Huang H, Huang Y, Wang M, et al. Preparation of hollow Zn2SnO4 boxes@C/graphene ternary composites with a triple buffering structure and their electrochemical performance for lithium-ion batteries. Electrochim Acta. 2014;147:201–208. doi:10.1016/j.electacta.2014.09.117
- Alamdari S, Ghamsari MS, Afarideh H, et al. Preparation and characterization of GO-ZnO nanocomposite for UV detection application. Opt Mater (Amst). 2019;92:243–250. doi:10.1016/j.optmat.2019.04.041
- Li R, Zhang L, Shi L, et al. Mxene Ti3C2: An effective 2D light-to-heat conversion material. ACS Nano. 2017;11(4):3752–3759. doi:10.1021/acsnano.6b08415
- Liu J, Wu Q, Yi Y, et al. Assembling synthesis of barium chromate nano-superstructures using eggshell membrane as template. Bull Korean Chem Soc. 2004;25(12):1775–1778. doi:10.5012/bkcs.2004.25.12.1775
- Luo Q, Chai B, Xu M, et al. Study of nanostructure and ethanol vapor sensing performance of WO3 thin films deposited by e-beam evaporation method under different deposition angles: application in breath analysis devices. Appl Phys A. 2018;124:1–8. doi:10.1007/s00339-017-1423-2
- Lian W, Wang L, Wang X, et al. Facile preparation of BiOCl/Ti3C2hybrid photocatalyst with enhanced visible-light photocatalytic activity. Funct Mater Lett. 2019;12(01):1850100. doi:10.1142/S179360471850100X
- Baskoro F, Wong CB, Kumar SR, et al. Graphene oxide-cation interaction: inter-layer spacing and zeta potential changes in response to various salt solutions. J Memb Sci. 2018;554:253–263. doi:10.1016/j.memsci.2018.03.006
- Khalil WF, El-Sayyad GS, Rouby E, et al. Graphene oxide-based nanocomposites (GO-chitosan and GO-EDTA) for outstanding antimicrobial potential against some candida species and pathogenic bacteria. Int J Biol Macromol 2020;164:1370–1383. doi:10.1016/j.ijbiomac.2020.07.205
- Yaqoob T, Rani M, Neffati R, et al. Novel GO/LiCr2O4 nanocomposite synthesis, characterizations and electrode testing for electrochemical applications. Mater Sci Engin B. 2023;287:116118. doi:10.1016/j.mseb.2022.116118
- Lufrano F, Staiti P. Performance improvement of nafion based solid state electrochemical supercapacitor. Electrochim Acta. 2004;49(16):2683–2689. doi:10.1016/j.electacta.2004.02.021
- Sahu SC, Samantara AK, Dash A, et al. Graphene-induced Pd nanodendrites: A high performance hybrid nanoelectrocatalyst. Nano Res. 2013;6:635–643. doi:10.1007/s12274-013-0339-1
- Sagadevan S, Zaman Chowdhury Z, Johan MR, et al. A one-step facile route synthesis of copper oxide/reduced graphene oxide nanocomposite for supercapacitor applications. J Exp Nanosci. 2018;13(1):284–296. doi:10.1080/17458080.2018.1542512
- Korkmaz S, Kariper İA. Graphene and graphene oxide based aerogels: synthesis, characteristics and supercapacitor applications. J Energ Storage. 2020;27:101038. doi:10.1016/j.est.2019.101038
- Arvas MB, Gencten M, Sahin Y. One-step synthesized N-doped graphene-based electrode materials for supercapacitor applications. Ionics (Kiel). 2021;27:2241–2256. doi:10.1007/s11581-021-03986-2
- Obodo RM, Ahmad A, Jain GH, et al. 8.0 MeV copper ion (Cu++) irradiation-induced effects on structural, electrical, optical and electrochemical properties of Co3O4-NiO-ZnO/GO nanowires. Mater Sci Energ Technol. 2020;3:193–200. doi:10.1016/j.mset.2019.10.006
- Iqbal MZ, Haider SS, Siddique S, et al. Capacitive and diffusion-controlled mechanism of strontium oxide based symmetric and asymmetric devices. J Energ Storag. 2020;27:101056. doi:10.1016/j.est.2019.101056
- Iqbal MZ, Faisal MM, Ali SR, et al. Co-MOF/polyaniline-based electrode material for high performance supercapattery devices. Electrochim Acta. 2020;346:136039. doi:10.1016/j.electacta.2020.136039
- Iqbal MZ, Khan J. Optimization of cobalt-manganese binary sulfide for high performance supercapattery devices. Electrochim Acta. 2021;368:137529. doi:https://doi.org/10.1016/j.electacta.2020.137529.
- Akram M, Rani M, Batool K, et al. Synthesis and characterization of quaternary GO/CoCrO3/SiO2/Ag2WO4Nanocomposite based on energy storage and photocatalytic applications. Mater Sci Engin B. 2023;298:116838. doi:10.1016/j.mseb.2023.116838
- Batool K, Rani M, Rasool F, et al. Multinary nanocomposite of GO@SrO@CoCrO3@FeCr2O4@SnO2@SiO2 for superior electrochemical performance and water purification applications. Heliyon. 2023;9(4):e20675–e20675-15.
- Alam S, Iqbal MZ. Nickel-manganese phosphate: An efficient battery-grade electrode for supercapattery devices. Ceram Int. 2021;47(8):11220–11230. doi:10.1016/j.ceramint.2020.12.247
- Alam S, Iqbal MZ, Khan J. Green synthesis of nickel-manganese/polyaniline-based ternary composites for high-performance supercapattery devices. Int J Energy Res. 2021;45(7):11109–11122. doi:10.1002/er.6593
- Alzaid M, Iqbal MZ, Alam S, et al. Binary composites of nickel-manganese phosphates for supercapattery devices. J Energ Storage. 2021;33:102020. doi:10.1016/j.est.2020.102020
- Aguedo J, Lorencova L, Barath M, et al. Electrochemical impedance spectroscopy on 2D nanomaterial MXene modified interfaces: application as a characterization and transducing tool. Chemosensors. 2020;8(4):127. doi:10.3390/chemosensors8040127
- Ali SR, Iqbal MZ, Faisal MM, et al. Diffusion control and surface control mechanism in hierarchical nanostructured porous zinc-basedMOFmaterial for supercapattery. Int J Energy Res. 2022;46(10):14424–14435.
- Ayman I, Rasheed A, Ajmal S, et al. CoFe2O4nanoparticle-decorated 2D MXene: A novel hybrid material for supercapacitor applications. Energy Fuels. 2020;34(6):7622–7630. doi:10.1021/acs.energyfuels.0c00959
- Ali SR, Iqbal MZ, Faisal MM, et al. Diffusion control and surface control mechanism in hierarchical nanostructured porous zinc-basedMOFmaterial for supercapattery. Int J Energy Res. 2022;46(10):14424–14435. doi:10.1002/er.8169
- Iqbal MZ, Alam S, Afzal AM, et al. Binary composites of strontium oxide/polyaniline for high performance supercapattery devices. Solid State Ionics. 2020;347:115276. doi:10.1016/j.ssi.2020.115276
- Akram M, Rani M, Shafique R, et al. Fabrication of LaCrO3@SiO2 nanoparticles supported with graphene-oxide for capacitive energy storage and photocatalytic degradation applications. J Inorg Organomet Polym Mater. 2024;34:361–373.
- Butt TM, Janjua NK, Mujtaba A, et al. B-site doping in lanthanum cerate nanomaterials for water electrocatalysis. J Electrochem Soc. 2020;167(2):0026503. doi:10.1149/1945-7111/ab63c0
- Eloul S, Batchelor-McAuley C, Compton RG. Thin film-modified electrodes: a model for the charge transfer resistance in electrochemical impedance spectroscopy. J Solid State Electrochem. 2014;18:3239–3243. doi:10.1007/s10008-014-2662-1
- Heiduschka P, Munz AW, Göpel W. Impedance spectroscopy and scanning tunneling microscopy of polished and electrochemically pretreated glassy carbon. Electrochim Acta. 1994;39(14):2207–2223. doi:10.1016/0013-4686(94)E0166-W
- Shinde SK, Yadav HM, Ghodake GS, et al. Novel and efficient hybrid supercapacitor of chemically synthesized quaternary 3D nanoflower-like NiCuCo2S4 electrode. Ceram Int. 2021;47(11):15639–15647. doi:10.1016/j.ceramint.2021.02.134
- Isacfranklin M, Yuvakkumar R, Ravi G, et al. Quaternary Cu2FeSnS4/PVP/rGO composite for supercapacitor applications. ACS Omega. 2021;6(14):9471–9481. doi:10.1021/acsomega.0c06167
- Wang F, Yao G, Xu M, et al. Large-scale synthesis of macroporous SnO2 with/without carbon and their application as anode materials for lithium-ion batteries. J Alloys Compd. 2011;509(20):5969–5973. doi:10.1016/j.jallcom.2011.03.041