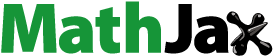
ABSTRACT
During the 35th Indian Scientific Expedition to Antarctica, measurements of atmospheric carbon dioxide (CO2) were carried out using a Li-Cor CO2/H2O analyser at Bharati, the Indian Antarctic research station. This study examines the short-term variability of atmospheric CO2 during the austral summer (January–February) of 2016. An average of 396.25 ± 4.20 ppm was observed during the study period. Meteorological parameters such as relative humidity, precipitation, wind speed, air temperature and atmospheric boundary layer height in conjunction with photosynthetically active radiation, the biological activity indicator which modulates atmospheric CO2 concentration have been investigated. High wind speed (>20 m s−1) combined with precipitation scavenges CO2 in the atmosphere, resulting in low concentrations at the study site. The lowest CO2 concentration of 385 ppm coincided with heavy precipitation of 15 mm during study period. Statistical analysis of the data shows that precipitation and relative humidity independently correlated 55% (r = −0.55) and 32% (r = −0.32), respectively, with the variability of CO2 mixing in the atmosphere at the study site. Atmospheric CO2 was significantly correlated with precipitation alone with a p value of 0.003. Further, multiple regression analysis was performed to test the significant relation between variability of atmospheric CO2 and meteorological parameters. Long-range air-mass transport analysis depicted that the majority of the air masses are reaching the study site through the oceanic region.
Introduction
CO2, CH4 and H2O are the major greenhouse gases in the atmosphere on account of their abundance and contribution to the greenhouse effect (Stocker et al. Citation2013).The greenhouse gases play a role in the climate system by absorbing long-wave infrared radiation. CO2 levels have been consistently increasing since pre-industrial times and daily mean values reached 400 ppm in May 2013 at the reference site of Mauna Loa, Hawaii (Monastersky Citation2013). This increase is caused by human activities and is contributing to increasing the Earth’s surface temperature (Huang et al. Citation2016). A study by Turner & Overland (Citation2009) indicated that northern and western regions of Antarctica are warming by +0.56°C per decade. Atmospheric CO2 concentrations are determined by mechanisms such as respiration and photosynthesis in the terrestrial biosphere, anthropogenic emissions, land use and land cover as well as uptake by oceans. Depending upon partial pressure of CO2 and AT, CO2 dissolves in the atmosphere, producing a weak carbonic acid, H2CO3 (Lower Citation1999). Snow scavenges atmospheric species such as CO2, O3 and black carbon from the atmosphere (Chaubey et al. Citation2010). Takagi et al. (Citation2005) explained the role of snow cover on emissions of CO2 from snowpack to the atmosphere. A large difference in CO2 was observed below and above the snow surface, which indicates that the snow cover act as cap for CO2 between the atmosphere and the snowpack. Local and long-range winds play a significant role in wind-driven mass transfer between snow and atmosphere (Jones et al. Citation1999; Takagi et al. Citation2005). In tropical regions, humidity plays a significant role in mixing of CO2 in the atmosphere through a dilution process (Mahesh et al. Citation2014; Sreenivas et al. Citation2016).
Polar regions are the most important soil carbon reservoirs on Earth (Gutt et al. Citation2012; Carvalho et al. Citation2013). C.D. Keeling et al. (Citation1976) brought out that the concentration of CO2 in the Antarctic atmosphere increased by 3.7% from 1957 to 1971. At Jubany Station, Antarctica (62° 14ʹ S, 58° 40ʹ W), CO2 measurements, based on a non-dispersive infrared gas analyser, showed an increasing trend, from 356.75 ppm in 1994 to 384.74 ppm in 2009, at an average annual rate of 1.3 ppm yr−1 (R.F. Keeling et al. Citation2008). During the 2012–15 period, many other stations in Antarctica, such as Casey (66.28° S, 110.53° E), Syowa (69° S, 39.6° E), Palmer (64.92° S, 64° W), Halley (75.6° S, 26.5° W) and Amundsen–Scott South Pole Station (90° S, 24.8° W), also showed an increasing CO2 trend, with annual rates of 2.40 ppm yr–1, 2.43 ppm yr–1, 2.42 ppm yr–1, 2.52 ppm yr–1 and 2.49 ppm yr–1, respectively. Compared to the 2011–12 period, the annual increases of CO2 observed in 2014–15 at these sites were 0.54–0.56%, 0.57–0.63%, 0.53–0.63% and 0.53–0.57%, respectively, except over Casey (0.56–0.52%) (Sun et al. Citation2014). Over Antarctica, background CO2 concentrations showed an average growth rate of 2.10 ppm yr–1, with the highest during summer (Cristofanelli et al. Citation2011). During 2015, CO2 rates of increase over Antarctica stations are lesser (higher) than global (Antarctica as a whole) rate of 2.93 (2.10) ppm yr−1. The monthly mean CO2 mole fraction measured at Zhongshan Station (69°22′2′′S, 76°21′49′′E) is similar to that of other stations in Antarctica, and their annual amplitudes were all within the range of 384 to 392 ppm during the period 2010 to 2013 (Sun et al. Citation2014). Schmithüsen et al. (Citation2015) showed that raising atmospheric CO2 over most of Antarctica causes an increase in the long-wave cooling in central Antarctica.
Recently, the British Antarctica Survey and the US National Oceanic and Atmospheric Administration have reported 400 ppm of CO2, a milestone record over Antarctica (Kahn Citation2016). The NRSC of the Indian Space Research Organization installed a Li-Cor CO2/H2O analyser at the Indian Antarctic station during 2016, as part of 35th Indian Scientific Expedition to Antarctica, to measure high-frequency CO2 concentration. The objective of the present study is to assess CO2 variability in relation to local meteorological parameters at Bharati, the Indian Antarctic research station, during the austral summer.
Material and methodology
Bharati Station is located in the Larsemann Hills, an Antarctic Specially Managed Area, between Thala Fjord and Quilty Bay, at 69.24° S, 76.11° E (). It is approximately 35 m above the sea level and about 50 m from the seashore. Bharati consists of one multi-purpose building, a satellite camp and a number of smaller container modules. Three diesel-fired combined heat and power-generating units in the main building provide electrical power for the station.
CO2 and H2O are continuously monitored using a Li-Cor CO2/H2O non-dispersive infrared gas analyser (model Li-840A). Meteorological parameters – AT, RH, surface pressure, WS, wind direction and precipitation – were measured using an automatic weather station installed near the CO2 sensor mast; the weather station meets the standards of the World Meteorological Organization. Details of the parameters used, instruments and their make are summarized in . At the site, the prevailing surface winds are from the east–north-east around the year. In quantifying the net precipitation, a known amount of hot water was used to melt the snow collected from the snow gauge.
Table 1. Details of the data used and their sources. The period was 22 January–25 February 2016.
Calibration is crucial for eliminating the instrumental drifts and generating precise, accurate measurements (Mahesh et al. Citation2015). In the present set-up, the Li-Cor analyser is periodically calibrated using National Oceanic and Atmospheric Administration CO2 calibration gases (369.398 ppm and 434.814 ppm), following the World Meteorological Organization’s recommended calibration procedure (Brailsford Citation2012). The precision and accuracy of the instrument were assessed by performing internal calibration with 369.398 ppm and 434.814 ppm spans of CO2. The 60 s (1 σ) average precision of CO2 was 92 ppb and 78 ppb, with an accuracy of 0.33% and 0.10% of the reading, respectively.
In addition to the Li-Cor and weather station meteorological measurements, we made use of daily measurements of PAR and BLH on from the European Centre for Medium-range Weather Forecasting Interim Reanalysis, with a resolution of 0.25°×0.25° (http://apps.ecmwf.int/datasets/data/interim-full-daily/levtype=sfc/). Seidel et al. (Citation2010) reported that uncertainties are greater in shallow boundary layers. BLH estimation also depends on the method of estimation and vertical resolution of the data over the region.
We also computed five-day backward air-mass trajectories using the HYSPLIT model (Draxler & Rolph Citation2003) at altitudes of 1, 2 and 3 km during the study period. Even though trajectory analysis has inherent uncertainties (Stohl et al. Citation1998), it is quite useful in determining long-range transport of air masses and identifying probable sources as well.
Results
Influence and significance of meteorological parameters on CO2 mixing
During the study period, average daily maximum (minimum) of AT, RH, WS and surface pressure were 1.14°C (−8.45°C), 97.65% (48.32%), 25.09 m s−1 (7.41 m s−1) and 1001.81 hPa (967.33 hPa), respectively (). One of the lowest CO2 levels, observed on 30 January 2016, coincided with high RH and high WS (, ). A summary of CO2 distribution under varied environmental conditions at the study site is shown in . When the temperature was positive (>0°C), the median CO2 values were high compared to other temperature bins. An enhancement in CO2 concentration could have been due to an increase in snowmelt (Takagi et al. Citation2005). The median CO2 concentration for 90–100% RH shows a marked contrast in comparison with lower RH levels, possibly due to the scavenging effect of snowfall, which can significantly elevate RH levels (>90%). A similar contrast in the median CO2 concentrations is also observed during instances of relatively low (<20 m s−1) and high (>20 m s−1) WS. The wind direction bin shows high CO2 concentrations when the winds were from the north-west, followed by the north-east, and low concentrations were associated with winds from the east and south-east.
Figure 2. Daily variation of atmospheric CO2 with meteorological parameters (a) AT (°C), (b) RH (%), (c) WS (m s−1) and (d) surface pressure (hPa).

Figure 3. Box and whisker plots of atmospheric CO2 with meteorological parameters (a) AT (°C), (b) RH (%), (c) WS (m s−1) and (d) wind direction (deg.). The lower and upper whiskers shows 5th and 95th percentiles of the data. The lower and upper quartiles of the vertical boxes represent the 25th and 75th percentiles, respectively – together they comprise the middle 50% of the data. The horizontal line in each vertical box indicates the median of the data. Values which are beyond whiskers are outliers. Lowest and highest CO2 values are represented at the 5th and 95th percentiles of the data. (e) Frequency distribution of WS and mean CO2. (f) Precipitation influence on daily mean CO2.

displays hourly averaged CO2 concentration corresponding to each WS bin (bin size 5 m s−1), where it was observed that 90.5% of WSs were in the range of 1.1 m s−1 to 20 m s−1 and the remaining 9.5% were >20 m s−1. During high WS (>20 m s−1), CO2 concentrations decreased with the increase of WS and mean RH. also shows that high precipitation combined with high WS resulted in one of the lowest daily CO2 concentrations recorded during the study period. A low CO2 concentration of 385 ppm was observed during the high-intensity snowfall (precipitation) of 15 mm along with the highest WS and RH of 25 m s−1 and 97%, respectively. The highest daily mean CO2 concentration of 401 ppm was observed on 3 February 2016, with WS about 12 m s−1 and RH of 55%.
PAR and BLH – the two other parameters we took into consideration – are shown in , along with local meteorological observations. Correlation coefficients (r) of CO2 against PAR, surface pressure, AT, BLH, WS, RH and precipitation are summarized in . It is clear that the variability of atmospheric CO2 mixing over Bharati, Antarctica was mainly associated with prevailing meteorological conditions. Statistical analyses of CO2 concentration against PAR, BLH and meteorological parameters show good correlation, but the strongest statistical correlation was with precipitation, with a p value 0.003 (). To further examine the relative influence of meteorological parameters on CO2 concentration, we implemented multiple regression analysis (Benallal et al. Citation2017), as given in Eqn. 1. It is a method used to examine the sensitivity between one dependent variable (Y = CO2) and one or more independent variables Xi (Neter et al. Citation1996; Norman et al. Citation2007). Dueñas et al. (Citation2002) followed a similar approach to assess the influence of meteorological parameters on ozone concentration variations at a (Mediterranean) coastal station.
Table 2. Statistical correlation between CO2 and its influencing parameters at Bharati Station.
Figure 4. Correlation of CO2 with different meteorological parameters at Bharati station, during summer 2016.

The coefficients are estimated using the method of least squares (Bickel & Doksum Citation1997). The results of the multiple regression analysis are given in .
Table 3. Training and results of the least squares multiple regression analysis performed between meteorological parameters and the CO2 mixing ratio (the dependent variable).
Forward selection removes the effect of relatively less significant parameters on CO2 variability compared to highly significant ones. Statistical correlation of CO2 was trained against the independent variables by accepting those p values less than 0.05 and rejecting those with p values greater than 0.10. The independent variables PAR, surface pressure, AT, BLH, WS and RH were removed from this test because of their statistical insignificance. In the short-term analysis, we attempted to establish an empirical relation between the variability of atmospheric CO2 and precipitation at the study site during austral summer, as follows:
where is 396.05 and
is
Though there is no causal connection with CO2 concentration, Eqn. 2 shows that the parameter that most strongly fluctuates along with the CO2 concentrations at the study region is precipitation.
Long-range air-mass influence on local CO2
We computed five-day isentropic backward air-mass trajectories for all the days during the study period. There were six-hour intervals between trajectories, with the first trajectory starting at 00:00 UTC. Trajectories which reached the study site at 3, 2 and 1 km altitudes are shown in –. We separated the trajectory into four clusters based on their pathways: north-east, north-west, south-east and south-west. The majority of air-mass trajectories at 3 and 2 km during study period originated from the north-west (42% and 23%) and north-east (30% and 45%). Air masses coming from the icy Antarctic continent (south-west and south-east of the station) were low compared to their north-easterly and north-westerly components. At 1 km altitude, air masses reaching the study site were from the north-east (47%) and south-east (33%), which was consistent with the surface winds that originated from the north and north-east. Computing differences in CO2 (in %) for each sector from the total mean of the data showed that the maximum positive change was in the north-west (0.76%) sector, followed by the north-east (0.08%) and the south-west (0.06%) (). Easterly wind showed a change of −0.26%. These results indicate that air masses with high CO2 concentration flowed to the study site from across both the Antarctic sea ice and the continent itself.
Discussion and conclusions
In our study of atmospheric CO2 at Bharati Station evaluated against local meteorological parameters statistical analysis showed that metrological conditions – specifically precipitation (snowfall) and RH – were major contributors in variability of atmospheric CO2 concentration. Lowest CO2 values were correlated with high snowfall days, which could be due to a snow scavenging effect at the study site. Studies by Takagi et al. (Citation2005) and Chaubey et al. (Citation2010) also reported the snow scavenging effect on atmospheric species such as CO2 and black carbon in Antarctica.
The air-mass trajectories that we computed showed that air masses with high CO2 concentrations were flowing to Bharati Station from across the Antarctic sea ice and from the continent itself. An average of 396.25 ± 4.20 ppm of CO2 was observed during the study period at the station, with a maximum daily mean CO2 concentration of about 400 ppm. Similar observations were recently made by the British Antarctic Survey and National Oceanic and Atmospheric Administration: an atmospheric CO2 concentration of 400 ppm was a record high concentration that reached the South Pole (Kahn Citation2016), which is the same level as that recorded at Mauna Loa in 2013 (Monastersky Citation2013). CO2 concentrations over Antarctica – the most remote and thinly populated continent – are approaching those of densely populated areas: the annual average CO2 mixing ratios during 2013–15 over the cities of Ahmedabad, India, and Nanjin, China, were 413 ± 13.70 ppm and 406.50 ± 20 ppm, respectively (Huang et al. Citation2016). Monitoring CO2 over Antarctica and other locations around the world continues to be an important activity.
Acknowledgements
We thank the Director of the NRSC, Dr Y.V.N. Krishna Murthy, for supporting the continuation of polar science research at NRSC. We also thank Dr M.V.R. Sesha Sai, Deputy Director of the Earth and Climate Science Area, for his support. The authors sincerely thank the Programme Directors of Logistics and Science at the National Centre for Antarctic and Ocean Research, Goa, and voyage leader Dr Shailendra Saini and Mr Brijesh Desai, both at the same institution, for their support of the data collection. We also gratefully acknowledge the India Meteorological Department for sharing the meteorological data from the study site. We thank Dr Matthew A. Lazzara, Associate Scientist/Meteorologist at Antarctic Meteorological Research Center, for providing the information about radiosonde data collected at McMurdo Station. We also sincerely thank the Subject Editor and anonymous referees for their constructive comments and suggestions, which have certainly helped to improve the quality of the manuscript.
Disclosure statement
No potential conflict of interest was reported by the authors.
Additional information
Funding
References
- Benallal M.A., Moussa H., Lencina-Avila J.M., Touratier F., Goyet C., El Jai M.C., Poisson N. & Poisson A. 2017. Satellite-derived CO2 flux in the surface seawater of the Austral Ocean south of Australia. International Journal of Remote Sensing 38, 1600–8.
- Bickel P. & Doksum K. 1997. Mathematical statistics: basic ideas and selected topics. San Francisco: Holden-Day.
- Brailsford G. (ed.) 2012. 16th WMO/IAEA meeting on carbon dioxide, other greenhouse gases and related tracers measurements techniques (GGMT-2011). GAW report 206. Geneva: Research Department, Atmospheric Research and Environmental Branch, World Meteorological Organization.
- Carvalho J.V.D.S., Mendonça E.D.S., La Scala N., Reis C., Reis E.L. & Schaefer C.E. 2013. CO2-C losses and carbon quality of selected Maritime Antarctic soils. Antarctic Science 25, 11–18.
- Chaubey J.P., Moorthy K.K., Babu S.S., Nair V.S. & Tiwari A. 2010. Black carbon aerosols over coastal Antarctica and its scavenging by snow during the Southern Hemispheric summer. Journal of Geophysical Research—Atmospheres 115, D10210, doi: 10.1029/2009JD013381.
- Cristofanelli P., Calzolari F., Bonafè U., Lanconelli C., Lupi A., Busetto M., Vitale V., Colombo T. & Bonasoni P. 2011. Five‐year analysis of background carbon dioxide and ozone variations during summer seasons at the Mario Zucchelli Station (Antarctica). Tellus B 63, 831–842.
- Draxler R.R. & Rolph G.D. 2003. HYSPLIT (Hybrid Single Particle Lagrangian Integrated Trajectory) model. Silver Spring: National Oceanic and Atmospheric Administration Air Resources Laboratory. Accessed on the internet at http://www.arl.noaa.gov/ready/hysplit4.html on 15 January 2017.
- Dueñas C., Fernández M.C., Cañete S., Carretero J. & Liger E. 2002. Assessment of ozone variations and meteorological effects in an urban area in the Mediterranean coast. The Science of the Total Environment 299, 97–113.
- Gutt J., Zurell D., Bracegridle T.J., Cheung W., Clark M.S., Convey P., Danis B., David B., Broyer C.D., Prisco G., Griffiths H., Laffont R., Peck L.S., Pierrat B., Riddle M., Saucède T., Turner J., Verde C., Wang Z. & Grimm V. 2012. Correlative and dynamic species distribution modelling for ecological predictions in the Antarctic: a cross-disciplinary concept. Polar Research 31, article no. 11091, doi: 10.3402/polar.v31i0.11091.
- Huang J., Yu H., Guan X., Wang G. & Guo R. 2016. Accelerated dryland expansion under climate change. Nature Climate Change 6, 166–171.
- Jones P.D., New M., Parker D.E., Martin S. & Rigor I.G. 1999. Surface air temperature and its changes over the past 150 years. Reviews of Geophysics 37, 173–199.
- Kahn B. 2016. Antarctic CO2 hits 400 ppm for first time in 4 million years. Climate Central, 15 June. Accessed on the internet at http://www.climatecentral.org/news/antarctica-co2-400-ppm-million-years-20451 on 15 February 2017.
- Keeling C.D., Adams J.A., Ekdahl C.A. & Guenther P.R. 1976. Atmospheric carbon dioxide variations at the South Pole. Tellus 28, 552–564.
- Keeling R.F., Piper S.C., Bollenbacher A.F. & Walker J.S. 2008. Atmospheric CO2 records from sites in the SIO air sampling network. Oak Ridge: Carbon Dioxide Information Analysis Center, Oak Ridge National Laboratory, US Department of Energy. Accessed on the internet at http://cdiac.ess-dive.lbl.gov/trends/co2/sio-mlo on 18 January 2017.
- Lower S.K. 1999. Carbonate equilibria in natural waters. A Chem 1 reference text. Burnaby: Simon Fraser University.
- Mahesh P., Sharma N., Dadhwal V.K., Rao P.V.N. & Apparao B.V. 2014. Impact of land–sea breeze and rainfall on CO2 variations at a coastal station. Journal of Earth Science & Climatic Change 5, article no. 201, doi: 10.4172/2157-7617.1000201.
- Mahesh P., Sreenivas G., Rao P.V.N., Dadhwal V.K., Sai Krishna S.V.S. & Mallikarjun K. 2015. High-precision surface-level CO2 and CH4 using off-axis integrated cavity output spectroscopy (OA-ICOS) over Shadnagar, India. International Journal of Remote Sensing 36, 5754–5765.
- Monastersky R. 2013. Global carbon dioxide levels near worrisome milestone. Nature 497, 13–14.
- Neter J., Kutner M.H., Nachtsheim C.J. & Wasserman W. 1996. Applied linear statistical models. 4th edn. Boston: McGraw-Hill.
- Norman G.R., Wyrwich K.W. & Patrick D.L. 2007. The mathematical relationship among different forms of responsiveness coefficients. Quality of Life Research 16, 815–822.
- Schmithüsen H., Notholt J., König‐Langlo G., Lemke P. & Jung T. 2015. How increasing CO2 leads to an increased negative greenhouse effect in Antarctica. Geophysical Research Letters 42, 10422–10428.
- Seidel D.J., Ao C.O. & Li K. 2010. Estimating climatological planetary boundary layer heights from radiosonde observations: comparison of methods and uncertainty analysis. Journal of Geophysical Research—Atmospheres 115, D16113, doi: 10.1029/2009JD013680.
- Sreenivas G., Mahesh P., Subin J., Kanchana A.L., Rao P.V.N. & Dadhwal V.K. 2016. Influence of meteorology and interrelationship with greenhouse gases (CO2 and CH4) at a suburban site of India. Atmospheric Chemistry & Physics 16, 3953–3967.
- Stocker T.F., Qin D., Plattner G.K., Alexander L.V., Allen S.K., Bindoff N.L., Bréon F.M., Church J.A., Cubasch U., Emori S., Forster P., Friedlingstein P., Gillett N., Gregory J.M., Hartmann D.L., Jansen E., Kirtman B., Knutti R., Krishna Kumar K., Lemke P., Marotzke J., Masson-Delmotte V., Meehl G.A., Mokhov I.I., Piao S., Ramaswamy V., Randall D., Rhein M., Rojas M., Sabine C., Shindell D., Talley L.D., Vaughan D.G. & Xie S.P. 2013. Technical summary. In T.F. Stockeret al. (eds.): Climate change. The physical science basis. Contribution of Working Group I to the fifth assessment report of the Intergovernmental Panel on Climate Change. Pp. 33–115. Cambridge: Cambridge University Press.
- Stohl A., Hittenberger M. & Wotawa G. 1998. Validation of the Lagrangian particle dispersion model FLEXPART against large scale tracer experiment data. Atmospheric Environment 32, 4245–4264.
- Sun Y., Bian L., Tang J., Gao Z., Lu C. & Schnell R.C. 2014. CO2 monitoring and background mole fraction at Zhongshan Station, Antarctica. Atmosphere 5, 686–698.
- Takagi K., Nomura M., Ashiya D., Takahashi H., Sasa K., Fujinuma Y., Shibata H., Akibayashi Y. & Koike T. 2005. Dynamic carbon dioxide exchange through snowpack by wind‐driven mass transfer in a conifer–broadleaf mixed forest in northernmost Japan. Global Biogeochemical Cycles 19, GB2012, doi: 10.1029/2004GB002272.
- Turner J. & Overland J. 2009. Contrasting climate change in the two polar regions. Polar Research 28, 146–164.