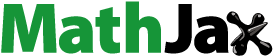
Abstract
This study investigated the effect of Phyllanthus emblica leaves and branches mixture (PE) on growth performance, oxidative status and intestinal characteristics in broilers, as well as the in vitro antioxidant potential of PE extract (PEE). As the result of in vitro evaluation, the PEE had a total phenolic compound content of 95.5 ± 2.75 mg of Gallic acid equivalent/g dry weight (DW), and a total flavonoid content of 3.04 ± 0.06 mg of quercetin equivalent/g DW. The PEE exhibited significant antioxidant activity through various mechanisms, including chelation of ferrous iron, reducing power, scavenging 1, 1-diphenyl-2-picrylhydrazyl (DPPH) radicals, inhibition of liposome peroxidation and trolox equivalent antioxidant capacity (TEAC). A total of 320 Ross 308 broilers were randomly allocated into four groups: control, basal diet supplemented with 0.5%, 1%, 2% PE. Each group was fed for 35 d. The results showed that the group fed with 0.5% PE had significantly better weight gain, and improved feed conversion ratio (FCR) compared to the control group. Moreover, each PE group significantly reduced coliform and Clostridium perfringens in the ileum and caecum. Additionally, PE group with 0.5% concentration had significantly higher villus height in the jejunum compared to the other group. Furthermore, compared to the control group, each PE group had significantly lower levels of malondialdehyde (MDA) and higher levels of superoxide dismutase (SOD), catalase (CAT), and glutathione peroxidase (GPx) activity in the serum. In the peripheral blood mononuclear cells (PBMCs) of chickens, each PE group showed significantly higher mRNA levels of antioxidant-regulated genes, including Nrf2, GST, GCLC, SOD1 and HO-1. In contrast, the expression levels of NOX1 and ROMO1 genes were significantly lower. In conclusion, the inclusion of 0.5% PE in the basal diet could have a positive impact on the growth performance of broilers by modulating intestinal characteristics and oxidative status.
HIGHLIGHT
Phyllanthus emblica affects the growth performance, oxidative status and intestinal characteristics in broilers.
The 0.5% PE group had significantly better weight gain and improved FCR.
The 0.5% PE in the basal diet could potentially enhance growth performance by modulating intestinal characteristics and oxidative status in broilers.
Introduction
Oxidative stress, which disrupts the redox balance of animal cells and causes uncontrolled oxidative damage to animal tissue, is a significant problem for poultry industries worldwide (Akbarian et al. Citation2016; Lin and Lee Citation2021; Lee et al. Citation2022; Liu et al. Citation2022). Oxidative stress is typically initiated by reactive oxygen species (ROS), including superoxide anion (O2−), hydrogen peroxide (H2O2) and hydroxyl radical (•OH) (Ganie et al. Citation2011; Lee et al. Citation2017). ROS take part in numerous physiological processes, such as cellular signalling mechanisms (Droge Citation2002; Liu et al. Citation2008; Lee et al. Citation2019). However, at excessive levels, ROS result in oxidative damage by inducing protein oxidation, lipid peroxidation, and DNA mutations (Bar-Or et al. Citation2015; Lee et al. Citation2020a,Citation2020b). This can lead to reduced immune functions, an elevated risk of suffering from diverse pathological conditions, and metabolic and physiological disorders in poultry (Machlin and Bendich Citation1987; Chen et al. Citation2021). Due to genetic selection for increased body weight, larger breast muscles and faster growth rates, broilers are considered to be particularly susceptible to oxidative stress (Estévez Citation2015). Therefore, the oxidative damage caused by ROS has become an important issue that affects poultry growth (Fellenberg and Speisky Citation2006; Lee et al. Citation2019; Chuang et al. Citation2020).
Phytochemicals, which are non-nutritive compounds derived from plants, are well-known for their antioxidant properties. They help prevent or alleviate oxidative stress by inhibiting the initiation or propagation of oxidative chain reactions (Tachakittirungrod et al. Citation2007; Lee et al. Citation2019; Chuang et al. Citation2021). Cells have developed defense systems to regulate the production of ROS. The nuclear factor erythroid 2-related factor 2 (Nrf2), a redox-sensitive transcription factor, can be activated by many dietary phytochemicals derived from various fruits, vegetables, and herbal medicines (Lee et al. Citation2017; Huang and Lee Citation2018; Chueh et al. Citation2019; Lee et al. Citation2019). Under conditions of oxidative stress, Nrf2 participates in the regulation of non-enzyme antioxidants and a series of phase II detoxification enzymes (Duan et al. Citation2016). Therefore, the Nrf2 pathway plays an important role in upregulating its target antioxidant enzymes and preventing oxidative stress-induced intracellular damage (Chen et al. Citation2012).
Phyllanthus emblica L., commonly known as Emblica officinalis and Indian gooseberry, is a plant belonging to the Euphorbiaceae family. It is widely distributed in tropical and subtropical regions of India, China, Indonesia and Thailand. Accumulated evidence demonstrates that all parts of P. emblica exhibit high phenolic contents and antioxidant capacities (Khan Citation2009; Iamsaard et al. Citation2014). Therefore, this plant, particularly its fruits, has been extensively utilised in various traditional medicinal systems to treat a wide array of diseases. Moreover, the fruits of P. emblica supplemented in broiler feed have been widely investigated in previous studies (Kumar et al. Citation2012; Patil et al. Citation2012; Patel et al. Citation2016; Mandal et al. Citation2017). However, the annual supply of P. emblica fruits is limited due to seasonal factors. The leaves and branches of P. emblica, an attractive new alternative source that contains both antioxidant and antibacterial activities, are low-cost and easy to obtain (Nain et al. Citation2012; Sripanidkulchai and Fangkrathok Citation2014). Little is known about the mode of action of the leaves and branches of P. emblica in broilers. Therefore, the aim of this study is to determine the effects of dietary supplementation of a phytogenic product containing a mixture of P. emblica leaves and branches on the production performance, oxidative status, and intestinal characteristics in broiler chickens.
Materials and methods
Plant collection and extract preparation
The leaves and branches of Phyllanthus emblica were collected from the Miaoli District Agricultural Research and Extension Station. The leaves and branches were collected, dried at 50 °C for 3 h and then ground into powder form using a 0.5-mm sieve before being added to the feed. The weight of the leaves and branches powder was measured, and it was then extracted with distilled water at a temperature of 95 °C for 2 h. The resulting solution was filtered using Whatman® No.1 paper to obtain the PE extract (PEE), which was subsequently stored at −20 °C for in vitro analysis.
Quantification of bioactive compounds
The total phenolic content was determined using the Folin–Ciocalteu reagent, following the method described by Kujala et al. (Citation2000). The 50 μL of diluted PEE was mixed with 0.5 mL of Folin–Ciocalteu reagent (1 N) and 1 mL of sodium carbonate (7.5%). After incubating at room temperature (RT) for 30 min, the absorbance was measured at 730 nm. Total phenolic content in PEE was determined using the equation of the standard gallic acid (GA) graph, expressed in milligrams of the GA equivalent (mg GAE). The flavonoid content was measured using the colorimetric method developed by Pourmorad et al. (Citation2006). Briefly, the 0.5 mL diluted PEE was added to the reaction mixture consisting of methanol (1.5 mL), 10% aluminium chloride (0.1 mL), 1 M potassium acetate (0.1 mL) and 2.8 mL of distilled water, and kept at RT for 30 min. The absorbance of the reaction mixture was measured at 415 nm using a spectrophotometer. The flavonoid content was estimated by preparing quercetin solutions and calculating the calibration curve. The results were expressed in milligrams of quercetin equivalent (mg QE). The analysis of high-performance liquid chromatography (HPLC) was conducted using an HPLC instrument (HITACHI, Kyoto, Japan) equipped with a UV detector (L-2490), a column (Transgenomic CARBOSep CH0682 Pb, 300 mm × 7.8 mm), a pump (L- 2130), and a computer system with HPLC D-2000 Elite. The lyophilised PEE was dissolved in a mixture of methanol and water (2:8) and then filtered through a 0.22-μm membrane filter. Five reference standards, including gallic acid (GA), ellagic acid (EA), rutin (RU) and quercetin (QU) (Sigma, USA), were analysed at their respective wavelengths. The sample and standards injection volume was 20 μL. Under the determination of UV spectra at specific wavelengths, the chromatographic peak and retention time in the samples were compared with the reference standards. Quantification was carried out by measuring the integrated peak areas of the sample and using related standard curves.
Ferrous chelating capacity assay
The ferrous chelating capacity assay was determined according to the method described by Dinis et al. (Citation1994). The diluted PEE (0.25 mL), ferrous chloride tetrahydrate (0.025 mL, 2 mM), and methane (0.925 mL) were mixed. After incubating at RT for 30 s, the 0.05 mL of ferrozine (5 mM) was added. The mixture was left to stand at RT for 10 s, and the absorbance of the reaction mixture was measured at 415 nm using a spectrophotometer. The percentage of inhibition of ferrozine-Fe2+ complex formation was calculated using the following formula:
where A0 and A1 represent the absorbance of the control and samples (PEE and EDTA), respectively. The control was determined by replacing the sample with methanol. In this experiment, EDTA was used as a positive control.
Reducing power assay
The reducing power was assayed using the method developed by Oyaizu (Citation1986). The 2.5 mL of diluted PEE was mixed with 2.5 mL of phosphate buffer (0.2 M, pH 6.6), and 2.5 mL of potassium ferricyanide (1%). After incubating at 50 °C for 20 min, the reaction was terminated by adding 2.5 mL of 10% trichloroacetic acid. The 5 mL upper layer of the solution was then mixed with 5 mL of distilled water and 1 mL of ferric trichloride (0.1%). The mixture was incubated at RT for 10 s, and the absorbance of the reaction mixture was measured at 700 nm using a spectrophotometer. Butylated hydroxytoluene (BHT) and ascorbic acid (VitC) were used as positive controls. Increased absorbance of the reaction mixture indicates a higher level of reducing power.
1, 1-Diphenyl-2-picrylhydrazyl) free radical scavenging capacity assay
The ability of 1-diphenyl-2-picrylhydrazyl (DPPH) radical scavenging was investigated using the method described by Blois (Citation1958). 1 mL of diluted PEE was added to 0.5 mL of DPPH (1 mM). The reaction mixture was incubated at RT in darkness for 30 min, and the absorbance was measured at 517 nm using a spectrophotometer. Decreased absorbance of the reaction mixture indicates a higher scavenging ability. The scavenging effect on DPPH was calculated using the following equation:
where A0 and A1 represent the absorbance of the control and samples (PEE and BHT), respectively. The control was determined by replacing the sample with methanol. In this experiment, BHT was used as a positive control.
Liposome peroxidation inhibiting ability assay
The inhibitory ability of liposome oxidation was determined and modified following the method described by Duh et al. (Citation1999). Above all, 2 g of lecithin was added to 200 mL of phosphate buffer (20 mM, pH 7.4), and the solution was thoroughly emulsified using an ultrasonic cleaner. The 0.5 mL diluted PEE was added to the reaction mixture, which consisted of the emulsified lecithin solution (0.5 mL, 10 mg lecithin/mL), 25 mM ferric trichloride (0.1 mL), 25 mM ascorbic acid (0.1 mL), and 1.2 mL of phosphate buffer (20 mM, pH 7.4). After incubating at 37 °C for 2 h, the solution was mixed with 1 mL of BHT (20 mg/mL), 2 mL of TBA (1%), and 1 mL of TCA (2.8%). The mixture was left to stand at 100 °C for 30 min, and then centrifuged (12,000 x rpm, 10 min). The supernatant fluid was collected and its absorbance was measured at 532 nm using a spectrophotometer. The inhibition rate of liposome oxidation can be calculated using the following equation:
where A0 and A1 represent the absorbance of the control and samples (PEE, BHT and VitC), respectively. The control was determined by replacing the sample with distilled water. In this experiment, BHT and VitC were used as positive controls.
Trolox equivalent antioxidant capacity assay
The Trolox equivalent antioxidant capacity (TEAC) was determined using the method described by Arnao et al. (Citation1996). Above all, the TEAC reagent was prepared by mixing ABTS (1,000 µM), H2O2 (500 µM), peroxidase (44 U/ml) and distilled water at a ratio of 1:1:1:6. The TEAC reagent was reacted at RT in the dark for 1 h before use. The 0.1 mL diluted PEE was then mixed with 0.9 mL of TEAC reagent. The reaction mixture was allowed to stand at RT in darkness for 10 min, and the absorbance was measured at 734 nm using a spectrophotometer. Trolox, a water-soluble analogue of vitamin E, was used to generate a standard curve at different concentrations. The scavenging ability of PEE was calculated by comparing it to the standard curve. The results were calculated corresponding to the concentrations of BHT and VitC, expressed in micrograms (µg) of Trolox antioxidant equivalent per millilitre.
Experimental birds and housing
The experiment was conducted at National Chung Hsing University, Taiwan, and the experimental protocol for animal use was approved by the Animal Care and Use Committee. A total of 320 1-day-old male broiler chicks (Ross 308) were evenly divided by weight (approximately 48.0 to 49.0 g/birds) and randomly allocated to one of four treatments. Each treatment had four replicates, with 20 birds per pen, resulting in a total of 80 birds per treatment. All the birds were placed in a temperature-controlled house. The temperature was maintained at 33 ± 1 °C until the birds reached 7 d of age, and it was gradually decreased to 27 ± 1 °C until the birds reached 21 d of age. After this point, the broilers were kept at approximately 27 °C.
Feeding schedule and dietary composition
The experiment lasted for 35 d, divided into 2 phases: the starter phase (1 to 21 d) and the finisher phase (22 to 35 d). Diets (in mash form) and water were provided ad libitum. The birds in the control group were fed basal diets consisting of corn-soybean meal. The other three groups were given experimental diets, which were based on the basal diet and supplemented with an additional 0.5% PE, 1% PE and 2% PE (Table ). The proximate composition was analysed according to the Association of Official Analytical Chemists (AOAC) (Citation2000). Crude protein, crude fat, ash and acid detergent fibre levels were determined using methods 990.03 (Kjeldahl N × 6.25), 945.16, 967.05 and 973.187, respectively. The results showed no significant deviations from the calculated values. During the entire experimental period (35 d), the diets were prepared using the same batch of ingredients and formulated to meet the requirements suggested by the Ross Broiler Management Manual (2014) and the NRC (Citation1994).
Table 1. Ingredients and chemical composition (g/kg as fed) of the experimental diets for broilersTable Footnote1.
Performance, serum, plasma and intestinal content collection
Body weight of chickens per pen and feed consumption were recorded at 1, 21, and 35 d of age. Body weight gain and feed conversion ratio (FCR) were calculated based on this data. At 35 d of age, sixteen birds per treatment group (four birds per replicate) were randomly selected for sampling. Blood samples were collected (5 mL) by puncturing the wing vein using a vacutainer tube. The blood was then centrifuged at 3,000 × g for 10 min to obtain the supernatant fluid containing serum. The serum was transferred into sterilised eppendorf tubes and then stored at −20 °C until it was analysed for antioxidant enzyme activity and malondialdehyde (MDA) levels. Blood samples were collected (5 mL) via wing-vein puncture into a tube containing 1% EDTA. The blood was then prepared for the isolation of chicken peripheral blood mononuclear cells (cPBMCs). After the blood was collected, the birds were euthanized through exsanguination and dissected to obtain the gastrointestinal tract. The contents of the ileum and caecum were collected in sterile plastic plates and promptly placed on ice for further analysis. The samples of intestinal segments (3 cm) were also collected from the jejunum and ileum regions. After the flushing process, the intestinal segments were fixed in 10% formalin for subsequent morphological assays.
Microbial populations in ileal and caecal contents
Strains of lactic acid bacteria, coliform and Clostridium perfringens were cultured using specific media. The lactic acid bacteria were cultured on MRS medium (de Man Rogosa and Sharpe agar, Difco 288130), the coliform bacteria were cultured on CCA medium (Chromocult® coliform agar, Merck) and the Clostridium perfringens were cultured on TSC agar (tryptose sulphite cycloserine agar, Difco TM, BD, Franklin Lakes, NJ). Lactic acid bacteria and Clostridium perfringens were incubated under anaerobic conditions, while coliforms were incubated under aerobic conditions. After incubating at 37 °C for 48 h, the microbial counts were calculated. Bacterial populations were expressed as log10 colony-forming units (CFU) per gram of intestinal contents.
Morphometric analysis of the small intestine
After being washed in distilled water, the formalin-fixed intestinal tissues were embedded in paraffin wax. The tissues were then sectioned at 3 μm and stained using the haematoxylin and eosin staining methods. Histological sections were examined using a light microscope equipped with a Motic Image Plus 2.0 analysis system. Images were viewed at 4× magnification to measure morphometric parameters of intestinal architecture. Villus height was measured by using the vertical distance from the tip of the villus to the villus crypt junction. Crypt depth is determined by the depth of the invagination between adjacent villi. The ratio of villus height to crypt depth was also calculated.
Serum Biochemical determination
MDA content and the activities of SOD, CAT and GSH-Px were measured using assay kits purchased from Cayman Chemical Co., Ltd. The MDA content was expressed in micromolar (µM), while the antioxidant enzyme activities were expressed as units (U) per millilitre of serum.
Peripheral blood mononuclear cell Isolation
The plasma was layered on Ficoll® Paque Plus and centrifuged at 200 × g for 10 min. Chicken peripheral blood mononuclear cells (cPBMCs) were collected from the gradient interface, washed three times with PBS, and centrifuged for 10 min at 200 × g. After removing the suspension, the cell count was adjusted to 108 cells/mL with PBS. Pipetted and transferred into a sterilised tube, cPBMCs were centrifuged for 10 min at 200 × g and then the suspension was removed. 1 mL of Trizol reagent (Invitrogen, Waltham, Massachusetts) was added, and the mixture was stored at −80 °C.
RNA Isolation and quantitative reverse transcription–polymerase chain reaction
The Trizol mixture was extracted with chloroform and then precipitated using isopropanol. The RNA pellet was washed once with 75% ethanol, dried for 10 min, and dissolved in RNase-free water. Determined by spectrophotometry, the RNA concentration was diluted to 50 ng/µL. Total RNA concentration and purity, cDNA synthesis, and qPCR analysis were determined following the method described by Lin et al. (Citation2014). The designs of gene-specific primers were determined based on the genes of Gallus gallus (chickens). Table lists the features of the primer pairs. After normalising the gene expression data using the calculated GeNorm normalisation factor, the means and standard deviations (SDs) were calculated from the normalised expression data for samples with the same treatments.
Table 2. Characteristics and performance data of the primers used for qPCR analysis.
Statistical analyses
Data were subjected to ANOVAs as a completely randomised design using the GLM procedure of the SAS software (SAS, Citation2004). The determination of significant statistical differences among the mean values of the four treatment groups was conducted using Tukey’s honestly significant difference test with a significance level of p < 0.05.
Results
Bioactive compounds in PE
Table shows the concentration of bioactive compounds in PE. The total phenolic compound content was 95.5 ± 2.75 mg of GAE/g DW, while the total flavonoid content was 3.04 ± 0.06 mg of QE/g DW. This table also presents the concentration of specific phenolic compounds. The gallic acid, ellagic acid, rutin and quercetin contents of PE were 21.7 ± 0.46, 13.6 ± 0.78, 3.16 ± 0.04 and 2.17 ± 0.08 mg/g DW, respectively.
Table 3. Chemical composition and content of the bioactive compounds of Phyllanthus emblica leaves and branches mixtureTable Footnote1.
Ferrous iron chelating capacity
The capacity of chelating ferrous iron by the PEE is presented in Figure . Compared to EDTA, PEE demonstrated an equivalent chelating effect of 99.9% at concentrations ranging from 0.78,125 to 25.0 mg/mL. At a concentration of 25 mg/mL, PEE exhibited a 47.3% equivalent chelating effect.
Reducing power
Figure illustrates the ferrous iron chelating capacity of the PEE. BHT, VitC and PE reached their maximum reducing power at a concentration of 0.5 mg/mL. There was no observed increase in reducing power as the concentration increased. At a concentration of 1 mg/mL, the highest reducing power of PE was approximately equivalent to 85.3% of VitC and 91.5% of BHT.
DPPH radical scavenging capacity
The capacity of DPPH Radical Scavenging of the PEE is shown in Figure . The PEE was capable of neutralising the DPPH free radicals through hydrogen donation activity at concentrations of 0.03,125, 0.0,625, 0.125, 0.25, 0.5, 1 and 2 mg/ml. The percentages of neutralisation were 18.3%, 20.2%, 28.3%, 42.1%, 45.7%, 49.9%, and 51.0%, respectively. At a concentration of 2 mg/mL, the scavenging capacity of the PEE was approximately identical to 54.92% of BHT.
Inhibition of liposome peroxidation
Figure represents the response curve of the PEE on the percentage inhibition of lipid peroxidation. The inhibitory ability of the PEE was 72.6% at a concentration of 20 mg/mL. In comparison, the inhibitory abilities of BHT and VitC at the same concentration were 86.5% and 79.5%, respectively.
Trolox equivalent antioxidant capacity (TEAC)
Table illustrates the TEAC value of the PEE. The TEAC of PEE at 250 µg/mL was approximately equivalent to BHT and VitC at 80 and 60 µg/mL, respectively. The TEAC of PEE at 200 µg/mL was approximately equivalent to BHT and VitC at 40 and 50 µg/mL, respectively. Lastly, the TEAC of PEE at 50 µg/mL was approximately equivalent to BHT and VitC at 10 and 20 µg/mL, respectively.
Table 4. Trolox equivalent antioxidant capacity (TEAC) of Phyllanthus emblica leaves and branches mixture aqueous extracts, Butylated hydroxytoluene and ascorbic acid.
Growth performance
Table shows the effect of supplementing PE in diets on the growth performance of broilers aged 1 to 35 days. The inclusion of 0.5% PE in diets resulted in increased weight gain and improved feed conversion ratio (FCR) (p < 0.05) during the starter phase (0–21 d), finisher phase (22–35 d) and overall study period (0–35 d). Moreover, during the entire study period, birds fed 1% PE exhibited significantly better FCR (p < 0.05) compared to birds fed control diets. However, there were significant decreases in body weight and weight gain in birds fed diets containing 2% PE (p < 0.05).
Table 5. Effect of Phyllanthus emblica leaves and branches mixture on growth performance of broilers.
Microbial population in ileum and ceca
Table shows the effect of supplementing PE in diets on the microbial population in the broiler ileum and caeca after 35 d. There were no significant differences in the lactic acid bacteria in the ileum and caeca among all treatments. As for the treatments added with PE, coliform and Clostridium perfringens counts were significantly lower than those in the control group (p < 0.05).
Table 6. Effect of Phyllanthus emblica leaves and branches mixture supplemented in diet on lactic acid bacteria, coliform and Clostridium perfringens count in intestinal content of 35-day-old broilersTable Footnote1.
Intestinal morphology
The effects of dietary supplementation of PE in diets on the intestinal morphology of broilers after 35 d are shown in Figure and Table . Birds fed diets supplemented with 0.5%, 1%, and 2% PE showed a significant increase in villus height and villus height to crypt depth ratio, along with a significant decrease in crypt depth in the jejunum (p < 0.05). Moreover, the inclusion of 0.5% PE in the diets resulted in higher villus height and villus height to crypt depth ratio compared to the 1% and 2% PE treatments (p < 0.05). Diets supplemented with PE significantly decreased crypt depth and increased the villus height to crypt depth ratio in the ileum compared to the control group (p < 0.05). Among the different groups, the addition of 0.5% PE in the diets resulted in the lowest crypt depth and the highest villus height to crypt depth ratio (p < 0.05).
Figure 2. Photomicrography of jejunum and ileum of 35d broiler fed with control and PE. (A) Control, jejunum (B) 0.5% PE, jejunum (C) 1% PE, jejunum (D) 2% PE, jejunum (E) Control, ileum (F) 0.5% PE, ileum (G) 1% PE, ileum (H) 2% PE, ileum. PE: Phyllanthus emblica leaves and branches mixture. Haematoxylin and eosin stain (40×) (method according to Tsai et al. (Citation2021)).

Table 7. Effect of Phyllanthus emblica (PE) leaves and branches mixture supplemented in diet on intestinal morphology of 35-day-old broilersTable Footnote1.
Serum antioxidant enzyme and MDA value assessments
Table presents the impact of PE supplementation in broiler diets on serum antioxidant enzyme activities and MDA values after 35 d. The results indicate that birds fed diets with 0.5%, 1%, and 2% PE exhibited significantly lower serum MDA values and higher serum SOD, CAT and GSH-Px activities compared to the control group (p < 0.05).
Table 8. Effects of Phyllanthus emblica (PE) leaves and branches mixture supplemented in diets on the serum antioxidant enzymes activities and MDA levels of 35-day-old broilersTable Footnote1.
Relative mRNA concentration of antioxidant-regulated genes in chicken PBMCs
Figure illustrates the impact of PE supplementation in broiler diets on the expression levels of antioxidant response-related transcription factors in chicken PBMCs after 35 d. In comparison to the control group, the birds fed 0.5%, 1%, and 2% PE exhibited significantly lower expression levels of NOX1 and ROMO1. Conversely, the expression levels of Nrf2, GST, GCLC, SOD1 and HO-1 were significantly higher (p < 0.05). Moreover, diets supplemented with 0.5 and 1% PE exhibited the highest expression levels of GCLC, SOD1 and HO-1 compared to the other groups (p < 0.05).
Discussion
Content of the bioactive compounds
A strong and positive correlation between the total phenolic and flavonoid content of natural herbs and their antioxidant capacity has been found in previous studies (Djeridane et al. Citation2006; Wan et al. Citation2016; Su et al. Citation2020; Tsai et al. Citation2022). This correlation also implies that phenolic and flavonoid compounds were the main and important antioxidant components. The total phenolic and flavonoid contents of PEE in this study were 95.5 mg GAE/g and 3.04 mg QE/g, respectively. Iamsaard et al. (Citation2014) reported that aqueous extracts of P. emblica leaves and branches contained 514 and 651 mg GAE/g, respectively. The variation in total phenolic contents observed among researchers can be attributed to various factors such as the source and parts of the herb, temperature, season, genetics, and agronomic factors. Additionally, differences in methods, solvents, and extraction parameters such as temperature and time may also contribute to the variation (Hilton and Palmer-Jones Citation1973; Zheng and Wang Citation2001). The presence of phenolic and flavonoid compounds, such as GA, EA, RU and QU) in PEE was confirmed through HPLC analysis, which is consistent with previous studies (Khan Citation2009; Chaiittianan and Sripanidkulchai, Citation2014; Tahir et al. Citation2016). Moreover, GA and EA were the major bioactive compounds found in PE (Variya et al. Citation2016). Additionally, previous studies have conducted phytochemical analysis of PE, which indicated that PE contains higher amounts of hydrolysable tannins. These tannins are classified into gallotannins (esters of GA) and ellagitannins (esters of EA), and they exhibit biological properties such as antioxidant and antimicrobial activities (Zhang et al. Citation2001; Citation2004; Khan Citation2009; Yang and Liu Citation2014).
In vitro antioxidant activity
Ferrous ions can react with H2O2 through the Fenton reaction, resulting in the production of hydroxyl radicals (•OH). Hydroxyl radical, which is a much stronger oxidant than the superoxide anion (O2−), causes oxidative damage to DNA and serves as the initiator of lipid peroxidation (Ayala et al. Citation2014). Additionally, the ferrous ion also decomposes lipid hydroperoxides into lipid peroxyl and alkoxyl radicals, thereby attacking other lipids and accelerating the propagation of lipid peroxidation (Valko et al. Citation2006). Cellular membrane structures were destroyed by lipid peroxidation (Rhee et al. Citation1996). This oxidative destruction causes cell death, tissue injuries and diseases (Esterbauer et al. Citation1991). Therefore, the capacity of metal chelation, reducing power, free radical scavenging and inhibition of lipid peroxidation are indicators of the antioxidant activity of plant materials. In the present study, the antioxidant properties were evaluated in terms of metal chelating capacity (Figure ), reducing power (Figure ), free radical scavenging capacity (Figure ), inhibitory ability in a lipid peroxidation chain reaction (Figure ), and TEAC (Table ). The results demonstrated that the PEE contained phenolic and flavonoid components, including GA, EA, RU, and QU, which potentially exerted in vitro antioxidant activities. Stoilova et al. (Citation2007) reported that plant extracts exhibit antioxidant activity due to their polyphenol components, including phenolic acids, flavonoids, and tannins. These components act as donors of hydrogen atoms or electrons and have the ability to capture free radicals. Moreover, polyphenol components possess Fe2+ chelating activity and protect against the initiation and propagation of free radical-mediated lipid peroxidation (Poltanov et al. Citation2009; Kaur et al. Citation2012).
Growth performance
The beneficial factors of growth performance in animals fed herbal extracts or polyphenol substances include improvements in appetite and feed intake, intestinal microbiota, morphology of the gastrointestinal tract, digestibility and absorption of nutrients, regulation of oxidant status, and immune responses (Rahimi et al. Citation2011; Costa et al. Citation2013; Qin and Hou Citation2017; Su et al. Citation2020; Tsai et al. Citation2022). In our study, the addition of 0.5% PE to the diet resulted in improved weight gain and FCR compared to the control group. This suggests that PE may modulate intestinal function and oxidant status, thereby stimulating growth in broilers. To date, there have been no references in the publications regarding the inclusion of P. emblica leaves and branches in broiler diets. However, previous studies have investigated the fruits of P. emblica, which are also rich in GA and EA. Patel et al. (Citation2016) reported that basal diets supplemented with 0.4% and 0.8% P. emblica fruits had a positive effect on the growth performance of broiler chickens. P. emblica fruits in broiler diets were beneficial in improving FCR during the extreme summer period (Mandal et al. Citation2017). In contrast, decreased tendencies in feed intake and weight gain were found in the 2% PE group, possibly due to the presence of tannins mentioned in PE. Tannins, as anti-nutritional factors in poultry nutrition, are primarily associated with high concentrations of tannins in diets. This not only results in a bitter or astringent taste in the feed but also reduces feed palatability, feed efficiency, growth rate, and the digestibility of proteins and minerals (Jansman Citation1993).
Physiological function of polyphenols
The bioavailability of dietary polyphenols is closely related to the modulation of intestinal health and antioxidant status in animals (Lee et al. Citation2017; Citation2019; Lin and Lee Citation2020; Liu et al. Citation2022). The absorption of polyphenols in the monogastric animal intestine was determined by the chemical structure of the polyphenols (Lee et al. Citation2017). After consuming dietary polyphenols, low-molecular-weight polyphenols with monomeric or dimeric structures (e.g. GA and EA) can be released from the food matrix through direct solubilisation in the intestinal fluids or the action of digestive enzymes. These polyphenols are then absorbed in the small intestine (Saura-Calixto, Citation2011). Polymeric polyphenols, such as tannins, and low-molecular-weight polyphenols that are associated with dietary fibre, cannot be dissolved and absorbed in the small intestine. Instead, they reach the hindgut, where bacterial enzymes can ferment them (Saura-Calixto et al. Citation2007). Furthermore, dietary fibre matrices are degraded, and the original structures are extensively broken down into low-molecular-weight polyphenols, which are absorbed in the hindgut. These absorbable polyphenols and unabsorbed dietary polyphenols in the intestine play an important role in modulating the intestinal ecology and suppressing pathogens. They also have antioxidant properties, which improve intestinal health (Saura-Calixto, Citation2011). After being absorbed by gut epithelial cells, low-molecular-weight polyphenols are transformed via the portal vein into the liver, where they undergo conjugation reactions (methylation, glucuronidation, and sulfation). These conjugate derivatives are then rapidly released into the plasma and distributed to cells and tissues through systemic circulation (Manach et al. Citation2004). Consequently, the antioxidant capacities in the animal body are enhanced.
Microbial populations in ileal and caecal contents
Phytogenic products are natural and well-known for their antibacterial activities (Windisch et al. Citation2008). As the principal active substances, polyphenol components are often mentioned for their antibacterial properties against pathogens (Singh et al. Citation2016). The mechanism of action for the antimicrobial activity of polyphenol compounds is based on their strong interaction with bacterial lipid membranes (Sun et al. Citation2009; Yu et al. Citation2011). With their partially lipophilic nature, polyphenols are believed to interact effectively with bacterial lipid membrane and lipopolysaccharide interfaces. As a result, they can passively diffuse across the cell membrane, causing destruction and acidification of the membrane (Xu et al. Citation2014). This phenomenon causes not only the leakage of cell constituents, leading to the irretrievable loss of proteins, nucleic acids, and inorganic ions such as K+, but also the inhibition of efflux pumps and enzymes (Campos et al. Citation2009; Borges et al. Citation2013). In this study, the reduction of coliform and Clostridium perfringens in the ileum and caecum of each PE group, compared to the control group, aligns with the findings of other studies that investigated the effects of different phytogenic products on broiler chickens’ diets (Guo et al. Citation2004; Jamroz et al. Citation2005; Murugesan et al. Citation2015). Similarly, PE has been shown to have in vitro antimicrobial efficacy against E. coli (Nain et al. Citation2012; Sripanidkulchai and Fangkrathok Citation2014). Gut microflora has a significant effect on gut health, thereby impacting host nutrition, health, and growth performance (Barrow Citation1992). Therefore, the reduction of pathogens in the gut has a positive effect on the growth performance of broilers, as was found in the 0.5% PE group.
Intestinal morphology
Lengthened villi height increases body weight gain (Zijlstra et al. Citation1996) and improves gut health status by increasing the total luminal villus absorptive area capable of expression of brush border enzymes and higher absorption of available nutrients in the villus surface (Caspary, Citation1992). The crypt is viewed as a villus factory; furthermore, a deeper crypt indicates faster tissue turnover to arouse renewal of the villus, which suggests that the gut was under inflammation from toxins and demanding high energy to repair the sloughing or atrophy of villi (Yason et al. Citation1987). Therefore, changes in intestinal morphology may influence nutrient absorption and gut health, thereby affecting the growth performance of animals. In the present study, we found that dietary supplementation with 0.5% PE had greater villus height/crypt depth than other groups in the jejunum, as well as the greatest villus height/crypt depth found in the 0.5 and 1% PE groups in the ileum, which closely corresponded during the overall period with the enhanced growth performance and feed efficiency obtained in the 0.5% PE group and the enhanced feed efficiency obtained in the 1% PE group. Our results are in line with the previous findings of Viveros et al. (Citation2011), who reported that dietary polyphenol-rich grape pomace concentrate (60 g/kg) could ameliorate the gut morphology (villus height: crypt depth) and increase the feed efficiency of broiler chicks. In addition, gut integrity and function show a direct correlation with pathogens attaching to the mucosa (Droleskey et al. Citation1994). The increased counts of pathogenic bacteria in the gastrointestinal tract led to a shorter villus and a deeper crypt (Cook and Bird Citation1973). Our previous study showed that mucosal architecture, in terms of villus height/crypt depth was improved by decreased populations of coliform and Clostridium perfringens in the ileum. In a review, phytogenic products can stimulate mucus secretion in the stomach and intestine, thereby preventing the adhesion of pathogens, stabilising favourable microbiota, protecting the intestinal villi and improving the digestion and absorption of nutrients (Costa et al. Citation2013).
Serum biochemical determination
Oxidative stress caused by excessive levels of ROS leads to cellular oxidative imbalance and critical changes to cellular structure and function (McCord and Edeas Citation2005; Chen et al. Citation2021). Therefore, to limit the potential toxicity of ROS, cells develop non-enzymatic and enzymatic antioxidant systems. Ji (Citation2007) reported that enzymatic antioxidants are essential indicators of ROS production and also serve as a key to maintaining redox balance and cell functionality. The three main enzymatic antioxidants involved in scavenging oxygen free radicals are SOD, GPx and CAT (McCord Citation1979). First, SOD catalyses the dismutation of O2− to H2O2 (Liu and Mori Citation1993). Thereafter, GPx and CAT detoxify the H2O2 produced into H2O (Weiss Citation1986; Jaeschke Citation1995). •OH generated from H2O2 by the Fenton reaction initiates lipid peroxidation, generating lipid hydroperoxides that are converted to aldehyde products such as MDA (Muralikrishna and Hatcher Citation2006). Therefore, increasing the activities of SOD, GPx and CAT consistent with reduced MDA concentration shows the capacity of broilers to scavenge the ROS and prevent the propagation of lipid peroxidation. Furthermore, the activities of these antioxidant enzymes require a dietary supply of the appropriate antioxidants to maintain the redox balance (Aluwong et al. Citation2013). Zhang et al. (Citation2009) observed that supplementation of ginger, which is full of polyphenol components, in broiler diets significantly increased SOD and GPx activity and lowered MDA in serum. Wang et al. (Citation2016) also reported that the intake of Artemisia annua L. leaves enhanced SOD, CAT and GPx activities and reduced MDA concentrations in serum due to the phenolic compounds and flavonoids found in Artemisia annua L. leaves. Similar to other studies, our animal experiment demonstrated that PE supplementation in basal diets improves the antioxidant status of broiler chickens by means of positive changes in serum enzymatic antioxidants and MDA levels. Therefore, the improved antioxidant status of broilers in PE groups observed in this study could partially be attributed to the antioxidant compounds in PE.
Relative mRNA concentration of antioxidant-regulated genes in chicken PBMCs
The cells develop various mechanisms for controlling and neutralising the deleterious effects of ROS to maintain redox homeostasis. Romo1 is a protein that induces mitochondrial ROS production; furthermore, Romo1 acts as a modulator to transfer ROS into the cytosol (Chung et al. Citation2006). Increased expression of ROMO1 not only elevates intracellular ROS levels, but also causes nuclear DNA damage and the proliferation of cancer cells (Na et al. Citation2008). Therefore, Romo1 is a crucial regulator of intracellular ROS production induced by oxidative stress. NOX1, one of the NADPH oxidase families, has the function of regulating intracellular ROS production through catalysing O2, using NADPH as an electron donor, thus producing O2− (Spencer and Engelhardt Citation2014). Upregulated expression of NOX1 increases oxidative stress and causes cardiovascular disease (Brown and Griendling Citation2015). In our study, the significantly lower ROMO1 and NOX1 expressions in all PE groups demonstrated reduced ROS in broiler chickens. In contrast, under normal conditions, Nrf2 is sequestered in the cytoplasm by associating with a protein, namely kelch-like ECH-associated protein 1 (Keap1) (McMahon et al. Citation2006). The Keap1 protein, as an inhibitor for inactivating Nrf2, regulates the stability of Nrf2 (Sun et al. Citation2007). Upon recognition of ROS or electrophilic molecules, Nrf2 dissociates from Keap1 and translocates into the nucleus, furthermore, reacting with antioxidant response element (ARE) and triggering expression of cytoprotective genes, phase II detoxifying enzyme genes, such as GST and HO-1, and antioxidant enzymes, such as GCLC and SOD1 (Niforou et al. Citation2014). Thus, the regulation of the Nrf2 pathway is regarded as a critical step in initiating the antioxidant response and preventing cells from being damaged by ROS (Copple et al. Citation2008). GCLC is the catalytic form of glutamate-cysteine ligase (GCL), which serves as the crucial and rate-limiting enzyme in glutathione (GSH) synthesis. GSH, a non-enzymatic antioxidant, can directly convert H2O2 to non-toxic H2O under catalysis by GPx, thereby protecting against oxidative stress (Kachadourian et al. Citation2011). Moreover, GST can be conjugated with GSH, playing an important role in the detoxification of oxidative compounds (Espinosa-Diez et al. Citation2015). In our study, the higher GCLC and GST expressions in all PE groups showed the potential for maintaining redox balance and ameliorating oxidative stress injury. CuZnSOD (SOD1) is one of the three isoforms of the SOD enzyme, located mainly in the cytosol. Additionally, ROS levels, especially O2−, correspond with changes in cytosolic SOD1 activity or expression (Espinosa-Diez et al. Citation2015). In this study, the higher expression of SOD1 found in PE groups is consistent with the serum SOD activity curve. HO-1 is an inducible enzyme that can catalyse prooxidant haem degradation, thus generating carbon monoxide (CO) and biliverdin (Choi and Alam Citation1996). CO as well as bilirubin, the product of biliverdin, have potent antioxidant and anti-inflammatory properties (Naidu et al. Citation2009). Consequently, increased HO-1 expression in all PE groups exerts potential cytoprotective activity. Similarly, Lin et al. (Citation2017) reported that supplementation of mulberry leaves in laying hens’ diets significantly lowers NOX1 and ROMO1 mRNA expression, while increasing Nrf2, HO-1 and GST mRNA expression, which has a similar positive effect in agreement with our study.
In conclusion, broilers fed diet supplemented with 0.5% PE show enhanced growth performance by reducing coliform and Clostridium perfringens in the ileum and caecum, increased villus height/crypt depth ratio in the jejunum or ileum, increased antioxidant enzyme activities, and decreased MDA concentration in the serum, furthermore exerting antioxidant effects by activating the Nrf2-ARE-mediated antioxidative response.
Authors’ contributions
Conceptualisation, T. T. Lee and H. T. Zheng; materials analysis, T. T. Lee and C. H. Shih; methodology, T. T. Lee and S. C. Chang; supervision, T. T. Lee; investigation, T. T. Lee and L. J. Lin; writing—original draft preparation, T. T. Lee and H. T. Zheng; writing—review and editing, T. T. Lee, L. J. Lin. and S. C. Chang.
Acknowledgments
The authors would like to thank colleagues in the Ministry of Science and Technology (MSTC 112-2313-B-005-043-MY3; 111-2634-F-005-001 project Smart Sustainable New Agriculture Research Center (SMARTer) and the iEGG and Animal Biotechnology Center from The Feature Areas Research Center Program within the framework of the Higher Education Sprout Project by the Ministry of Education (MOE -112-S-0023-A) in Taiwan for supporting this study.
Disclosure statement
No potential conflict of interest was reported by the authors.
Data availability statement
The data presented in this study are available on request from the corresponding author upon reasonable request
References
- Akbarian A, Michiels J, Degroote J, Majdeddin M, Golian A, Smet SD. 2016. Association between heat stress and oxidative stress in poultry; mitochondrial dysfunction and dietary interventions with phytochemicals. J Anim Sci Biotechno. 7:1–14.
- Aluwong T, Kawu M, Raji M, Dzenda T, Govwang F, Sinkalu V, Ayo J. 2013. Effect of yeast probiotic on growth, antioxidant enzyme activities and malondialdehyde concentration of broiler chickens. Antioxidants (Basel). 2(4):326–339. doi: 10.3390/antiox2040326.
- Arnao MB, Cano A, Hernández-Ruiz J, García-Cánovas F, Acosta M. 1996. Inhibition by L-ascorbic acid and other antioxidants of 2,2’-azino-bis (3-ethylbiazoline-6-sulfonic acid) oxidation catalyzed by peroxidase: a new approach for determining total antioxidant status of food. Anal Biochem. 236(2):255–261. doi: 10.1006/abio.1996.0164.
- Association of Official Analytical Chemists (AOAC). 2000. Official methods of analysis. 17th ed. Washington (DC): Association of Official Analytical Chemist.
- Aviagen. 2014. Ross Broiler management handbook. http://en.aviagen.com/assets/Tech_Center/Ross_Broiler/Ross-Broiler-Handbook-2014i-EN.pdf.
- Ayala A, Muñoz MF, Argüelles S. 2014. Lipid peroxidation: production, metabolism, and signaling mechanisms of malondialdehyde and 4-hydroxy-2-nonenal. Oxid Med Cell Longev. 2014:360438. doi: 10.1155/2014/360438.
- Bar-Or D, R, Bar O, LT, Rael, EN, Brody. 2015. Oxidative stress in severe acute illness. Redox Biol. 4:340–345. doi: 10.1016/j.redox.2015.01.006.
- Barrow PA. 1992. Probiotics for chickens. In: Fuller R, editor. Probiotics. London: Chapman and Hall; p. 225–257.
- Blois MS. 1958. Antioxidant determinations by the use of a stable free radical. Nature. 181(4617):1199–1200. doi: 10.1038/1811199a0.
- Borges A, Ferreira C, Saavedra MJ, Simoes M. 2013. Antibacterial activity and mode of action of ferulic and gallic acids against pathogenic bacteria. Microb Drug Resist. 19(4):256–265. doi: 10.1089/mdr.2012.0244.
- Brown DI, Griendling KK. 2015. Regulation of signal transduction by reactive oxygen species in the cardiovascular system. Circ Res. 116(3):531–549. doi: 10.1161/CIRCRESAHA.116.303584.
- Campos FM, Couto JA, Figueiredo AR, Tóth IV, Rangel AOSS, Hogg TA. 2009. Cell membrane damage induced by phenolic acids on wine lactic acid bacteria. Int J Food Microbiol. 135(2):144–151. doi: 10.1016/j.ijfoodmicro.2009.07.031.
- Caspary WF. 1992. Physiology and pathophysiology of intestinal absorption. Am J Clin Nutr. 55(Suppl 1):299S–308S. doi: 10.1093/ajcn/55.1.299s
- Chaiittianan R, Sripanidkulchai B. 2014. Development of a nanoemulsion of Phyllanthus emblica L. branch extract. Drug Dev Ind Pharm. 40(12):1597–1606. doi: 10.3109/03639045.2013.838580.
- Chen HH, Chen YT, Huang YW, Tsai HJ, Kuo CC. 2012. 4-Ketopinoresinol, a novel naturally occurring ARE activator, induces the Nrf2/HO-1 axis and protects against oxidative stress-induced cell injury via activation of PI3K/AKT signaling. Free Radic Biol Med. 52(6):1054–1066. doi: 10.1016/j.freeradbiomed.2011.12.012.
- Chen LW, Chuang WY, Hsieh YC, Lin HH, Lin WC, Lin LJ, Chang SC, Lee TT. 2021. Effects of dietary supplementation with Taiwanese tea byproducts and probiotics on growth performance, lipid metabolism, and the immune response in red feather native chickens. Anim Biosci. 34(3):393–404. doi: 10.5713/ajas.20.0223.
- Choi A, Alam J. 1996. Heme oxygenase-1: function, regulation, and implication of a novel stress-inducible protein in oxidant-induced lung injury. Am J Respir Cell Mol Biol. 15(1):9–19. doi: 10.1165/ajrcmb.15.1.8679227.
- Chuang WY, Lin LJ, Shih HD, Shy YM, Chang SC, Lee TT. 2021. The potential utilization of high-fiber agricultural by-products as monogastric animal feed additives: a review. Animals. 11(7):2098. doi: 10.3390/ani11072098.
- Chuang WY, Liu CL, Tsai CF, Lin WC, Chang SC, Shih HD, Shy YM, Lee TT. 2020. Evaluation of waste mushroom compost as a feed supplement and its effects on the on fat metabolism and antioxidant capacity of broilers. Animals. 10(3):445. doi: 10.3390/ani10030445.
- Chueh CC, Lin LJ, Lin WC, Huang SH, Jan MS, Chang SC, Chung WS, Lee TT. 2019. Antioxidant capacity of banana peel and its modulation of Nrf2-ARE associated gene expression in broiler chickens. Ital J Anim Sci. 18(1):1394–1403. doi: 10.1080/1828051X.2019.1667884.
- Chung YM, Kim JS, Yoo YD. 2006. A novel protein, Romo1, induces ROS production in the mitochondria. Biochem Biophys Res Commun. 347(3):649–655. doi: 10.1016/j.bbrc.2006.06.140.
- Cook RH, Bird FH. 1973. Duodenal villus area and epithelial cellular migration in conventional and germ-free chicks. Poult Sci. 52(6):2276–2280. doi: 10.3382/ps.0522276.
- Copple IM, Goldring CE, Kitteringham NR, Park BK. 2008. The Nrf2-Keap1 defence pathway: role in protection against drug-induced toxicity. Toxicology. 246(1):24–33. doi: 10.1016/j.tox.2007.10.029.
- Costa LB, Luciano FB, Miyada VS, Gois FD. 2013. Herbal extracts and organic acids as natural feed additives in the pig diet. S Afr J Anim Sci. 43:181–193.
- Dinis TC, Maderia VM, Almeida LM. 1994. Action of phenolic derivates (acetoaminophen, salycilate, and 5- aminosalycilate) as inhibitors of membrane lipid peroxidation and as peroxyl radical scavengers. Arch Biochem Biophys. 315(1):161–169. doi: 10.1006/abbi.1994.1485.
- Djeridane A, Yousfi M, Nadjemi B, Boutassouna D, Stocker P, Vidal N. 2006. Antioxidant activity of some Algerian medicinal plants extractscontaining phenolic compounds. Food Chem. 97(4):654–660. doi: 10.1016/j.foodchem.2005.04.028.
- Droge W. 2002. Free radicals in the physiological control of cell function. Physiol. Rev. 82(1):47–95. doi: 10.1152/physrev.00018.2001.
- Droleskey RE, Oyofo BA, Hargis BM, Corrier DE, Deloach IR. 1994. Effect of mannose on Salmonella typhimurium-mediated loss of mucosal epithelial integrity in cultured chick intestinal segments. Avian Dis. 38(2):275–281. doi: 10.2307/1591949.
- Duan X, Li J, Li W, Xing X, Zhang Y, Li W, Zhao L, Sun G, Gao X-H, Li B. 2016. Antioxidant tert-butylhydroquinone ameliorates arsenic-induced intracellular damages and apoptosis through induction of Nrf2-dependent antioxidant responses as well as stabilization of anti-apoptotic factor Bcl-2 in human keratinocytes. Free Radic Biol Med. 94:74–87. doi: 10.1016/j.freeradbiomed.2016.02.009.
- Duh PD, Tu YY, Yen GC. 1999. Antioxidant activity of water extract of Harng Jyur (Chrysanthemum morifolium Ramat). Lebensm Wiss Technol. 32(5):269–277. doi: 10.1006/fstl.1999.0548.
- Espinosa-Diez C, Miguel V, Mennerich D, Kietzmann T, Sánchez-Pérez P, Cadenas S, Lamas S. 2015. Antioxidant responses and cellular adjustments to oxidative stress. Redox Biol. 6:183–197. doi: 10.1016/j.redox.2015.07.008.
- Esterbauer H, Schaur RJ, Zollner H. 1991. Chemistry and biochemistry of 4-hydroxynonenal, malonaldehyde and related aldehydes. Free Radic Biol Med. 11(1):81–128. doi: 10.1016/0891-5849(91)90192-6.
- Estévez M. 2015. Oxidative damage to poultry: from farm to fork. Poult Sci. 94(6):1368–1378. doi: 10.3382/ps/pev094.
- Fellenberg MA, Speisky H. 2006. Antioxidants: their effects on broiler oxidative stress and its meat oxidative stability. World Poult Sci J. 62(1):53–70. doi: 10.1079/WPS200584.
- Ganie SA, Haq E, Hamid A, Qurishi Y, Mahmood Z, Zargar BA, Masood A, Zargar MA. 2011. Carbon tetrachloride induced kidney and lung tissue damages and antioxidant activities of the aqueous rhizome extract of Podophyllum hexandrum. BMC Complement Altern. Med. 11:17.
- Guo FC, Williams BA, Kwakkel RP, Li HS, Li XP, Luo JY, Li WK, Verstegen MW. 2004. Effects of mushroom and herb polysaccharides, as alternatives for an antibiotic, on the cecal microbial ecosystem in broiler chickens. Poult Sci. 83(2):175–182. doi: 10.1093/ps/83.2.175.
- Hilton PJ, Palmer-Jones R. 1973. Relationship between the flavanol composition of fresh tea shoots and the theaflavin content of manufactured tea. J Sci Food Agric. 24(7):813–818. doi: 10.1002/jsfa.2740240709.
- Huang CM, Lee TT. 2018. Immunomodulatory effects of phytogenics in chickens and pigs. Asian-Australas J Anim Sci. 31(5):617–627. doi: 10.5713/ajas.17.0657.
- Iamsaard S, Arun S, Burawat J, Sukhorum W, Wattanathorn J, Nualkaew S, Sripanidkulchai B. 2014. Phenolic contents and antioxidant capacities of Thai-Makham Pom (Phyllanthus emblica L.) aqueous extracts. J Zhejiang Univ Sci B. 15(4):405–408. doi: 10.1631/jzus.B1300284.
- Jaeschke H. 1995. Mechanisms of oxidant stress-induced acute tissue injury. Proc Soc Exp Biol Med. 209(2):104–111. doi: 10.3181/00379727-209-43885b.
- Jamroz D, Wiliczkiewicz A, Wertelecki T, Orda J, Skorupińska J. 2005. Use of active substances of plant origin in chicken diets based on maize and locally grown cereals. Br Poult Sci. 46(4):485–493. doi: 10.1080/00071660500191056.
- Jansman AJM. 1993. Tannins in feedstuffs for simple-stomached animals. Nutr Res Rev. 6(1):209–236. doi: 10.1079/NRR19930013.
- Ji LL. 2007. Antioxidant signaling in skeletal muscle: a brief review. Exp Gerontol. 42(7):582–593. doi: 10.1016/j.exger.2007.03.002.
- Kachadourian R, Pugazhenthi S, Velmurugan K, Backos DS, Franklin CC, McCord JM, Day BJ. 2011. 2′,5′-Dihydroxychalcone-induced glutathione is mediated by oxidative stress and kinase signaling pathways. Free Radic Biol Med. 51(6):1146–1154. doi: 10.1016/j.freeradbiomed.2011.05.041.
- Kaur I, Chouhan PK, Jaryal M, Saxena S, Kanishak S. 2012. Antioxidant and antimicrobial activity of leaf extract of Adhatoda vasica against the bacteria isolated from the sputum samples of asthmatic patients. Int J Drug Res Technol. 2:273–278.
- Khan KH. 2009. Roles of Emblica officinalis in medicine – A review. Bot Res Int. 2:218–228.
- Kujala TS, Loponen JM, Klika KD, Pihlaja K. 2000. Phenolics and betacyanins in red beetroot (Beta vulgaris) root: distribution and effect of cold storage on the content of total phenolics and three individual compounds. J Agric Food Chem. 48(11):5338–5342. doi: 10.1021/jf000523q.
- Kumar M, Wadhwa D, Sharma VK, Sharma A. 2012. Effect of Amla (Emblica officinalis) pomace feeding on growth performance of commercial broilers. Indian J. Anim. Nut. 29:388–392.
- Lee TT, Chou CH, Wang CL, Lu HY, Yang WY. 2022. Bacillus amyloliquefaciens and Saccharomyces cerevisiae feed supplements improve growth performance and gut mucosal architecture with modulations on cecal microbiota in red-feathered native chickens. Anim Biosci. 35(6):869–883. doi: 10.5713/ab.21.0318.
- Lee MT, Lin WC, Lee TT. 2019. Potential crosstalk of oxidative stress and immune response in poultry through phytochemicals—A review. Asian-Aust. J. Anim. Sci. 32(3):309–319.
- Lee MT, Lin WC, Lin LJ, Wang SY, Chang SC, Lee TT. 2020a. Effects of dietary Antrodia cinnamomea fermented product supplementation on antioxidation, anti-inflammation, and lipid metabolism in broiler chickens. Asian-Aust. Asian-Australas J Anim Sci. 33(7):1113–1125. doi: 10.5713/ajas.19.0392.
- Lee MT, Lin WC, Lin LJ, Wang SY, Chang SC, Lee TT. 2020b. Effects of dietary Antrodia cinnamomea fermented product supplementation on metabolism pathways of antioxidant, inflammatory, and lipid metabolism pathways-a potential crosstalk. Asian-Australas J Anim Sci. 33(7):1167–1179. doi: 10.5713/ajas.19.0393.
- Lee MT, Lin WC, Yu B, Lee TT. 2017. Antioxidant capacity of phytochemicals and their potential effects on oxidative status in animals. Asian–Aust. Asian-Australas J Anim Sci. 30(3):299–308. doi: 10.5713/ajas.16.0438.
- Lin WC, Lee TT. 2020. Laetiporus sulphureus-fermented wheat bran enhanced the broiler growth performance by improving the intestinal microflora and inflammation status. Poult Sci. 99(7):3606–3616. doi: 10.1016/j.psj.2020.04.011.
- Lin WC, Lee TT. 2021. The Laetiporus sulphureus fermented product enhanced the antioxidant status, intestinal tight junction, and morphology of broiler chickens. Animals. 11(1):149. doi: 10.3390/ani11010149.
- Lin WC, Lee MT, Chang YL, Shih CH, Chang SC, Yu B, Lee TT. 2017. Effects of mulberry leaves on production performance and the potential modulation of antioxidative status in laying hens. Poult Sci. 96(5):1191–1203. doi: 10.3382/ps/pew350.
- Lin CC, Lin LJ, Wang SD, Chiang CJ, Chao YP, Lin J, Kao ST. 2014. The effect of serine protease inhibitors on airway inflammation in a chronic allergen-induced asthma mouse model. Mediators Inflamm. 2014:879326. doi: 10.1155/2014/879326.
- Liu B, Chen Y, St Clair DK. 2008. ROS and p53: a versatile partnership. Free Radic Biol Med. 44(8):1529–1535. doi: 10.1016/j.freeradbiomed.2008.01.011.
- Liu J, Mori A. 1993. Age-associated changes in superoxide dismutase activity, thiobarbituric acid reactivity and reduced glutathione level in the brain and liver in senescence accelerated mice (SAM): a comparison with ddY mice. Mech Ageing Dev. 71(1–2):23–30. doi: 10.1016/0047-6374(93)90032-m.
- Liu CL, Shih YR, Tang PC, Lin LJ, Lee TT. 2022. Effects of dietary supplementation with Bacillus spp. and Debaryomyces spp. on broiler’s growth performance, serum characteristics, intestinal microflora, and antioxidant activity. Ital. J Anim Sci. 21(1):717–728. doi: 10.1080/1828051X.2022.2059022.
- Machlin LJ, Bendich A. 1987. Free radical tissue damage: protective role of antioxidant nutrients. FASEB J. 1. FASEB J. 1(6):441–445. doi: 10.1096/fasebj.1.6.3315807.
- Manach C, Scalbert A, Morand C, Rémésy C, Jiménez L. 2004. Polyphenols: food sources and bioavailability. Am J Clin Nutr. 79(5):727–747. doi: 10.1093/ajcn/79.5.727.
- Mandal AB, Kulkarni R, Rokade JJ, Bhanja SK, Singh R. 2017. Effect of Dietary Addition of Amla (Emblica officinalis) on Performance and HSP70 Gene Expression in Coloured Broiler Chicken during Extreme Summer. Jour Anim Rese. 7(2):233–241. doi: 10.5958/2277-940X.2017.00035.3.
- Manual ROSS. 2009. ROSS broiler management manual. Scotland: Aviagen Limited.
- McCord JM. 1979. Superoxide, superoxide dismutase and oxygen toxicity. Rev Biochem Toxicol. 1:109–124.
- McCord JM, Edeas MA. 2005. SOD, oxidative stress and human pathologies: a brief history and a future vision. Biomed Pharmacother. 59(4):139–142. doi: 10.1016/j.biopha.2005.03.005.
- McMahon M, Thomas N, Itoh K, Yamamoto M, Hayes JD. 2006. Dimerization of substrate adaptors can facilitate cullinmediated ubiquitylation of proteins by a “tethering” mechanism: a two-site interaction model for the Nrf2-Keap1 complex. J Biol Chem. 281(34):24756–24768. doi: 10.1074/jbc.M601119200.
- Muralikrishna AR, Hatcher JF. 2006. Phospholipase A2, reactive oxygen species, and lipid peroxidation in cerebral ischemia. Free Radic Biol Med. 40(3):376–387. doi: 10.1016/j.freeradbiomed.2005.08.044.
- Murugesan GR, Syed B, Haldar S, Pender C. 2015. Phytogenic feed additives as an alternative to antibiotic growth promoters in broiler chickens. Front Vet Sci. 2:21. doi: 10.3389/fvets.2015.00021.
- Na AR, Chung YM, Lee SB, Park SH, Lee MS, Yoo YD. 2008. A critical role for Romo1-derived ROS in cell proliferation. Biochem Biophys Res Commun. 369(2):672–678. doi: 10.1016/j.bbrc.2008.02.098.
- Naidu S, Vijayan V, Santoso S, Kietzmann T, Immenschuh S. 2009. Inhibition and genetic deficiency of p38 mAPK up-regulates heme oxygenase-1 gene expression via Nrf2. J Immunol. 182(11):7048–7057. doi: 10.4049/jimmunol.0900006.
- Nain P, Saini V, Sharma S. 2012. In-vitro antibacterial and antioxidant activity of Emblica officinalis leaves extract. Int J Pharm Pharm Sci. 4:385–389.
- Niforou K, Cheimonidou C, Trougakos IP. 2014. Molecular chaperones and proteostasis regulation during redox imbalance. Redox Biol. 2:323–332. doi: 10.1016/j.redox.2014.01.017.
- NRC. 1994. Nutrient requirements of poultry. 9th rev. ed. Washington (DC): National Academy Press.
- Oyaizu M. 1986. Studies on Products of Browning Reactions: antioxidative Activities of Product of Browning Reaction Prepared from Glucosamine. Jpn J Nutr Diet. 44(6):307–315. doi: 10.5264/eiyogakuzashi.44.307.
- Patel AP, Bhagwat SR, Pawar MM, Prajapati KB, Chauhan HD, Makwana RB. 2016. Evaluation of Emblica officinalis fruit powder as a growth promoter in commercial broiler chickens. Vet World. 9(2):207–210. doi: 10.14202/vetworld.2016.207-210.
- Patil RG, Kulkarni AN, Bhutkar SS, Korake RL. 2012. Effect of different feeding levels of Emblica officinalis (Amla) on performance of broilers. Res J Anim Husbandry Dairy Sci. 3:102–104.
- Poltanov EA, Shikov AN, Dorman HJ, Pozharitskaya ON, Makarov VG, Tikhonov VP, Hiltunen R. 2009. Chemical and antioxidant evaluation of Indian gooseberry (Emblica officinalis Gaertn., syn. Phyllanthus emblica L) supplements. Phytother Res. 23(9):1309–1315. doi: 10.1002/ptr.2775.
- Pourmorad F, Hosseinimehr SJ, Shahabimajd N. 2006. Antioxidant activity, phenol and flavonoid contents of some selected Iranian medicinal plants. Afr J Biotechnol. 5:1142–1145.
- Qin S, Hou DX. 2017. The Biofunctions of Phytochemicals and Their Applications in Farm Animals: the Nrf2/Keap1 System as a Target. Engineering. 3(5):738–752. doi: 10.1016/J.ENG.2017.03.011.
- Rahimi S, Teymouri Zadeh Z, Karimi Torshizi MA, Omidbaigi R, Rokni H. 2011. Effect of the three herbal extracts on growth performance, immune system, blood factors and intestinal selected bacterial population in broiler chickens. J Agric Sci Technol. 13:527–539.
- Rhee KS, Anderson LM, Sams AR. 1996. Lipid peroxidation potential of beef, chicken and pork. J Food Sci. 61(1):8–12. doi: 10.1111/j.1365-2621.1996.tb14714.x.
- SAS Institute Inc. 2004. SAS user’s guide version 9.1. Cary (NC): SAS Institute.
- Saura-Calixto F, Serrano J, Goni I. 2007. Intake and bioaccessibility of total polyphenols in a whole diet. Food Chem. 101(2):492–501. doi: 10.1016/j.foodchem.2006.02.006.
- Saura-Calixto F. 2011. Dietary fiber as a carrier of dietary antioxidants: an essential physiological function. J Agric Food Chem. 59(1):43–49. doi: 10.1021/jf1036596.
- Singh JP, Kaur A, Singh N, Nim L, Shevkani K, Kaur H, Arora DS. 2016. In vitro antioxidant and antimicrobial properties of jambolan (Syzygium cumini) fruit polyphenols. LWT Food Sci Technol. 65:1025–1030. doi: 10.1016/j.lwt.2015.09.038.
- Spencer NY, Engelhardt JF. 2014. The basic biology of redoxosomes in cytokine mediated signal transduction and implications for disease-specific therapies. Biochemistry. 53(10):1551–1564. doi: 10.1021/bi401719r.
- Sripanidkulchai B, Fangkrathok N. 2014. Antioxidant, antimutagenic and antibacterial activities of extracts from Phyllanthus emblica branches. Songklanakarin J Sci Technol. 36:669–674.
- Stoilova I, Krastanov A, Stoyanova A, Denev P, Gargova S. 2007. Antioxidant activity of a ginger extract (Zingiber officinale). Food Chem. 102(3):764–770. doi: 10.1016/j.foodchem.2006.06.023.
- Su BW, Lin WC, Lin LJ, Huang CM, Chuang WY, Wu DJ, Shih CH, Lee TT. 2020. Laying diet supplementation with Ricinus communis L. leaves to laying diet and evaluating of productive performance and potential modulation of antioxidative status. J Poult Sci. 57(4):259–269. doi: 10.2141/jpsa.0190077.
- Sun Y, Hung WC, Chen FY, Lee CC, Huang HW. 2009. Interaction of tea catechin (—)-epigallocatechin gallate with lipid bilayers. Biophys J. 96(3):1026–1035. doi: 10.1016/j.bpj.2008.11.007.
- Sun Z, Zhang S, Chan JY, Zhang DD. 2007. Keap1controls postinduction repression of the Nrf2-mediated Antioxidant response by escorting nuclear export of Nrf2. Mol Cell Biol. 27(18):6334–6349. doi: 10.1128/MCB.00630-07.
- Tachakittirungrod S, Okonogi S, Chowwanapoonpohn S. 2007. Study on antioxidant activity of certain plants in Thailand: mechanism of antioxidant action of guava leaf extract. Food Chem. 103(2):381–388. doi: 10.1016/j.foodchem.2006.07.034.
- Tahir I, Khan MR, Shah NA, Aftab M. 2016. Evaluation of phytochemicals, antioxidant activity and amelioration of pulmonary fibrosis with Phyllanthus emblica leaves. BMC Complement Altern Med. 16:406.
- Tsai CF, Lin LJ, WAng CH, Tsai CS, Chang SC, Lee TT. 2021. Assessment of intestinal immunity and permeability of broilers on partial replacement diets of two-stage fermented soybean meal by Bacillus velezensis and Lactobacillus brevis ATCC 367. Animals. 11(8):2336. doi: 10.3390/ani11082336.
- Tsai CF, Lin LJ, Wang CH, Tsai CS, Chang SC, Lee TT. 2022. Effects of fermented soybean meal by Bacillus velezensis, Lactobacillus spp. or their combination on broiler performance, gut antioxidant activity and microflora. Anim Biosci. 35(12):1892–1903. doi: 10.5713/ab.21.0529.
- Valko M, Rhodes CJ, Moncol J, Izakovic M, Mazur M. 2006. Free radicals, metals and antioxidants in oxidative stress-induced cancer. Chem Biol Interact. 160(1):1–40. doi: 10.1016/j.cbi.2005.12.009.
- Variya BC, Bakrania AK, Patel SS. 2016. Emblica officinalis (Amla): a review for its phytochemistry, ethnomedicinal uses and medicinal potentials with respect to molecular mechanisms. Pharmacol Res. 111:180–200. doi: 10.1016/j.phrs.2016.06.013.
- Viveros A, Chamorro S, Pizarro M, Arija I, Centeno C, Brenes A. 2011. Effects of dietary polyphenol-rich grape products on intestinal microflora and gut morphology in broiler chicks. Poult Sci. 90(3):566–578. doi: 10.3382/ps.2010-00889.
- Wan XL, Niu Y, Zheng XC, Huang Q, Su WP, Zhang JF, Zhang LL, Wang T. 2016. Antioxidant capacities of Artemisia annua L. leaves and enzymatically treated Artemisia annua L. in vitro and in broilers. Anim Feed Sci Technol. 221:27–34. doi: 10.1016/j.anifeedsci.2016.08.017.
- Weiss SJ. 1986. Oxygen, ischemia and inflammation. Acta Physiol Scand Suppl. 548:9–37.
- Windisch W, Schedle K, Plitzner C, Kroismayr A. 2008. Use of phytogenic products as feed additives for swine and poultry. J Anim Sci. 86:140–148.
- Xu C, Yagiz Y, Hsu WY, Simonne A, Lu J, Marshall MR. 2014. Antioxidant, antibacterial, and antibiofilm properties of polyphenols from Muscadine grape (Vitis rotundifolia Michx.) pomace against selected foodborne pathogens. J Agric Food Chem. 62(28):6640–6649. doi: 10.1021/jf501073q.
- Yang B, Liu P. 2014. Composition and biological activities of hydrolyzable tannins of fruits of Phyllanthus emblica. J Agric Food Chem. 62(3):529–541. doi: 10.1021/jf404703k.
- Yason CV, Summers BA, Schat KA. 1987. Pathogenesis of rotavirus infection in various age groups of chickens and turkeys: pathology. Am J Vet Res. 48(6):927–938.
- Yu X, Chu S, Hagerman AE, Lorigan GA. 2011. Probing the interaction of polyphenols with lipid bilayers by solid-state NMR spectroscopy. J Agric Food Chem. 59(12):6783–6789. doi: 10.1021/jf200200h.
- Wang S, Towler MJ, Weathers PJ. 2016. Root regulation of artemisinin production in Artemisia annua: trichome and metabolite evidence. Planta. 244(5):999–1010. doi: 10.1007/s00425-016-2560-0.
- Zhang YJ, Abe T, Tanaka T, Yang CR, Kouno I. 2001. Phyllanemblinins A–F, new ellagitannins from Phyllanthus emblica. J Nat Prod. 64(12):1527–1532. doi: 10.1021/np010370g.
- Zhang YJ, Nagao T, Tanaka T, Yang CR, Okabe H, Kouno I. 2004. Antiproliferative activity of the main constituents from Phyllanthus emblica. Biol Pharm Bull. 27(2):251–255. doi: 10.1248/bpb.27.251.
- Zhang GF, Yang ZB, Wang Y, Yang WR, Jiang SZ, Gai GS. 2009. Effect of ginger root (Zingiber officinale) processed to different particle sizes on growth performance, antioxidant status, and serum metabolites of broiler chickens. Poult Sci. 88(10):2159–2166. doi: 10.3382/ps.2009-00165.
- Zheng W, Wang SY. 2001. Effect of plant growth temperature on antioxidant capacity in strawberry. J Agric Food Chem. 49(10):4977–4982. doi: 10.1021/jf0106244.
- Zijlstra RT, Whang KY, Easter RA, Odle J. 1996. Effect of feeding a milk replacer to early-weaned pigs on growth, body composition, and small intestinal morphology, compared with suckled littermates. J Anim Sci. 74(12):2948–2959. doi: 10.2527/1996.74122948x.