ABSTRACT
Marburg virus (MARV) has been a major concern since 1967, with two major outbreaks occurring in 1998 and 2004. Infection from MARV results in severe hemorrhagic fever, causing organ dysfunction and death. Exposure to fruit bats in caves and mines, and human-to-human transmission had major roles in the amplification of MARV outbreaks in African countries. The high fatality rate of up to 90% demands the broad study of MARV diseases (MVD) that correspond with MARV infection. Since large outbreaks are rare for MARV, clinical investigations are often inadequate for providing the substantial data necessary to determine the treatment of MARV disease. Therefore, an overall review may contribute to minimizing the limitations associated with future medical research and improve the clinical management of MVD. In this review, we sought to analyze and amalgamate significant information regarding MARV disease epidemics, pathophysiology, and management approaches to provide a better understanding of this deadly virus and the associated infection.
Introduction
Marburg virus (MARV) causes deadly outbreaks with a high fatality rate. It is responsible for several outbreaks since its concurrent discovery and characterization in 1967 in Marburg, Germany; Frankfurt, Germany; and Belgrade, Yugoslavia (now Serbia). The majority of the MARV outbreaks occurred in Africa. MARV is into the NIAID Category A Priority Pathogen list, and is the primary cause of MARV disease (MVD) [Citation1]. MVD is deadly and often becomes untreatable in humans and non-human primates (NHPs), resulting in hemorrhagic fever and organ dysfunctions, such as liver failure, the infection of the spleen, brain, and renal tissues, and coagulation problems throughout the body [Citation2,Citation3].
This virus belongs to the order Mononegavirales, the family Filoviridae, and the genus Marburgvirus. This genus only includes one species, named Marburg marburgvirus, generally known as Marburg virus [Citation4]. Various studies have shown that MARV has five different lineages based on the phylogenetic analysis of genomic sequence data obtained from samples collected during different outbreaks [Citation4,Citation5]. These lineages have been reclassified into two separate viruses: the Ravn virus (RAVV) and MARV [Citation4]. The human-to-human transmission characteristics of MARV are similar to those of the better characterized Ebolaviruses, including Ebola virus (EBOV) [Citation6], Sudan virus, and Bundibugyo virus [Citation7]. Due to the sporadic nature of MARV, distinguishing the natural reservoirs of this virus has been difficult. However, vigorous attempts and ongoing research have successfully determined the natural sources of this virus, which defines the viral transmission mechanism. The studies have substantiated that apart from Rousettus aegyptiacus bat species as the major natural source for MARV, Hipposideros caffer and some other Chiroptera can also serve as natural sources of infection [Citation8,Citation9]. Currently available clinical data have suggested that MVD has three stages associated with distinct symptoms [Citation10]. Laboratory findings have indicated that the primary target of the virus is mononuclear phagocytic cells, followed by the epithelial cells in various organs [Citation11]. However, diverse human exposure to this virus and the unorganized nature of currently available information have served as impediments to both researchers and policy-makers attempting to design appropriate guidelines for combating this disease. Though some drugs or vaccines have been successfully developed for MVD, its malignancy is still a great concern [Citation12].
This review elaborately describes the history of MVD outbreaks, summarizes available information regarding the viral structure and genome, and describes the known sources of MARV and the transmission methods of both natural source-to-human and human-to-human infection pathways. This review also describes the pathophysiology, cellular tropism, immune evasion, and sites of major damage within the host body to provide a better understanding of the pathogenesis of MVD. Currently available clinical findings and management approaches are also described to help researchers to make appropriate decisions in preparation for future MVD outbreaks. No methods have been approved for the control of MVD outbreaks, and numerous studies remain necessary to develop drugs or vaccines. Our review will help future researchers comprehend the background of this virus and provide a profound understanding of the mechanism of infection, which will facilitate the MARV disease management and designing of future drugs and vaccines.
Methodology
An organized literature search strategy was followed to find all the published articles, which reported outbreak history, genome sequence, structure, sources, pathophysiology, damaging prospects, cellular tropism, immune evasion, clinical findings, symptoms, transmission, and management of Marburg virus. To retrieve the information, we thoroughly searched for relevant literature through Google Scholar, Scopus, and PubMed between 1967 and October 2021. We have developed some specific Boolean words based on our objective, as shown in . These words were developed by using outcome term, descriptive term, population term, and area term. Boolean words “AND”, as well as “OR” along with [All fields] and [MeSH terms] searching techniques were used for literature searching in Scopus and PubMed. Advanced search strategy has also been maintained in Google Scholar, and some adjustments have been made on the basis of all search engine requirements.
Table 1. Electronic database search algorithm.
Genome and structure of Marburg virus
MARV is a pleomorphic virus which is observed in six, circular, U, rod-like and most commonly in filamentous shape [Citation13]. Usually, MARV virions are of 80 nm in diameter and although their length varies greatly, the average length of a MARV virion is 790 nm [Citation14]. The surface of the virion is shielded with 5 to 10 nm-long spikes placed at interludes of approximately 10 nm [Citation13,Citation15].
It is a non-segmented negative-sense virus which contains a 19.1 kb long RNA genome that encodes seven genes in the following linear order-3'-NP-VP35-VP40-GP-VP30-VP24-L-5' [Citation16]. Each of these seven genes has a highly conserved transcription start and stop signal and also possess unconventionally long noncoding nucleotide sequence at the 3' and 5' extremities [Citation16]. These noncoding regions containing cis-acting element play role in replication, transcription and also in DNA packaging [Citation16,Citation17]. All but two genes of MARV are segregated by 4–97 nucleotides long intergenic regions, the transcription stop signal of VP24 and the transcription start signal of VP30 gene share a five nucleotide long overlapping sequence UAAUU [Citation18] ().
Figure 1. Virion structure and genome organization of Marburg virus. Top, the Marburg virus structure along with depicting the structural proteins. Bottom, an illustration of the genome organization of the Marburg virus. This seven-gene strain of Marburg virus has been drawn roughly to scale. The light blue boxes indicate noncoding areas, as well as the colored box code regions for genes. The red arrows demonstrate the position of the transcriptional start signals, whilst the pale brown bars highlight conserved transcriptional stop signals. The genes are segregated by intergenic regions, indicated using black arrows, with the exception of the overlapping sequence (black triangle) between VP24 and VP30. At the extreme ends, the 3' and 5' trailer sequence is shown.
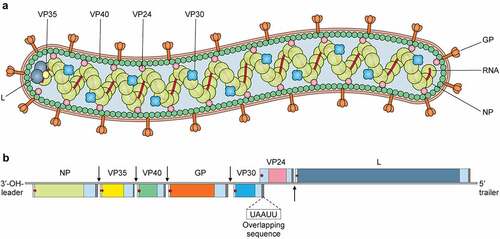
All of the seven genes in the MARV genome are monocistronic [Citation16] and are responsible for encoding the following seven structural proteins- Nucleoprotein (NP), Viral protein 35 (VP35), Viral protein (VP40), Glycoprotein (GP), Viral protein 30 (VP30), Viral protein 24 (VP24) and Large Protein (L). The genome of MARV is encapsulated with a nucleocapsid complex made up of four structural proteins- NP, VP35, VP30 and L [Citation19]. NP is the major nucleocapsid protein which forms a tubular helical structure which interacts with VP35 and the resulting complex interacts with L [Citation19,Citation20]. Here the L protein functions as the RNA dependent RNA polymerase and VP35 works as the polymerase cofactor [Citation21]. All four nucleocapsid complex proteins play essential role in viral genome replication and transcription. MARV contains a host derived membrane layer, which is spiked in regular intervals. These spikes, made up of heavily glycosylated protein (GP), are necessary for attachment to susceptible host cells [Citation15]. The inner matrix of the virion is formed by VP40 and is responsible for budding and association with matrix and nucleocapsid [Citation22,Citation23]. VP24 shows interaction with both NP and other cellular membranes and is associated with the release of progeny virions from the cell [Citation24]. illustrates the functions and characteristics of the proteins in MARV.
Table 2. Marburg virus genes, proteins and their characteristics and functions.
Historical outbreaks and disease epidemiology
The first outbreak of MARV was documented in Marburg, Germany, in 1967, where scientists and laboratory technicians were doing experiments with tissue derived from African green monkeys (Chlorocebus aethiops), collected from Uganda in an attempt to produce vaccines for polio [Citation43,Citation44]. Concurrently, additional outbreaks erupted in Yugoslavia and Frankfurt. Electron microscopy was performed to identify and characterize the virus on plasma from infected guinea pigs, and it was named the “Marburg virus” [Citation44].
The next documented MARV outbreak occurred in South Africa in 1975, which infected three people. The first individual became infected while traveling to Zimbabwe, and his companion and a nurse became infected through human-to-human transmission [Citation45,Citation46]. In 1980, a third outbreak of MARV was identified in Kenya, where a male became infected following a visit to Kitum cave, and a doctor became infected while treating this individual [Citation47]. Another small outbreak occurred in Kenya in 1987, which resulted in the detection of a new strain of MARV. However, the only infected individual was a 15-years-old Danish boy who became infected 7 days after a visit to Kitum cave [Citation48].
In 1988, 1990, 1991, and 1995, laboratory accidents resulted in occurrences of MARV infection in Russia (). The subsequent MARV outbreak took place between 1998 and 2000, in the DRC (Democratic Republic of the Congo), which was associated with 154 total infections. Initially, young gold miners became infected during their mining work in the village of Durba, and the outbreak later spread to the nearby village of Watsa. At least nine genetically diverse MARV lineages were identified during this outbreak [Citation8,Citation49]. Another severe MARV outbreak took place in Angola’s Uige region, which was first identified in October 2004, and continued through July 2005. The outbreak was initially identified as due to the death of a hospital employee in Uige, and continued to spread through other provinces. The largest numbers of infections and deaths associated with a single outbreak to date occurred during this outbreak, which resulted in 252 infection cases and 227 deaths, representing a fatality rate of 90%. Clinical investigations revealed similarities between the genetic sequences of previously identified MARV isolates and those associated with the 2004–2005 Angolan MARV outbreak [Citation50].
Table 3. Marburg virus outbreaks epidemiology and case fatality rate (CFR) in accordance with the outbreak strain.
To date, no other severe outbreaks have occurred following the 2004–2005 Angolan outbreak, apart from certain sporadic cases in various zones of the world. Four miners were infected in the gold and lead mines of Uganda’s Kamansanga district during mining operations in 2007 [Citation51]. This outbreak occurrence of Uganda related to the two following MARV cases, the first one was found in the USA and the next one was found in the Netherlands (). However, the US patient survived this MARV infection, but the Dutch patient died afterward (). Interestingly, these two individuals became MARV infected while traveling Uganda’s Maramagambo forest python cave [Citation52,Citation53]. Moreover, Uganda was the site of the next three epidemics (). The first one occurred in the Kabale region of Uganda in 2012, lasting for three weeks, during which fifteen individuals became MARV infected, four of whom died later on. The genomic sequence of 2012 Uganda’s outbreak strain was similar to the formerly identified strain’s genomic sequences [Citation54]. The following MARV outbreak also took place in Uganda in 2014, in the city of Kampala, when a healthcare worker became infected and passed away after a few days. This MARV strain also had similarities in genome sequences with the formerly determined MARV strain’s genome sequences from Egyptian fruit bats [Citation55]. Another recent epidemic occurrence of MARV took place in Uganda’s Kween region in 2017. In this outbreak, 4 people in the same family were infected with MARV and only one person survived later. This strain’s genome sequences also had similarities with the previously identified strain [Citation56]; however, the clinical findings associated with this outbreak remain inadequate, and intense research is currently ongoing.
Figure 2. Outbreak history of Marburg virus. The red color on the map demonstrates the occurrences of outbreaks associated with new strains of the Marburg virus. Primarily, MARV outbreaks have been identified in four countries in Africa: The Democratic Republic of Congo, Angola, Kenya, and Uganda. However, an outbreak in Zimbabwe was also associated with a novel strain of the Marburg virus, which caused an outbreak in South Africa. The yellow color on the map shows infections in which the source is known to have originated from another country. This type of outbreak occurred in Germany, the Netherlands, the USA, and South Africa. The green color shows the outbreaks associated with unintentional laboratory exposures. This sort of epidemic took place only in Russia.
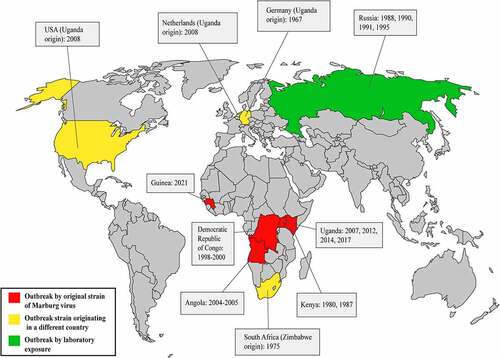
Lastly, the most recent outbreak has been declared in Guinea in August 2021 and ended in September 2021, where one male got infected and died; but there are no data available regarding the strain [Citation57,Citation58].
The disease epidemiology of MARV, along with its case fatality rate is shown in , which were collected from all outbreak data associated with MARV infection.
Source and transmission of Marburg virus
Historically, numerous MARV strains have been isolated from both animals and humans. Animals, specifically bats are the natural reservoirs of MARV [Citation9]. MARV strains were isolated from reservoir hosts in different courses of time to comprehend their genomic variations and disease pathogenesis. Moreover, the presence of MARV in reservoir hosts have been substantiated through some laboratory tests, most specifically through PCR-positive tests. The number of isolated strains responsible for disease spread within the reservoir host species at different year and country is shown in (Supplementary Table S1). Rousettus aegyptiacus species of bat most frequently acts as a reservoir of MARV, along with Hipposideros caffer and some unclassified Chiroptera as the minor sources. It is because the majority of the MARV strains were collected from Rousettus aegyptiacus species, which included 3 from Gabon in 2005 [Citation68]; 1 from Kenya [Citation69] and 10 from Uganda in 2007 [Citation8]; 5 from Uganda in 2008 [Citation8,Citation70]; 1 from Gabon [Citation71] and 16 from Uganda in 2009 [Citation70]; 30 from Uganda in 2012 [Citation72]; 1 from South Africa in 2013; 5 from Sierra Leone [Citation73] and 1 from South Africa in 2017 [Citation74]; and 6 from Sierra Leone [Citation73], and 2 from Zambia in 2018 [Citation75]. Furthermore, 12 MARV strains were collected from unclassified Chiroptera in DRC in 1999 [Citation9], and 1 MARV strain was collected from Hipposideros caffer in Uganda in 2007 [Citation8].
Figure 3. Number of MARV strains identified from reservoir bat species, which were responsible for the disease spread in different years and countries.
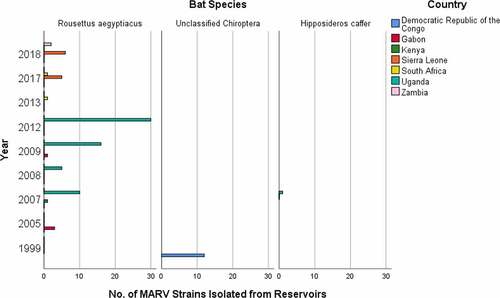
The bat-to-bat transmission of MARV strains may occur in several ways. A recent study that detected the shedding of MARV in rectal, oral, and urine samples from MARV-inoculated bats, also found that MARV is present in oral and blood samples of in-contact bats. This study proved the horizontal transmission of MARV from infected bats to the in-contact bats () [Citation76]. The previous study found the presence of MARV in the lung, intestine, kidneys, bladder, salivary gland, and females’ reproductive tract tissues of inoculated bats, which helped to presume that MARV transmission may occur vertically or horizontally within the reservoirs [Citation77]. It is also hypothesized that bat-to-bat transmission may occur through biting [Citation7], sexual interactions [Citation7,Citation70], or by hematophagous arthropods [Citation76].
Figure 4. Transmission and spread of Marburg virus. Reservoirs of the Marburg virus, such as African fruit bats, can spread the virus among themselves by direct contamination, through sexual transmission, or due to biting. Direct contact with reservoir hosts or viral-contaminated fruit consumption may spread the virus to humans and non-human primates (NHPs). Transmission between humans and NHPs may occur through direct contact, and NHP-to-human transmission occurs due to bushmeat consumption and through direct contact. Direct contact and aerosols can facilitate both human-to-human and NHP-to-NHP transmission.
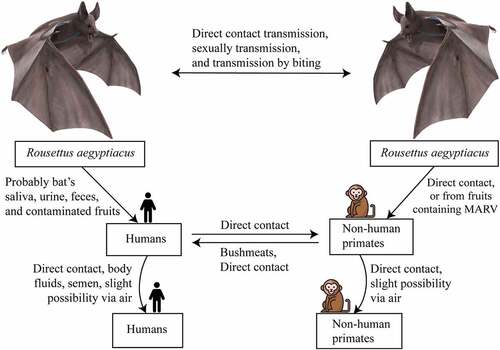
Intermediate hosts, such as non-human primates (NHPs) and animals hunted for bushmeat, in addition to natural reservoirs, for example saliva, urine, and excrement of bat, are the primary vectors of MARV transmission. The transmitting paths from reservoir hosts to humans remain unknown [Citation78,Citation79]. However, it is possible that bat’s saliva, urine, and feces, as well as MARV-contaminated fruits, are the probable causes of transmission in humans as well as NHPs () [Citation76,Citation80]. provides all of the known primary and secondary sources of MARV transmission, along with their infection pathways.
Table 4. Sources and infection types of Marburg virus.
In the early stages, MARV may spread to humans by infected intermediate animals. Besides, MARV may transmit due to sexual intercourse within humans, since the presence of virus antigens in the semen of infected males has already been substantiated [Citation78]. The studies on EBOV also suggested the persistence of EBOV RNA as well as infectious EBOV in semen after the patients’ recoveries from Ebola virus disease, which shares a similar human-to-human transmission dynamic as MVD [Citation87–89]. Furthermore, the virus can transmit from one human to another due to direct contact with blood, as well as other body fluids, for example feces, saliva, urine, teardrops, mucous, and breast milk (). Managing and providing healthcare service to the MARV infected patient, and inappropriate handling of human corpses also leads to increased chances of MARV transmission [Citation90]. Transmission has also been hypothesized to occur through the air during an outbreak, as the virus may survive aerosols [Citation91], hence mucosal membranes are highly susceptible to viral transmission through the air (). Apart from aerosol survival, an important study suggested that Lake Victoria MARV can also survive on liquids for longer periods, and on solids surfaces (plastics and glasses) for more than 3 weeks at low temperature [Citation92]. Therefore, fomite transmission of MARV can play an important role in virus spreading, especially during an outbreak. Other forms of MARV transmission include the breakdown of skin cells, and the parenteral or enteral introduction of drugs and foods [Citation93].
Clinical findings and symptoms
Most available clinical data were obtained from a few large outbreaks, especially the 1967 outbreak of Germany and Belgrade of Yugoslavia, the 1998 to 2000 outbreak of DRC, and the 2004 to 2005 outbreak of Angola [Citation44,Citation60,Citation94]. The clinical characteristics of a MARV-infected patient may vary depending upon various factors, including strain virulence, physical status, host susceptibility, and medical maintenance. To date, the reported incubation period has ranged between 2–21 days, and the average duration is 5 to 9 days, in humans [Citation60,Citation95].
MHF can be divided into 3 distinct phases; initial generalization phase, that is followed by early organ phase, and then the late organ phase or convalescence phase, according to disease progression [Citation96] ().
Table 5. MVD symptoms, according to the phase of infection.
Phase 1 (generalization phase)
The initial generalization phase lasts for five days after disease onset. The early symptoms that are present during this phase include generic flu-like characteristics, accompanied by high fever (39–40 °C). In addition, debilitating symptoms, including fatigue, loss of appetite, abdominal pain, severe weight loss, severe nausea, vomiting, watery diarrhea, and anorexia, have been reported by many patients. Severe headaches, myalgia, chills, and malaise are also common signs [Citation46,Citation83,Citation93]. The end of this initial phase is frequently characterized by conjunctivitis, dysphasia, enanthem, and pharyngitis. A characteristic maculopapular rash may develop on different body parts (prominently on the neck, back, and stomach), which represents a distinctive characteristic of filovirus infection. Other symptoms include lymphadenopathy, leukopenia, and thrombocytopenia [Citation97–101].
Phase 2 (early organ phase)
A sustained high fever and other general symptoms accompany the early organ phase, which lasts from five to thirteen days after the onset of symptoms. Patients may manifest conjunctival infection, prostration, shortness of breath (dyspnea), viral exanthema, irregular vascular permeability, and edema [Citation93]. Neurological symptoms, such as confusion, encephalitis, irritability, delirium, and aggression, have also been reported in patients [Citation46,Citation83,Citation102]. Approximately 75% of patients are present with hemorrhagic manifestations, including mucosal bleeding, melena, petechiae, bloody diarrhea, visceral hemorrhagic effusions, uncontrolled leakage from venipuncture sites, hematemesis, and ecchymoses. Bleeding from the nose, gums, and vagina has also been reported. Because hemorrhagic symptoms are present in some patients, the MARV infection is typically denoted MHF in these cases, although this is still not favorable to all cases. Multiple organs, including the kidney, liver, and pancreas, are affected during this phase of infection. Elevated serum activity was also noticed in most infected individuals [Citation98–101].
Phase 3 (late organ phase/convalescent phase)
The late stage of MARV infection results in two distinct outcomes: infection either becomes fatal or patients enter a prolonged phase of restoration. Fatality generally occurs between eight and sixteen days after the onset of symptoms. Typically, shock and multi-organ failure are the primary drivers of death [Citation83,Citation102]. The late organ phase (in non-fatal cases) starts on day thirteen and lasts until day twenty and beyond during the course of the disease. Severe metabolic disturbances, including convulsions and severe dehydration, result in severe negative effects on overall patient health, resulting in multi-organ dysfunction and anuria. Orchitis has been reported in some cases during this phase. Neurological symptoms persist during this stage. An additional complication includes spontaneous abortion in pregnant women [Citation66,Citation102]. Myalgia, exhaustion, partial amnesia, sweating, peeling skin in rash-affected areas, and secondary infections are noticeable signs during this prolonged phase. Arthralgia, hepatitis, asthenia, ocular disease, and psychosis are common complications during the convalescent phase of the infection [Citation98–101].
Viral dissemination and cellular tropism of Marburg virus
Viral entry and budding
The dissemination and replication of MARV in hosts facilitates the penetration and navigation of viral particles into several cells [Citation103]. MARV gains entry to the host through mucosal surfaces, breakage and scratches of the skin, or by inoculation, and the virus gains access to tissues remote from their infection site by damaging subcellular mechanisms. The entry of MARV into the host cell includes three different phases: i) cellular attachment, ii) endocytosis, and iii) fusion. Several potential cell entry mechanisms have been proposed, which include clathrin-mediated endocytosis, macropinocytosis, and glycoprotein-facilitated receptor binding. However, these mechanisms are not mutually exclusive and apply to different parts of the entry pathway [Citation104]. Various attachment factors, such as tyrosine kinase receptors and C-like lectins; as well as cellular receptors, such as NPC1 have been identified as possible mediators of viral entry [Citation105]. In case of budding, the intracellular localizing of recombinant VP40 and its release in the VLP (virus-like particle) form are greatly affected by over-expression or inhibition of myosin-10 and Cdc42 proteins, which are also crucial in filopodium formation and function. Moreover, MARV VP40 can interact with the viral nucleocapsid, and provide an interface of MARV subviral particles as well as filopodia. Filopodia are closely contacted with the adjacent cells, thus usurping these structures can facilitate spreading of MARV to the neighboring cells [Citation106]. This is why high viral titers in the blood are seen in both animals and humans infected with MARV. When viruses are released from basolateral membranes, they provide access to the underlying tissue and vasculature, leading to severe infections. Furthermore, the basolateral portion of hepatocytes as well as biliary epithelial cells were found to be responsible for MARV budding. This activity is associated with VP40 protein, which is vital for releasing infectious particles within the infected host to promote disease progression () [Citation107,Citation108].
Figure 5. MARV entry, viral dissemination, and cellular tropism. The yellow color in the figure shows viral entry mechanisms, whereas the red color shows viral dissemination pathways. MARV enters the host and spreads throughout the lymphatic and vascular systems. The light brown color indicates the damaged cellular organelles. MARV causes necrosis in many organs, including the liver, spleen, kidneys, gastrointestinal tract, and endocardium.
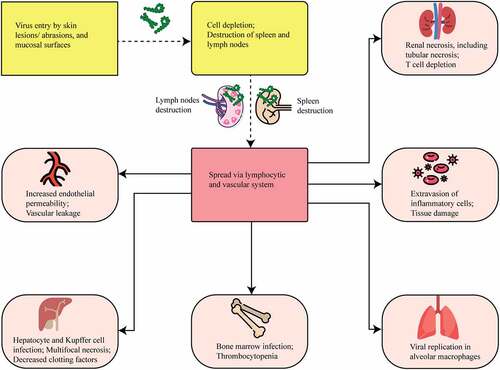
Cellular damage and viral tropism
MARVs are among the most devastating and virulent pathogens that affect humans. Autopsies of MARV-infected patients have revealed swelling of the heart, brain, spleen, kidneys, and lymph nodes. Also in NHPs, hemorrhages were identified in mucous membranes and soft tissues; the most severe necrotic lesions were observed in the lymph nodes, liver, spleen, testes, ovaries, gastrointestinal tract, and endocardium [Citation67]. These organs contain a high number of reticuloendothelial cells that can migrate and spread to several organs, causing abnormal vascular permeability and the activation of clotting cascade. During the later stage of the disease, hemorrhages are seen in the gastrointestinal tract, in the pericardial, pleural, and peritoneal cavities, and in the renal tubule, associated with the fibrin deposition [Citation59].
Liver
The severity of MARV’s hepatic damages is apparently greater than EBOV [Citation59]. In MARV infections, the liver features distinctive histopathology characteristics. Studies on fatal humans and NHPs have shown that the liver represents a critical replicating organ for MARV [Citation109]. Hepatocyte necrosis ranges from focal to widespread, with slight inflammation, which causes swelling, the degradation of liver cells and the reticuloendothelial system, mild to moderate steatosis, and upper cell hyperplasia. The elevation of liver enzymes, such as alanine amino-transferase, aspartate amino-transferase, serum glutamic pyruvic transaminase, and serum glutamic oxaloacetic transaminase, are hallmarks of MARV infection [Citation86]. Hepatocytes may show basophilic inclusions of the cytoplasm near necrotic eosinophilic regions, consisting of viral nucleocapsid aggregates [Citation110,Citation111]. The asialoglycoprotein receptor has been recognized as a liver specific receptor, likely to mediate MARV infections [Citation112]. Because the synthesis of several clotting factors occur in liver, pathologically changes in liver can potentiate abnormalities in coagulation, including DIC (disseminated intravascular coagulation), which are associated with MARV infection and increase the risk of multi-organ failure. Furthermore, hypotension and hypovolemia are consequences of adrenal gland infections, associated with the retardation of steroid-synthesizing enzymes, causing shock [Citation113].
Spleen and lymph nodes
MARV infection in humans causes damage to lymphatic tissues, for example necrosis of the follicles and the medulla of lymph nodes, and red pulp of the spleen, in addition to lymphocyte depletion. Interestingly, lymphocytes are not infected by the virus, although bystander apoptosis occurrence results in lymphocyte depletion [Citation114,Citation115]. In NHPs, both the red and white pulp of the spleen display moderate necrosis, and lymphoid depletion in the white pulp was apparent, whereas the red pulp accumulated fibrin and cellular debris. The sinuses were covered with a small quantity of cell debris and granular material [Citation67]. In humans, viral antigen was found in the marginal region of the red pulp and macrophages but were not detected in germinal centers, despite severe necrosis. Marburg-like inclusions were often observed in macrophages but were never detected in lymphatic cells. Fibrin and fibrinocellular debris were deposited throughout the red pulp. Sometimes virions have been observed in combination with the deposit [Citation110,Citation116,Citation117].
Lungs
The lung alveoli often showed diffuse congestion, hemorrhage, suppurative pneumonia, and bacterial co-infection [Citation110,Citation116]. Small necrotic foci/micronecrosis, and fibrin were identified in alveolar macrophages, and the endothelia of alveolar capillaries were often disturbed in MARV-infected patients [Citation110].
Gastrointestinal tract and small intestine
A large volume of plasma cells and monocytes exist in lymphatic organs and the mucous membranes of the stomach and intestines of NHPs [Citation67]. In humans, the submucosa showed severe edema, including the invasion of degenerated inflammatory cells (such as neutrophils) and multiple hemorrhage foci. Autolysis of the intestine impeded cellular identification. The gastrointestinal tract showed mild focal mononuclear penetration in the lamina propria of the gastric, small intestinal and colonic mucosa. Macrophages exhibited Marburg-like inclusions, and virions were present in reticular fibrils and debris from necrotic cells. These results explain the human-to-human transmissions that can occur due to exposure to bloody stools [Citation110].
Kidneys
The kidneys were swollen, pale, and hemorrhagic; tubular necrosis and parenchymal damage cause tubular dysfunction, which leads to proteinuria in MARV patients [Citation109]. Multiple suppurative embolic foci associated with gram-negative bacteria were observed. Viral antigen was observed multifocally in glomerulus, proximal tubular epithelial cells, as well as in interstitial connective tissues near capillaries. Marburg virus-like inclusions occur in intertubular tissues macrophage and fibroblast-like cells, and some virions were observed in the glomerular capillaries, but no viral antigen was observed in the medulla [Citation110]. Proximal tubular cells (PTCs) and mitoribosomes (mitochondrial ribosomes) were infected by MARV and induce significant changes in gene expression, leading to acute kidney injury in MARV infection via disruption of the PTC’s energy supply [Citation118].
Skin
Skin and mucous membranes typically show hemorrhagic abnormalities in MHF, which is associated with skin lesions. Limited histopathological changes occur to skin tissues, including endothelial cell swelling, focal hemorrhage, necrosis, and dermal edema. Cutaneous effects appear regularly between the 2nd and 7th days after symptoms onset, and can occur during the recovery period as well [Citation119]. Epidermal DCs, endothelial cells, connective tissue fibroblasts, and even the epithelium of sebaceous glands and sweat contain viral antigens [Citation59].
Testis
A study showed that following the onset of symptoms, MARV can persist in the semen for up to 7 weeks [Citation120]. The inclusion of viral antigens in seminiferous tubules supports the potential for sexual transmission of MARV [Citation121]. Scrotal pain is often identified, with a few orchitis cases, and necrosis has been described in the testicles and ovaries of MHF patients [Citation86]. Persistent testicular MARV infections among NHP survivors can lead to severe testicular injuries, including sperm cell loss and inflammatory invasion. MARV persists primarily in Sertoli cells, resulting in the breakdown of the blood–testis barrier [Citation84]. MARV infection also induces focal orchitis, germ cell destruction, and the abundant deposition of IgG antibodies [Citation122].
Bone marrow
Morphological changes to the bone marrow in MHF remain imprecisely defined. MARV antigen infects normocellular bone marrow, causing focal necrosis. However, thrombocytopenia can be observed without a concomitant reduction in platelet production in MARV cases, similar to EBOV cases [Citation122].
Heart and central nervous system
Morphological myocardial injuries observed in MARV autopsy cases have not been uniform. Multiple suppurative embolic foci and lesions containing gram-negative bacteria (such as Pseudomonas) have been observed in the myocardium, but no viral antigen has been associated with these lesions [Citation110]. A few MARV cases have demonstrated panencephalitis, with glial nodules and mild perivascular lymphocytic infiltration in the brain [Citation86].
Endothelial cell dysfunction
Endothelial cells are one of the target cells for MARV replication because replication in endothelial cells maintains and strengthens the viremic phase. This hypothesis is supported by the observance of viral budding from the apical plasma membrane. However, basolateral budding would enable viruses to spread into the tissues even early during the infection process. Endothelial cells are responsible for the maintenance of barriers between blood and the surrounding tissue; therefore, viral replication may cause the loss of barrier function, allowing viral spread into the tissues. Endothelial cell activation and the associated secretion of inflammatory mediators can also increase vascular permeability. DIC occurs due to the increased consumption of coagulation factors, resulting in shock syndrome and hemorrhage [Citation123,Citation124].
Immune evasion of MARV
The mechanisms that mediate MARV pathogenesis remain poorly established at the molecular level in vivo [Citation125]. MARV VP40 plays a role in the virion as a matrix protein and has recently been shown to be involved in host innate immune antagonism through various mechanisms [Citation126,Citation127]. In early-stage MARV infection, no differences in B cell expression were observed in the NHP model, and changes in B cell levels did not appear until late in the infection [Citation128]. MARV infection causes changes to the host gene expression profile within 24–48 hours after infection, and most of these changes affect genes associated with immunoregulations, coagulations, and apoptotic pathways. Moreover, the gene expression change is linked with interferon-stimulated gene (ISG) production in hepatocytes, which can act as severe antiviral suppression mediators. These findings suggest that MARV can successfully abolish interferon (IFN) reactions, including type 1 and type 2 IFN signaling, provoke an inflammatory cytokine response, and demonstrate rapid replication kinetics [Citation129,Citation130].
Hematological modifications have also been observed, such as early leukopenia, neutrophilia, and monocytosis, with moderate eosinophilia [Citation86]. These changes cause immuno-suppression in MVD patients, leading to the secondary infection by bacteria [Citation85]. Moreover, elevated levels of soluble nitric oxide and proinflammatory cytokine in the blood induce intravascular apoptosis [Citation2]. The activity of EBOV VP35 has been shown to obstruct the development of IFN by inhibiting IFN-regulatory factor 3 (IRF3) activation [Citation131,Citation132]; but this response has not yet explicitly established for MARV. Moreover, EBOV VP24 inhibits type 1 IFN signaling by restricting the nuclear importing of phosphorylated STAT1 [Citation133]; however, MARV VP24 did not demonstrate this phenomenon. Rather, MARV VP40 did inhibit the JAK-STAT pathway by restricting the phosphorylation of both STAT1 and STAT2 [Citation127]. However, no available data exists to describe the acquired immune responses following MHF recovery. Both IgM and IgG antibodies were observed during the DRC outbreak after symptom onset among MARV survivors, indicating the development of MARV-specific antibodies [Citation134].
Pathophysiology of Marburg virus
MARV usually penetrates the body through cracked skin, causing damage to multiple cell types and organs, resulting in Marburg hemorrhagic fever (MHF) [Citation135]. The most severe clinical features of MVD include inappropriate fluid distribution, coagulation complications, shock, and multi-organ failures. MARV mainly infects macrophages, monocytes, Kupffer cells, and DCs, according to evidence from MARV-infected monkeys [Citation115]. MARV targets mononuclear phagocytic cells, for instance monocytes and macrophages, triggering the cellular activation and permitting damage to secondary targets, such as endothelial cells [Citation10,Citation11,Citation67]. Moreover, the activation of macrophages and monocytes releases cytokines and pro-inflammatory mediators, resulting in the progression of shock, which is a primary cause of death in MVD [Citation10,Citation136].
MARV pathophysiology in humans
Limited comprehensive clinical studies exist for MARV due to the rural conditions as well as severe occurrences of most of the MARV outbreaks in Africa, and the compilation of laboratory and pathological evidence from patients has traditionally been inadequate. Clinical findings to date emerge from information linked to the first epidemic in Germany, with the South African outbreak, and smaller outbreaks in other areas of Africa [Citation137]. Experiments on cultured cells of the MARV patient indicated substantial adaptive reactions against MARV by increasing immune cells, in beginning. In addition, immunoglobulin G (IgG) reaction to the viral NP and GP was found while investigating the serum sample of patients, and 2 patients had notable neutralizing titer of the antibodies. Gradually, neutralizing antibody titer declined, and this declination started 21 months post-infection, and diminished lower than detecting limit 27 months post-infection [Citation138].
At organ level, in patients with MARV infection, it seems that MARV mainly targets the adrenal glands and the liver (), as well as lymphoid tissues for replication [Citation139]. Autopsies performed on RAVV infected patients, a close-relative of MARV, indicated multiple malignant effects, including the swelling of the kidneys, heart, brain, and lymphoid tissues, in addition to hemorrhages of the soft tissues and mucosa [Citation67]. The hepato-tropism of MARV was discovered in an in vitro analysis, suggesting that the hepatocyte receptor asialoglycoprotein may worsen MARV infections [Citation112]. The necrosis of parenchymal cells in the liver destroys the reticuloendothelial system, resulting in increased liver enzyme levels in association with MARV infection [Citation96,Citation140]. The infection of other organs can result in various symptoms, including proteinuria due to malfunction of kidney [Citation134], heart and lungs hemorrhages [Citation1], scrotum pain, and necrosis of the testicles and ovaries [Citation114].
Figure 6. MARV hemorrhagic fever pathophysiology model in humans. Marburg virus primarily targets dendritic cells, monocytes, parenchyma cells at a liver, adrenocortical cells, and several lymphoid tissues. Infection of dendritic cells leads to poor stimulating condition of T lymphocyte that causes lymphocyte apoptotic condition. Due of this, body’s immunity is suppressed and cytokines/chemokines number is increased, which leads to shock as well as multiorgan damaging occurrence. Macrophage or monocyte infection leads to uncontrolled cytokines/chemokines activation, and they continue the damaging of T lymphocyte and endothelial cell. Endothelial cell infection causes increase of blood vessels permeability and DIC (disseminated intra-vascular coagulopathy), while both occurrences lead to hemorrhages. Systemic replication can also occur because of this infection in endothelial cells. Parenchymal cell infection occurrences in liver can decrease coagulation factors, and these occurrences can cause hemorrhages later on. Adrenocortical cells of adrenal gland infecting occurrence by MARV can lead to disorders in the metabolism and dysregulated blood pressure; and hemorrhage occurs at a later stage due to these infections. MARV infection the on lymphoid tissues of lymphatic system, especially lymph nodes and spleen infections lead to tissue necrosis and malfunctioning adaptive immunity. Shock and lymphoid organ damage can occur in the later stage.
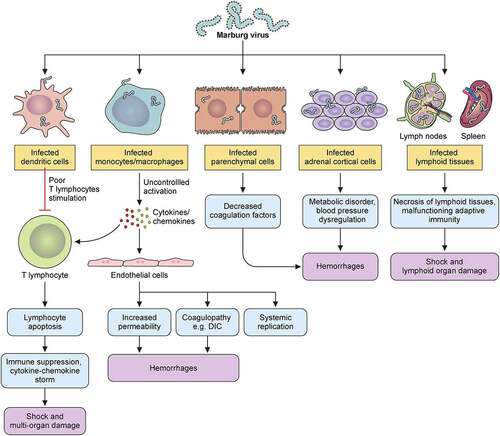
At the cellular level, macrophage, and dendritic cell (DC) are the primary targets for MARV entrance [Citation110,Citation141]. These findings were supported by the identification of virions and antigens associated with the virus using immunohistochemical and electron microscopy experiments during the 1987 Kenyan outbreak [Citation110], as well as by the observation of viral infections in the macrophages of macaques based on the results of peripheral smear of blood mono-nuclear cell populations from flow cytometry study [Citation128]. The infection of DCs causes the paralysis of inherent immune response, and the abnormalities of lymphocytes stimulation [Citation125]. The macrophage infections promote the production of proinflammatory cytokines including TNF-α (tumor necrosis factor-alpha), which can cause bystander apoptosis in lymphocyte populations, leading to immunosuppression and lymphopenia (). By stimulating monocytes, infected macrophages help in the dissemination of MARV, which induces the development of cytokines and chemokines [Citation142,Citation143]. Although the activation is not associated with MARV replication, this process contributes significantly to viral spread and delineates the pantropic nature of MARV and annihilation of focal tissues [Citation10]. Furthermore, modifications in vascular permeability are induced by interleukin (IL)-6 and TNF-α, which are both formed by macrophages [Citation144]. Furthermore, tissue factors production by infected macrophage leads to the abnormal coagulations, such as DIC, and this is additionally supported by liver hepatocyte cells infection and causing decreased coagulating factor synthesis from liver () [Citation145]. Infections on adrenocortical cells cause hypotension as well as metabolism disturbance, and these can cause multiorgan failure and shock, together with immunosuppression and coagulopathy () [Citation146].
MARV pathophysiology in animals
MARV pathophysiology has been observed in animal models, including guinea pigs, mice, hamsters, baboon [Citation147,Citation148], and certain species of NHPs, such as rhesus macaques, common marmosets, cynomolgus macaques, squirrel monkeys, and African green monkeys [Citation149,Citation150]. MARV has been shown to infect Kupffer and monocytes cells, as well as DCs and macrophages, in animal models. The reduction of NK (natural killer) cells, and CD4 and CD8 T lymphocyte cells generally occur throughout the viral infection time because of the death of these cells through apoptosis [Citation67,Citation151].
In the MARV-infected humanized mouse model, Marburg virus manifests malignancy in a greater range. MARV primarily infects macrophages, B cells, T cells, NK cells, and phagocytes in these mice [Citation152]. In non-adapted wild type MARV-Ravn infected mice models, virus infection causes acute illness, faded and enlarged livers, featuring necrosis, enlarged spleens, and reduced lymphocytic cell populations [Citation153], while mouse-adapted MARV-Ravn models are accompanied by several symptoms, such as reduced physical activity, weight loss, rumpled fur 3 to 4 days post-infection, and death within 5 to 7 days [Citation154].
Guinea pig shows mild feverish manifestation when infected by host-adapted variant of MARV. However, consecutive passaging the guinea pigs with MARV can cause malignant conditions among the animals. In fact, one of the first MARV guinea pig model studies showed that 100% of the MARV-inoculated guinea pigs die 7–9 days post-infection after 8 passages by whole blood [Citation155]. This feature was further used to generate and characterize a guinea pig model against MARV Angola strain. In the experiment of MARV Angola strain on guinea pigs, it was substantiated that MARV infections can cause lymphocytopenia, thrombocytopenia, as well as greater infecting occurrences in kidney cells, liver cells, spleens, and lungs [Citation156]. The viral pathophysiology of MARV in guinea pigs appears to resemble those observed in mice and humans. Furthermore, vaccination studies have been widely performed in guinea pigs, and one study testing the efficiency of trivalent rVSV vaccine vectors demonstrated that 100% protection is possible when MARV infection occurs in them [Citation157].
Syrian hamster models usually show no manifestations when infected with wild-type MARV strains. But STAT2 null immunodeficient hamster models show infections of liver and spleen, neutrophil leukocytosis, and an increase of proinflammatory cytokine [Citation158]. Besides, another study confirmed that hamster-adapted variant of MARV in Syrian golden hamster models manifest similar pathophysiological response to MARV infection as those observed in human and NHP, including similar clinical symptoms, such as rashes, hemorrhages, coagulopathies, and malfunctioned immune response [Citation159].
Among all tested NHP models, rhesus and cynomolgus macaques are the best-characterized as well as widely used in MARV research due to their accurate recapitulation of human pathophysiology [Citation160]. MARV infection on NHPs showed that lymphocytosis is prevalent in the initial stages [Citation161], and leukocytosis and thrombocytopenia is seen within 5 to 6 days after infection because of the increased neutrophil levels. MARV then infects adrenal glands, hepatocytes, as well as lymphoid tissues. During the final stages, the virus infects the endothelia of several organ tissues. The viral antigens can be found in several organs, including the kidneys, liver, spleen and adrenal glands. Typically, diarrhea, anorexia, fever, rash, and hemorrhage may manifest after 2 to 6 days of infection, and viremia arises on the 3rd day. The highest titer is typically observed after 8 days of infection [Citation115].
In ferrets, an experiment recently demonstrated that MARV neither causes disease nor causes viremia in them [Citation162]. Although the serology reports for MARV infection were positive and these ferrets produced neutralizing antibodies, they did not develop clinical disease [Citation163]. Additional studies on ferrets may contribute to our comprehension of the various mechanisms that contribute to differences in pathophysiology, hence improving our understanding of the disease.
Management approaches for Marburg virus
No established management approach for MARV currently exists. Nevertheless, a popular treatment approach is the utilization of palliatives for pain alleviating purpose. Moreover, supportive treatment is often offered, including blood volume administration and electrolyte balance [Citation164]. Although there are currently no clinically approved vaccines or therapeutics to prevent or treat MVD, there are certainly established and effective approaches that can be used to manage outbreaks and cases.
Several supportive methods and therapies were used pre-clinically and clinically in the past, as well as several new approaches that have been studied in animal models [Citation2,Citation12,Citation165–167]. In supportive therapies, antibiotic was recommended in almost each of the outbreaks; yet numerous improved supportive therapeutic approaches were established in every outbreak. At the time of the first MARV occurrence in 1967, a variety of supportive approaches rather than only antibiotics were used, including cardiac glycosides, antipyretics, steroids, electrolytes, convalescent serum, and fluids supplement [Citation85,Citation168]. Clinicians introduced the use of parenteral fluids, analgesics, prophylactic heparins, and plasma of Lassa fever patients to the infected humans during the second outbreak [Citation46,Citation169]. Anti-malarial drugs and antibiotics were used in the 1980 outbreak of Kenya [Citation47]. Moreover, in the 1987 Kenya outbreak, heparins, steroids, antibiotics, and plasma were utilized, and concurrently dialysis was proceeded [Citation48]. In the 1990 Russian outbreak, extracorporeal hemosorbents were used, in addition to hemodialysis [Citation63]. In addition to antibiotics and anti-malarials, the next epidemic in the DRC in 1998–2000 had the implementation of modern acetaminophens, antiemetics, antacids, and intravenous fluids [Citation49]. During the 2004–2005 Angolan epidemic, no treatment was provided to patients initially, but antimalarials, antibiotics, analgesics, antiemetics, sedatives, and cimetidine were provided later, as well as oral rehydration was administered concurrently during the first 3 months and intra-venous fluid were given after 3 months [Citation170–172]. Although the information was unavailable regarding the MARV management of Uganda outbreak initially in 2007 but, some supportive managements were provided during the 2008 Uganda-origin outbreak of MARV, including blood transfusion, malaria prophylaxis, antiemetics, cholecystectomies, and antibiotic for the case that transferred to the USA [Citation52]. Moreover, intravenous fluid, plasma, hemofiltration, and hypertonic saline were provided to the case that transferred to the Netherlands [Citation166]. Newly, an innovative approach of remote controlled and pressure guided fluid supplementation has been proposed for the efficacious management of filovirus infected patients [Citation173].
Modern researches have investigated various drugs for MARV infection. A recent study of remdesivir against Marburg virus showed that it has therapeutic efficacies in cynomolgus macaque models, which makes it an important drug to be assessed further. The study showed that it had therapeutic efficacy when given at 4 or 5 days post inoculation once daily in vehicle, for 12 days with 5 mg/kg dose, or 10 mg/kg loading dose followed by 5 mg/kg dose [Citation174]. Another study showed that cholesterol conjugated fusion inhibitors are active in vitro against Marburg virus [Citation175]. Recently, 4-(aminomethyl) benzamide has also been substantiated to be a potent entry inhibitor of MARV infection [Citation176]. Furthermore, researchers demonstrated 33 hit compounds in vitro against MARV with their several pharmacological potentials [Citation177]. Some small molecules, such as BCX4430, favipiravir, aloperine etc. have also been showed to be effective against MARV infection [Citation178]. Again, an inhibitor compound FC -10696 has recently been discovered to inhibit the egress of MARV [Citation179]. Also, AVI-7288 has been indicated to show potentials as a post-exposure prophylaxis against MARV [Citation180].
Numerous experiments were conducted on rodent and NHP models for testing vaccine efficacies against MARV. To date, some vaccines have been trialed for human use [Citation78,Citation181,Citation182]. Among them, cAd3 vaccine, also known as chimpanzee adenovirus serotype 3 vector, encoded with wild type GP from MARV, is in phase 1 clinical trial for human use [Citation183]. The MVA-BN-Filo vaccine, also known as the modified vaccinia Ankara vector vaccine, is encoded with GP from Ebola, Sudan, MARV, and NP from Tai Forest virus. It has been planned for phase 2/3 trials after the successful completion of phase 1 trial [Citation78,Citation184]. MARV DNA plasmid vaccine, which is a MARV DNA plasmid that expresses GP from MARV Angola [Citation185], as well as from MARV Sudan [Citation186]. Both of them completed phase 1 clinical trial [Citation78]. rVSV-MARV-GP vaccine is recombinant vesicular stomatitis virus vector for MARV GP, that has been studied in NHP models, but human trial is not conducted yet [Citation78,Citation187,Citation188]. In addition, VLP vaccine or virus-like particles with GP vaccines are yet to be trialed in humans, as NHP studies of VLP vaccine have been completed [Citation189,Citation190]. In recent times, single vial trivalent vaccines have been invented, which showed high antibody levels in mouse and NHP models. This vaccine might help in easier distributing and administrating procedures of vaccines in rural and poor areas [Citation191]. Hence recombinant subunit vaccines platform should be allowed to develop safer and efficient multivalent vaccine candidates for protection against MARV [Citation192].
The experimental approaches have substantiated the efficacies of numerous treatments, which include anti-viral treatment, pre- and post-exposure vaccine treatment, and treatment with IFNs; these are displayed in the . However, Experimental approaches are still being processed in animal models as well as in humans to determine the efficacy of treatments and vaccines. A very recent study substantiated that combination therapy, especially usage of monoclonal antibody (MR186-YTE) with remdesivir, can significantly protect macaques from MARV infection. The study showed that monoclonal antibodies alone can give 100% protection, and 80% protection in NHPs when used together with remdesivir 5 days post-infection [Citation193]. Therefore, further investigation of monoclonal antibodies and combination therapies, as well as their human implementation can be another possible dimension of MVD management.
Table 6. Evaluation of Marburg virus treatment in NHP models.
Conclusion and future prospects
Rural and poor conditions, along with sporadic outbreaks in Africa, are principal reasons for unsatisfactory clinical findings of the Marburg virus. Although the African fruit bat has been identified as a potential natural reservoir for MARV and most of the outbreaks have been caused by spillover into human population from an animal reservoir, human-to-human transmission mediated by the care providers or health professionals who work in hospitals also played great role in the growth of MARV outbreaks. These outbreaks demonstrated that MARV could be malignant and able to cause severe outbreaks if not taken proper steps immediately. Although MARV strains originate only in African regions, the outbreak history suggests that other continents could also be at risk. Recent outbreaks have indicated the necessity of additional research exploring the pathophysiology and pathogenicity of MARV. Current data remain based on earlier studies of infected patients. In response to the intensity of previous outbreaks, epidemiologists have suggested MARV as a threat to global public health. Therefore, novel studies examining MARV remain crucial to provide unambiguous guidelines for the therapeutic management of patients, and for the development of vaccines. These steps could be used to minimize future outbreaks and reduces the CFRs. Vaccine studies have been ongoing for the past few years, and the virus is being studied on a variety of animal models, including NHPs, mouse models, guinea pigs, and hamsters. Vaccine studies should be perpetuated until the licensure and distribution of vaccines in humans occurs.
Author contributions
Conceptualization: Mehedy Hasan Abir, Ahmed Rakib, Talha Bin Emran, Mohammad Mahmudul Hassan; Methodology: Mehedy Hasan Abir, Ahmed Rakib, Talha Bin Emran, Mohammad Mahmudul Hassan; Data curation: Mehedy Hasan Abir, Tanjilur Rahman, Ayan Das, Silvia Naznin Etu, Iqbal Hossain Nafiz, Ariful Islam; Formal Analysis: Mehedy Hasan Abir, Tanjilur Rahman, Ayan Das, Silvia Naznin Etu, Iqbal Hossain Nafiz, Ahmed Rakib; Writing – original draft: Mehedy Hasan Abir, Tanjilur Rahman, Ayan Das, Silvia Naznin Etu, Iqbal Hossain Nafiz, Saikat Mitra; Writing – review & editing: Mehedy Hasan Abir, Talha Bin Emran, Kuldeep Dhama, Ariful Islam, Abolghasem Siyadatpanah, Shafi Mahmud, Mohammad Mahmudul Hassan; Visualization: Mehedy Hasan Abir, Silvia Naznin Etu, Ariful Islam, Abolghasem Siyadatpanah, Bonlgee Kim, Mohammad Mahmudul Hassan; Supervision: Bonlgee Kim, Talha Bin Emran, Kuldeep Dhama, Mohammad Mahmudul Hassan.
Supplemental Material
Download MS Excel (77 KB)Disclosure statement
No potential conflict of interest was reported by the author(s).
Data availability statement
The authors confirm that the data supporting the findings of this study are available within the article [and/or] presented in the table and figures.
Supplementary material
Supplemental data for this article can be accessed here.
Additional information
Funding
References
- Bente D, Gren J, Strong JE, et al. Disease modeling for Ebola and Marburg viruses. Dis Models Mech. 2009;2(1–2):12–17. DOI:10.1242/dmm.000471.
- Mehedi M, Groseth A, Feldmann H, et al. Clinical aspects of Marburg hemorrhagic fever. Future Virol. 2011;6(9):1091–1106. DOI:10.2217/fvl.11.79.
- van Paassen J, Bauer MP, Arbous MS, et al. Acute liver failure, multiorgan failure, cerebral oedema, and activation of proangiogenic and antiangiogenic factors in a case of Marburg haemorrhagic fever. Lancet Infect Dis. 2012;12(8):635–642. DOI:10.1016/S1473-3099(12)70018-X.
- Kuhn JH, et al. Family - filoviridae. In: King AMQ editor. Virus taxonomy. San Diego: Elsevier; 2012. pp. 665–671.
- Carroll SA, Towner JS, Sealy TK, et al. Molecular evolution of viruses of the family Filoviridae based on 97 whole-genome sequences. J Virol. 2013;87(5):2608–2616. DOI:10.1128/JVI.03118-12.
- Boadu A, Karpoormath R, Nlooto M. Exploration of alternate therapeutic remedies in Ebola virus disease: the case of reported antiviral phytochemical derived from the leaves Spondias Mombin linn. Adv Traditional Med. 2021. DOI:10.1007/s13596-021-00603-5
- Brian RA, Jones MEB, Sealy TK, et al. OralShedding of Marburg virus in experimentally infected Egyptian fruit bats(Rousettus Aegyptiacus). J Wildl Dis. 2015;51(1):113–124. DOI:10.7589/2014-08-198.
- Towner JS, Amman BR, Sealy TK, et al. Isolation of genetically diverse Marburg viruses from Egyptian fruit bats. PLoS Pathog. 2009;5(7):e1000536. DOI:10.1371/journal.ppat.1000536.
- Swanepoel R, Smit SB, Rollin PE, et al. Studies of reservoir hosts for Marburg virus. Emerg Infect Dis J. 2007;13(12):1847. DOI:10.3201/eid1312.071115.
- Stroher U, West E, Bugany H, et al. Infection and activation of monocytes by Marburg and Ebola viruses. J Virol. 2001;75(22):11025–11033. DOI:10.1128/JVI.75.22.11025-11033.2001.
- Aronson JF, et al. Viral hemorrhagic fevers. In: Fraire AE editor. Viruses and the lung: infections and non-infectious viral-linked lung disorders. Berlin, Heidelberg: Springer Berlin Heidelberg; 2014. pp. 123–132.
- Reynolds P, Marzi A. Ebola and Marburg virus vaccines. Virus Genes. 2017;53(4):501–515.
- Bharat TAM, Riches JD, Kolesnikova L, et al. Cryo-Electron tomography of Marburg virus particles and their morphogenesis within infected cells. PLoS Biol. 2011;9(11):e1001196. DOI:10.1371/journal.pbio.1001196.
- Welsch S, Kolesnikova L, Krähling V, et al. Electron tomography reveals the steps in filovirus budding. PLoS Pathog. 2010;6(4):e1000875. DOI:10.1371/journal.ppat.1000875.
- Feldmann H, Will C, Schikore M, et al. Glycosylation and oligomerization of the spike protein of Marburg virus. Virology. 1991;182(1):353–356. DOI:10.1016/0042-6822(91)90680-A.
- Feldmann H, Mühlberger E, Randolf A, et al. Marburg virus, a filovirus: méssenger RNAs, gene order, and regulatory elements of the replication cycle. Virus Res. 1992;24(1):1–19. DOI:10.1016/0168-1702(92)90027-7.
- Sanchez A, Kiley MP, Holloway BP, et al. Sequence analysis of the Ebola virus genome: organization, genetic elements, and comparison with the genome of Marburg virus. Virus Res. 1993;29(3):215–240. DOI:10.1016/0168-1702(93)90063-S.
- Mühlberger E. Filovirus replication and transcription. Future Virol. 2007;2(2):205–215.
- Becker S, Rinne C, Hofsäß U, et al. Interactions of Marburg virus nucleocapsid proteins. Virology. 1998;249(2):406–417. DOI:10.1006/viro.1998.9328.
- Becker S, Huppertz S, Klenk H-D, et al. The nucleoprotein of Marburg virus is phosphorylated. J Gen Virol. 1994;75(4):809–818. DOI:10.1099/0022-1317-75-4-809.
- Muhlberger E, Weik M, Volchkov VE, et al. Comparison of the transcription and replication strategies of Marburg virus and Ebola virus by using artificial replication systems. J Virol. 1999;73(3):2333–2342. DOI:10.1128/JVI.73.3.2333-2342.1999.
- Swenson DL, Warfield KL, Kuehl K, et al. Generation of Marburg virus-like particles by co-expression of glycoprotein and matrix protein. FEMS Immunol Med Microbiol. 2004;40(1):27–31. DOI:10.1016/S0928-8244(03)00273-6.
- Kolesnikova L, Bamberg S, Berghofer B, et al. The matrix protein of Marburg virus is transported to the plasma membrane along cellular membranes: exploiting the retrograde late endosomal pathway. J Virol. 2004;78(5):2382–2393. DOI:10.1128/JVI.78.5.2382-2393.2004.
- Bamberg S, Kolesnikova L, Moller P, et al. VP24 of Marburg virus influences formation of infectious particles. J Virol. 2005;79(21):13421–13433. DOI:10.1128/JVI.79.21.13421-13433.2005.
- Brauburger K, Deflubé LR, Mühlberger E. Filovirus transcription and replication Pattnaik, Asit K, Whitt, Michael A. In: Biology and pathogenesis of rhabdo- and filoviruses (Hackensack, New Jersey, USA: World Scientific Publishing Co., Inc.); 2015. p. 515–555.
- DiCarlo A, Biedenkopf N, Hartlieb B, et al. Phosphorylation of Marburg virus NP region II modulates viral RNA synthesis. J Infect Dis. 2011;204(suppl_3):S927–S933. DOI:10.1093/infdis/jir319.
- Liu B, Dong S, Li G, et al. Structural insight into nucleoprotein conformation change chaperoned by VP35 peptide in Marburg virus. J Virol. 2017;91(16):e00825–17. DOI:10.1128/JVI.00825-17.
- Bale S, Julien J-P, Bornholdt ZA, et al. Marburg virus VP35 can both fully coat the backbone and cap the ends of dsRNA for interferon antagonism. PLoS Pathog. 2012;8(9):e1002916. DOI:10.1371/journal.ppat.1002916.
- Edwards MR, Liu G, Mire CE, et al. Differential regulation of interferon responses by Ebola and Marburg virus VP35 proteins. Cell Rep. 2016;14(7):1632–1640. DOI:10.1016/j.celrep.2016.01.049.
- Bruhn JF, Kirchdoerfer RN, Urata SM, et al. Crystal structure of the Marburg virus VP35 oligomerization domain. J Virol. 2017;91(2):e01085–16. DOI:10.1128/JVI.01085-16.
- Kolesnikova L, Mittler E, Schudt G, et al. Phosphorylation of Marburg virus matrix protein VP40 triggers assembly of nucleocapsids with the viral envelope at the plasma membrane. Cell Microbiol. 2012;14(2):182–197. DOI:10.1111/j.1462-5822.2011.01709.x.
- Koehler A, Pfeiffer S, Kolesnikova L, et al. Analysis of the multifunctionality of Marburg virus VP40. J Gen Virol. 2018;99(12):1614–1620. DOI:10.1099/jgv.0.001169.
- Amiar S, et al. Lipid-Specific oligomerization of the Marburg virus matrix protein VP40 is regulated by two distinct interfaces for virion assembly. J Biol Chem. 2021;296 1–20. doi:10.1016/j.jbc.2021.100796 .
- Carette JE, Raaben M, Wong AC, et al. Ebola virus entry requires the cholesterol transporter Niemann–Pick C1. Nature. 2011;477(7364):340–343. DOI:10.1038/nature10348.
- Enterlein S, Volchkov V, Weik M, et al. Rescue of recombinant Marburg virus from cDNA is dependent on nucleocapsid protein VP30. J Virol. 2006;80(2):1038–1043. DOI:10.1128/JVI.80.2.1038-1043.2006.
- Wenigenrath J, Kolesnikova L, Hoenen T, et al. Establishment and application of an infectious virus-like particle system for Marburg virus. J Gen Virol. 2010;91(5):1325–1334. DOI:10.1099/vir.0.018226-0.
- Wan W, Kolesnikova L, Clarke M, et al. Structure and assembly of the Ebola virus nucleocapsid. Nature. 2017;551(7680):394–397. DOI:10.1038/nature24490.
- Edwards MR, Johnson B, Mire C, et al. The Marburg virus VP24 protein interacts with keap1 to activate the cytoprotective antioxidant response pathway. Cell Rep. 2014;6(6):1017–1025. DOI:10.1016/j.celrep.2014.01.043.
- Zhang APP, Bornholdt ZA, Abelson DM, et al. Crystal structure of Marburg virus VP24. J Virol. 2014;88(10):5859–5863. DOI:10.1128/JVI.03565-13.
- Page A, Volchkova V, Reid S, et al. Marburgvirus Hijacks Nrf2-dependent pathway by targeting Nrf2-negative regulator Keap1. Cell Rep. 2014;6(6):1026–1036. DOI:10.1016/j.celrep.2014.02.027.
- Kirchdoerfer RN, et al. Filovirus structural biology: the molecules in the machine. In: Mühlberger E; LL Hensley and JS Towner editors. Marburg- and Ebola viruses: from ecosystems to molecules. Cham: Springer International Publishing; 2017. pp. 381–417.
- Koehler A, Kolesnikova L, Becker S. An active site mutation increases the polymerase activity of the guinea pig-lethal Marburg virus. J Gen Virol. 2016;97(10):2494–2500.
- Feldmann H, Slenczka W, Klenk H-D. Emerging and reemerging of filoviruses. Vienna: Springer Vienna; 1996.
- Ristanović ES, Kokoškov NS, Crozier I, et al. A forgotten episode of Marburg virus disease: Belgrade, Yugoslavia, 1967. Microbiol Mol Biol Rev. 2020;84(2):e00095–19. DOI:10.1128/MMBR.00095-19.
- Feldmann H, Klenk H-D. Marburg and ebola viruses. In: Maramorosch K; FA Murphy and AJ Shatkin, Feldmann H and Klenk H-D. editors. Advances in virus research. Cambridge, Massachusetts, USA: Academic Press; 1996. pp. 1–52.
- Gear JS, Cassel GA, Gear AJ, et al. Outbreak of Marburg virus disease in Johannesburg. Br Med J. 1975;4(5995):489–493. DOI:10.1136/bmj.4.5995.489.
- Smith DH, Isaacson M, Johnson KM, et al. Marburg-Virus disease in Kenya. Lancet. 1982;319(8276):816–820. DOI:10.1016/S0140-6736(82)91871-2.
- Johnson ED, et al. Characterization of a new Marburg virus isolated from a 1987 fatal case in kenya. Vienna: Springer Vienna; 1996.
- Colebunders R, Tshomba A, Van Kerkhove M, et al. Marburg hemorrhagic fever in Durba and Watsa, Democratic Republic of the Congo: clinical documentation, features of illness, and treatment. J Infect Dis. 2007;196(s2):S148–S153. DOI:10.1086/520543.
- Towner JS, Khristova ML, Sealy TK, et al. Marburgvirus genomics and association with a large hemorrhagic fever outbreak in Angola. J Virol. 2006;80(13):6497–6516. DOI:10.1128/JVI.00069-06.
- Adjemian J, Farnon EC, Tschioko F, et al. Outbreak of Marburg hemorrhagic fever among miners in Kamwenge and Ibanda Districts, Uganda, 2007. J Infect Dis. 2011;204(suppl_3):S796–S799. DOI:10.1093/infdis/jir312.
- Leggiadro RJ. Imported case of Marburg hemorrhagic Fever—Colorado, 2008: centers for disease control and prevention: MMWR.: 2009;58:. 1377–1380. Pediatr Infect Dis J. 2010;29(5):400.
- Timen A, Koopmans MPG, Vossen ACTM, et al. Response to imported case of Marburg hemorrhagic fever, the Netherlands. Emerg Infect Dis J. 2009;15(8):1171. DOI:10.3201/eid1508.090015.
- Olival KJ, Hayman DTS. Filoviruses in bats: current knowledge and future directions. Viruses. 2014;6(4):1759–1788.
- Nyakarahuka L, Ojwang J, Tumusiime A, et al. Isolated case of Marburg virus disease, Kampala, Uganda, 2014. Emerg Infect Dis J. 2017;23(6):1001. DOI:10.3201/eid2306.170047.
- Nyakarahuka L, Shoemaker TR, Balinandi S, et al. Marburg virus disease outbreak in Kween District Uganda, 2017: Epidemiological and laboratory findings. PLoS Negl Trop Dis. 2019;13(3):e0007257. DOI:10.1371/journal.pntd.0007257.
- Aborode AT, et al. Marburg virus amidst COVID-19 pandemic in guinea: fighting within the looming cases. Int J Health Plann Manage. 2021 37 553–555. doi:10.1002/hpm.3332 .
- WHO. Marburg virus disease - Guinea. 2021.
- Siegert R. Marburg virus Appel, M.J.G., Gillespie, J.H. In: Canine distemper virus. Vienna: Springer Vienna; 1972. pp. 97–153.
- Martini GA. Marburg virus disease. clinical syndrome. In: Martini GA and R Siegert, . editors. Marburg virus disease. Berlin, Heidelberg: Springer Berlin Heidelberg; 1971. pp. 1–9.
- Conrad JL, Geldenhuys P, Crees M, et al. Epidemiologic investigation of Marburg virus disease, Southern Africa, 1975. Am J Trop Med Hyg. 1978;27(6):1210–1215. DOI:10.4269/ajtmh.1978.27.1210.
- Beer B, Kurth R, Bukreyev A. Characteristics of Filoviridae: Marburg and Ebola viruses. Naturwissenschaften. 1999;86(1):8–17.
- Nikiforov V, et al. A case of a laboratory infection with Marburg fever. Zhurnal Mikrobiologii, Epidemiologii I Immunobiologii. 1994;3:104–106
- Kimman TG, Smit E, Klein MR. Evidence-Based biosafety: a review of the principles and effectiveness of microbiological containment measures. Clin Microbiol Rev. 2008;21(3):403–425.
- Ignatyev G, et al. Immunity indexes in the personnel involved in haemorrhagic virus investigation. Proceedings of the 1996 ERDEC Scientific Conference on Chemical and Biological Defense Research. Aberdeen Proving Ground (MD); 1997.
- Bausch DG, Nichol ST, Muyembe-Tamfum JJ, et al. Marburg hemorrhagic fever associated with multiple genetic lineages of virus. N Engl J Med. 2006;355(9):909–919. DOI:10.1056/NEJMoa051465.
- Geisbert TW, Hensley LE, Gibb TR, et al. Apoptosis induced in vitro and in vivo during infection by Ebola and Marburg viruses. Lab Invest. 2000;80(2):171–186. DOI:10.1038/labinvest.3780021.
- Towner JS, Pourrut X, Albariño CG, et al. Marburg virus infection detected in a common African bat. PLoS One. 2007;2(8):e764. DOI:10.1371/journal.pone.0000764.
- Kuzmin IV, Niezgoda M, Franka R, et al. Marburg virus in fruit bat, Kenya. Emerg Infect Dis J. 2010;16(2):352. DOI:10.3201/eid1602.091269.
- Amman BR, Carroll SA, Reed ZD, et al. Seasonal Pulses of Marburg virus circulation in Juvenile Rousettus aegyptiacus bats coincide with periods of increased risk of human infection. PLoS Pathog. 2012;8(10):e1002877. DOI:10.1371/journal.ppat.1002877.
- Maganga GD, Bourgarel M, Ebang Ella G, et al. Is Marburg virus Enzootic in Gabon? J Infect Dis. 2011;204(suppl_3):S800–S803. DOI:10.1093/infdis/jir358.
- Amman B, Nyakarahuka L, McElroy AK, et al. Marburgvirus resurgence in kitaka mine bat population after extermination attempts, Uganda. Emerg Infect Dis J. 2014;20(10):1761. DOI:10.3201/eid2010.140696.
- Amman BR, Bird BH, Bakarr IA, et al. Isolation of Angola-like Marburg virus from Egyptian rousette bats from West Africa. Nat Commun. 2020;11(1):510. DOI:10.1038/s41467-020-14327-8.
- Pawęska J, Storm N, Markotter W, et al. Shedding of Marburg virus in naturally infected Egyptian Rousette bats, South Africa, 2017. Emerg Infect Dis J. 2020;26(12):3051. DOI:10.3201/eid2612.202108.
- Kajihara M, Hang’-Ombe BM, Changula K, et al. Marburg virus in Egyptian Fruit Bats, Zambia. Emerg Infect Dis J. 2019;25(8):1577. DOI:10.3201/eid2508.190268.
- Schuh AJ, Amman BR, Jones MEB, et al. Modelling filovirus maintenance in nature by experimental transmission of Marburg virus between Egyptian rousette bats. Nat Commun. 2017;8(1):14446. DOI:10.1038/ncomms14446.
- Paweska JT, Jansen van Vuren P, Masumu J, et al. Virological and serological findings in rousettus aegyptiacus experimentally inoculated with vero cells-adapted hogan strain of Marburg virus. PLoS One. 2012;7(9):e45479. DOI:10.1371/journal.pone.0045479.
- Kortepeter MG, Dierberg K, Shenoy ES, et al. Marburg virus disease: a summary for clinicians. Inter J Infect Dis. 2020;99:233–242.
- Jones MEB, Schuh A, Amman B, et al. Experimental inoculation of Egyptian rousette bats (Rousettus aegyptiacus) with viruses of the Ebolavirus and Marburgvirus Genera. Viruses. 2015;7(7):3420–3442. DOI:10.3390/v7072779.
- Amman BR, Schuh AJ, Albariño CG, et al. Marburg Virus persistence on Fruit as a Plausible Route of Bat to Primate Filovirus Transmission. Viruses. 2021;13(12):2394. DOI:10.3390/v13122394.
- Luby JP, Sanders CV. Green monkey disease (“Marburg Virus” Disease): a new zoonosis. Ann Intern Med. 1969;71(3):657–660.
- Siegert R, Shu H-L, Slenczka W, et al. Zur Ätiologie einer unbekannten, von affen ausgegangenen menschlichen Infektionskrankheit. Dtsch Med Wochenschr. 1967;92(51):2341–2343. DOI:10.1055/s-0028-1106144.
- Martini GA, Knauff HG, Schmidt HA, et al. Über eine bisher unbekannte, von Affen eingeschleppte Infektionskrankheit: Marburg-Virus-Krankheit. Dtsch Med Wochenschr. 1968;93(12):559–571. DOI:10.1055/s-0028-1105098.
- Coffin KM, Liu J, Warren TK, et al. Persistent Marburg virus infection in the testes of nonhuman primate survivors. Cell Host Microbe. 2018;24(3):405–416.e3. DOI:10.1016/j.chom.2018.08.003.
- Martini GA. Marburg virus disease. Postgrad Med J. 1973;49(574):542–546.
- Borchert M, Muyembe-Tamfum JJ, Colebunders R, et al. Short communication: a cluster of Marburg virus disease involving an infant. Trop Med Int Health. 2002;7(10):902–906. DOI:10.1046/j.1365-3156.2002.00945.x.
- Deen GF, Broutet N, Xu W, et al. Ebola RNA Persistence in Semen of Ebola Virus Disease Survivors — Final Report. N Engl J Med. 2015;377(15):1428–1437. DOI:10.1056/NEJMoa1511410.
- Thorson AE, Deen GF, Bernstein KT, et al. Persistence of Ebola virus in semen among Ebola virus disease survivors in Sierra Leone: a cohort study of frequency, duration, and risk factors. PLoS Med. 2021;18(2):e1003273. DOI:10.1371/journal.pmed.1003273.
- Uyeki TM, Erickson BR, Brown S, et al. Ebola Virus Persistence in Semen of Male Survivors. Clinl Infect Dis. 2016;62(12):1552–1555. DOI:10.1093/cid/ciw202.
- Bausch DG, Borchert M, Grein T, et al. Risk factors for Marburg hemorrhagic fever, Democratic Republic of the Congo. Emerg Infect Dis J. 2003;9(12):1531. DOI:10.3201/eid0912.030355.
- Johnston SC, Lin KL, Twenhafel NA, et al. Dose response of MARV/Angola infection in cynomolgus macaques following IM or aerosol Exposure. PLoS One. 2015;10(9):e0138843. DOI:10.1371/journal.pone.0138843.
- Piercy TJ, Smither SJ, Steward JA, et al. The survival of filoviruses in liquids, on solid substrates and in a dynamic aerosol. J Appl Microbiol. 2010;109(5):1531–1539. DOI:10.1111/j.1365-2672.2010.04778.x.
- Klenk H-D, Slenczka W, Feldmann H. Marburg and Ebola Viruses (Filoviridae). In: Granoff A and RG Webster, editors. Encyclopedia of Virology. 2nd ed . Oxford: Elsevier; 1999. pp. 939–945.
- Nyakarahuka L, Kankya C, Krontveit R, et al. How severe and prevalent are Ebola and Marburg viruses? a systematic review and meta-analysis of the case fatality rates and seroprevalence. BMC Infect Dis. 2016;16(1):708. DOI:10.1186/s12879-016-2045-6.
- Slenczka WG. The Marburg virus outbreak of 1967 and subsequent episodes. Curr Top Microbiol Immunol. 1999;235:49–75.
- Stille W, Böhle E. Clinical course and prognosis of Marburg Virus (“Green-Monkey”) Disease. In: Martini GA and R Siegert, Stille W and Böhle E. editors. Marburg Virus Disease. Berlin, Heidelberg: Springer Berlin Heidelberg; 1971. pp. 10–18.
- Borchert M, Mulangu S, Lefèvre P, et al. Use of protective gear and the occurrence of occupational Marburg hemorrhagic fever in health workers from watsa health zone, democratic republic of the congo. J Infect Dis. 2007;196(Supplement_2):S168–S175. DOI:10.1086/520540.
- Kuhn JH. Filoviruses - a compendium of 40 years of epidemiological, clinical, and laboratory studies. 2008/07/22 ed Arch Virol Supplementa. 2008;20;13–360.
- Hartman AL, Towner JS, Nichol ST. Ebola and Marburg hemorrhagic fever. Clin Lab Med. 2010;30(1):161–177.
- Feldmann H. Marburg hemorrhagic fever — the forgotten cousin strikes. N Engl J Med. 2006;355(9):866–869.
- Leroy E. Chapter 199 - Filoviral Hemorrhagic Fever: Marburg and Ebola Virus Fevers. In: Feigin RD, et al., editors. Feigin and Cherry’s Textbook of Pediatric Infectious Diseases. 6th ed. Philadelphia:W.B. Saunders; 2009. pp. 2524–2531.
- Borchert M, Van der Stuyft P. Epidemiology and control of Marburg haemorrhagic fever epidemics in Central africa. Afrika Focus. 2008;22(1):118–119.
- Gaudin R, Goetz JG. Tracking mechanisms of viral dissemination in vivo. Trends Cell Biol. 2021;31(1):17–23.
- Berg T. Small-Molecule modulators of c-Myc/Max and max/Max interactions. In: Vassilev L and D Fry, . editors. Small-Molecule inhibitors of protein-protein interactions. Berlin, Heidelberg: Springer; 2011. pp. 139–149.
- Bhattacharyya S, Hope TJ. Cellular factors implicated in filovirus Entry. Adv Virol. 2013;2013:487585.
- Kolesnikova L, Bohil AB, Cheney RE, et al. Budding of Marburg virus is associated with filopodia. Cell Microbiol. 2007;9(4):939–951. DOI:10.1111/j.1462-5822.2006.00842.x.
- Kolesnikova L, Ryabchikova E, Shestopalov A, et al. Basolateral budding of Marburg virus: VP40 retargets viral glycoprotein GP to the basolateral surface. J Infect Dis. 2007;196(Supplement_2):S232–S236. DOI:10.1086/520584.
- Schnittler H-J, Feldmann H. Marburg and Ebola hemorrhagic fevers: does the primary course of infection depend on the accessibility of organ-specific macrophages? Clinl Infect Dis. 1998;27(2):404–406.
- Shifflett K, Marzi A. Marburg virus pathogenesis – differences and similarities in humans and animal models. Virol J. 2019;16(1):165.
- Geisbert TW, Jaax NK. Marburg hemorrhagic fever: report of a case studied by immunohistochemistry and electron microscopy. Ultrastruct Pathol. 1998;22(1):3–17.
- Zaki SR, Shieh W-J, Greer P, et al. A novel immunohistochemical assay for the detection of Ebola virus in skin: implications for diagnosis, spread, and surveillance of Ebola hemorrhagic fever. J Infect Dis. 1999;179(Supplement_1):S36–S47. DOI:10.1086/514319.
- Becker S, Spiess M, Klenk H-D. The asialoglycoprotein receptor is a potential liver-specific receptor for Marburg virus. J Gen Virol. 1995;76(2):393–399.
- Kiley MP, et al. Filoviridae: marburg and ebola viruses, in laboratory diagnosis of infectious diseases principles and practice: VOLUME II viral. In: Lennette EH editor. Rickettsial, and chlamydial diseases. New York, NY: Springer; 1988. pp. 595–601.
- Marty AM, Jahrling PB, Geisbert TW. Viral hemorrhagic fevers. Clin Lab Med. 2006;26(2):345–386.
- Hensley LE, Alves DA, Geisbert JB, et al. Pathogenesis of Marburg hemorrhagic fever in cynomolgus macaques. J Infect Dis. 2011;204(suppl_3):S1021–S1031. DOI:10.1093/infdis/jir339.
- Martines RB, Ng DL, Greer PW, et al. Tissue and cellular tropism, pathology and pathogenesis of Ebola and Marburg viruses. J Pathol. 2015;235(2):153–174. DOI:10.1002/path.4456.
- Zaki SR, Goldsmith C. Pathologic features of filovirus infections in humans. Curr Top Microbiol Immunol. 1999;235:97–116.
- Koch B, Dolnik O, Filzmayer M, et al. FP217 Marburg virus & acute kidney injury. Nephrol Dialysis Transplantation. 2018;33(suppl_1):104. DOI:10.1093/ndt/gfy104.FP217.
- Nkoghe D, Leroy EM, Toung-Mve M, et al. Cutaneous manifestations of filovirus infections. Int J Dermatol. 2012;51(9):1037–1043. DOI:10.1111/j.1365-4632.2011.05379.x.
- Groß JV, Slanger TE, Cullen P, et al. Stopping possible sexual transmission of filoviruses. Clinl Infect Dis. 2015;60(12):1871–1872. DOI:10.1093/cid/civ188.
- Martini GA, Schmidt HA. Spermatogene übertragung des „virus Marburg“. Klinische Wochenschrift. 1968;46(7):398–400.
- Zapata JC, Cox D, Salvato MS. The role of platelets in the pathogenesis of viral hemorrhagic fevers. PLoS Negl Trop Dis. 2014;8(6):e2858.
- Schnittler HJ, Mahner F, Drenckhahn D, et al. Replication of Marburg virus in human endothelial cells. a possible mechanism for the development of viral hemorrhagic disease. J Clin Invest. 1993;91(4):1301–1309. DOI:10.1172/JCI116329.
- Fisher-Hoch SP, Platt GS, Neild GH, et al. Pathophysiology of shock and hemorrhage in a fulminating viral infection (Ebola). J Infect Dis. 1985;152(5):887–894. DOI:10.1093/infdis/152.5.887.
- Messaoudi I, Amarasinghe GK, Basler CF. Filovirus pathogenesis and immune evasion: insights from Ebola virus and Marburg virus. Nature Rev Microbiol. 2015;13(11):663–676.
- Ramanan P, Shabman RS, Brown CS, et al. Filoviral immune evasion Mechanisms. Viruses. 2011;3(9):1634–1649. DOI:10.3390/v3091634.
- Valmas C, Grosch MN, Schümann M, et al. Marburg virus evades interferon Responses by a mechanism distinct from Ebola virus. PLoS Pathog. 2010;6(1):e1000721. DOI:10.1371/journal.ppat.1000721.
- Fritz EA, Geisbert JB, Geisbert TW, et al. Cellular immune response to Marburg virus infection in cynomolgus macaques. Viral Immunol. 2008;21(3):355–364. DOI:10.1089/vim.2008.0023.
- Kash JC, Muhlberger E, Carter V, et al. Global suppression of the host antiviral response by Ebola- and Marburg viruses: increased antagonism of the type I interferon response is associated with enhanced virulence. J Virol. 2006;80(6):3009–3020. DOI:10.1128/JVI.80.6.3009-3020.2006.
- Ramanan P. Structural and biochemical characterization of Marburg virus VP35 and its role in immune evasion. Iowa State University; 2012.
- Basler CF, Mikulasova A, Martinez-Sobrido L, et al. The Ebola virus VP35 protein inhibits activation of interferon regulatory factor 3. J Virol. 2003;77(14):7945–7956. DOI:10.1128/JVI.77.14.7945-7956.2003.
- Cardenas WB, Loo Y-M, Gale M, et al. Ebola virus VP35 protein binds double-stranded RNA and inhibits alpha/Beta interferon production induced by RIG-I signaling. J Virol. 2006;80(11):5168–5178. DOI:10.1128/JVI.02199-05.
- Reid SP, Leung LW, Hartman AL, et al. Ebola virus VP24 binds karyopherin α1 and blocks STAT1 nuclear accumulation. J Virol. 2006;80(11):5156–5167. DOI:10.1128/JVI.02349-05.
- Rougeron V, Feldmann H, Grard G, et al. Ebola and Marburg haemorrhagic fever. J Clin Virol. 2015;64:111–119.
- Simmons G. Filovirus entry. In: Pöhlmann S and G Simmons, . editors. Viral entry into host cells. New York, NY: Springer New York; 2013. pp. 83–94.
- Brouckaert P, Fiers W. Tumor necrosis factor and the systemic inflammatory response syndrome. In: Rietschel ET and H Wagner, Brouckaert P and Fiers W. editors. Pathology of septic shock. Berlin, Heidelberg: Springer Berlin Heidelberg; 1996. pp. 167–187.
- Brauburger K, Hume AJ, Mühlberger E, et al. Forty-Five years of Marburg virus research. Viruses. 2012;4(10):1878–1927. DOI:10.3390/v4101878.
- Stonier SW, Herbert AS, Kuehne AI, et al. Marburg virus survivor immune responses are th1 skewed with limited neutralizing antibody responses. J Exp Med. 2017;214(9):2563–2572. DOI:10.1084/jem.20170161.
- Mohamadzadeh M, Chen L, Schmaljohn AL. How Ebola and Marburg viruses battle the immune system. Nat Rev Immunol. 2007;7(7):556–567.
- Kortepeter MG, Bausch DG, Bray M. Basic clinical and laboratory features of filoviral hemorrhagic fever. J Infect Dis. 2011;204(suppl_3):S810–S816.
- Bosio CM, Aman M&, Grogan C, et al. Ebola and Marburg viruses replicate in monocyte-derived dendritic Cells without inducing the production of cytokines and full Maturation. J Infect Dis. 2003;188(11):1630–1638. DOI:10.1086/379199.
- Basler CF, Amarasinghe GK. Evasion of interferon responses by Ebola and Marburg viruses. J Interferon Cytokine Res. 2009;29(9):511–520.
- Bixler SL, Goff AJ. The role of cytokines and Chemokines in filovirus infection. Viruses. 2015;7(10):5489–5507.
- Leroy EM, Gonzalez JP, Baize S. Ebola and Marburg haemorrhagic fever viruses: major scientific advances, but a relatively minor public health threat for africa. Clin Microbiol Infect. 2011;17(7):964–976.
- Adegboro B, Adeola O. Marburg haemorrhagic fever: recent advances. Afr J Clin Exp Microbiol. 2011;12(2). DOI:10.4314/ajcem.v12i2.64322
- Bray M, Paragas J. Experimental therapy of filovirus infections. Antiviral Res. 2002;54(1):1–17.
- Strickland-Cholmley M, Malherbe H. Examination of south african primates for the presence of Marburg virus. In: Martini GA and R Siegert, Strickland-Cholmley M and Malherbe H. editors. Marburg virus disease. Berlin, Heidelberg: Springer Berlin Heidelberg; 1971. pp. 195–202.
- Ascenzi P, Bocedi A, Heptonstall J, et al. Ebolavirus and marburgvirus: insight the Filoviridae family. Mol Aspects Med. 2008;29(3):151–185. DOI:10.1016/j.mam.2007.09.005.
- Simpson DIH. Marburg agent disease: in monkeys. Trans R Soc Trop Med Hyg. 1969;63(3):303–309.
- Cooper TK, Sword J, Johnson JC, et al. New insights into Marburg virus disease pathogenesis in the rhesus macaque model. J Infect Dis. 2018;218(suppl_5):S423–S433. DOI:10.1093/infdis/jiy367.
- Bausch DG, Sprecher AG, Jeffs B, et al. Treatment of Marburg and Ebola hemorrhagic fevers: a strategy for testing new drugs and vaccines under outbreak conditions. Antiviral Res. 2008;78(1):150–161. DOI:10.1016/j.antiviral.2008.01.152.
- Lavender KJ, Williamson BN, Saturday G, et al. Pathogenicity of Ebola and Marburg viruses is associated with differential activation of the myeloid compartment in humanized triple knockout-bone marrow, liver, and thymus mice. J Infect Dis. 2018;218(suppl_5):S409–S417. DOI:10.1093/infdis/jiy269.
- Wei H, Audet J, Wong G, et al. Deep-Sequencing of Marburg virus genome during sequential mouse passaging and cell-culture adaptation reveals extensive changes over time. Sci Rep. 2017;7(1):3390. DOI:10.1038/s41598-017-03318-3.
- Warfield KL, Bradfute SB, Wells J, et al. Development and characterization of a mouse model for Marburg hemorrhagic fever. J Virol. 2009;83(13):6404–6415. DOI:10.1128/JVI.00126-09.
- Simpson DI, Zlotnik I, Rutter DA. Vervet monkey disease. experiment infection of guinea pigs and monkeys with the causative agent. Br J Exp Pathol. 1968;49(5):458–464.
- Wong G, Cao W-G, He S-H, et al. Development and characterization of a guinea pig model for Marburg virus. Zool Res. 2018;39(1):32–41. DOI:10.24272/j.issn.2095-8137.2017.054.
- Mire CE, Geisbert JB, Versteeg KM, et al. A single-vector, single-injection trivalent filovirus vaccine: proof of concept study in outbred guinea pigs. J Infect Dis. 2015;212(Suppl 2):S384–8.
- Atkins C, Miao J, Kalveram B, et al. Natural history and pathogenesis of wild-type Marburg virus infection in STAT2 knockout Hamsters. J Infect Dis. 2018;218(suppl_5):S438–S447. DOI:10.1093/infdis/jiy457.
- Marzi A, Banadyga L, Haddock E, et al. A hamster model for Marburg virus infection accurately recapitulates Marburg hemorrhagic fever. Sci Rep. 2016;6(1):39214. DOI:10.1038/srep39214.
- Geisbert TW, Strong JE, Feldmann H. Considerations in the use of nonhuman primate models of Ebola virus and Marburg virus infection. J Infect Dis. 2015;212(suppl_2):S91–S97.
- Geisbert TW, Daddario‐dicaprio K, Geisbert J, et al. Marburg virus Angola infection of rhesus macaques: pathogenesis and treatment with recombinant nematode anticoagulant protein c2. J Infect Dis. 2007;196(Supplement_2):S372–S381. DOI:10.1086/520608.
- Wong G, Zhang Z, He S, et al. Marburg and ravn virus infections do not cause observable disease in ferrets. J Infect Dis. 2018;218(suppl_5):S471–S474. DOI:10.1093/infdis/jiy245.
- Cross RW, Mire CE, Agans KN, et al. Marburg and ravn viruses fail to cause disease in the domestic ferret (Mustela putorius furo). J Infect Dis. 2018;218(suppl_5):S448–S452. DOI:10.1093/infdis/jiy268.
- Jeffs B. A clinical guide to viral haemorrhagic fevers: Ebola, Marburg and lassa. Trop Doct. 2006;36(1):1–4.
- Cross RW, Mire CE, Feldmann H, et al. Post-Exposure treatments for Ebola and Marburg virus infections. Nat Rev Drug Discov. 2018;17(6):413–434. DOI:10.1038/nrd.2017.251.
- Clark DV, Jahrling PB, Lawler JV. Clinical management of filovirus-infected patients. Viruses. 2012;4(9):1668–1686.
- WHO. Ebola and Marburg virus disease epidemics: preparedness, alert, control, and evaluation. Geneva: World Health Organization; 2014.
- Todorovitch K, Mocitch M, Klašnja R. Clinical picture of two patients infected by the marburg vervet virus. In: Martini GA and R Siegert, Todorovitch K, Mocitch M, and Klašnja R. editors. Marburg virus disease. Berlin, Heidelberg: Springer Berlin Heidelberg; 1971. pp. 19–23.
- Frean J. The memoirs of margaretha isaacson. Vol. 9. Brisbane, Australia: Australasian College of Tropical Medicine; 2008. pp. 32–52.
- Ndayimirije N, Kindhauser MK. Marburg hemorrhagic fever in Angola — fighting fear and a lethal pathogen. N Engl J Med. 2005;352(21):2155–2157.
- Jeffs B, Roddy P, Weatherill D, et al. The médecins sans frontières intervention in the Marburg hemorrhagic fever epidemic, uige, Angola, 2005. I. lessons learned in the hospital. J Infect Dis. 2007;196(Supplement_2):S154–S161. DOI:10.1086/520548.
- Roddy P, Weatherill D, Jeffs B, et al. The médecins sans frontières intervention in the Marburg hemorrhagic fever epidemic, uige, Angola, 2005. II. lessons learned in the community. J Infect Dis. 2007;196(Supplement_2):S162–S167. DOI:10.1086/520544.
- Schultz MJ, Deen J, von Seidlein L, et al. Remote-Controlled and pulse pressure–Guided fluid treatment for adult patients with viral hemorrhagic fevers. Am J Trop Med Hyg. 2021;104(4):1172–1175. DOI:10.4269/ajtmh.20-1515.
- Porter DP, Weidner JM, Gomba L, et al. Remdesivir (GS-5734) is efficacious in cynomolgus macaques infected with Marburg virus. J Infect Dis. 2020;222(11):1894–1901. DOI:10.1093/infdis/jiaa290.
- Pessi A, Bixler SL, Soloveva V, et al. Cholesterol-Conjugated stapled peptides inhibit Ebola and Marburg viruses in vitro and in vivo. Antiviral Res. 2019;171:104592.
- Gaisina IN, Peet NP, Wong L, et al. Discovery and structural optimization of 4-(aminomethyl)benzamides as potent entry inhibitors of Ebola and Marburg virus infections. J Med Chem. 2020;63(13):7211–7225. DOI:10.1021/acs.jmedchem.0c00463.
- Zhang L, Lei S, Xie H, et al. Screening and identification of Marburg virus entry inhibitors using approved drugs. Virol Sin. 2020;35(2):235–239. DOI:10.1007/s12250-019-00184-3.
- Edwards MR, Basler CF. Current status of small molecule drug development for Ebola virus and other filoviruses. Curr Opin Virol. 2019;35:42–56.
- Han Z, Ye H, Liang J, et al. Compound FC-10696 inhibits egress of Marburg virus. Antimicrob Agents Chemother. 2021;65(7):e00086–21. DOI:10.1128/AAC.00086-21.
- Heald AE, Charleston JS, Iversen PL, et al. AVI-7288 for Marburg virus in nonhuman primates and humans. N Engl J Med. 2015;373(4):339–348. DOI:10.1056/NEJMoa1410345.
- Dulin N, Spanier A, Merino K, et al. Systematic review of Marburg virus vaccine nonhuman primate studies and human clinical trials. Vaccine. 2021;39(2):202–208. DOI:10.1016/j.vaccine.2020.11.042.
- Suschak JJ, Schmaljohn CS. Vaccines against Ebola virus and Marburg virus: recent advances and promising candidates. Hum Vaccines Immunother. 2019;15(10):2359–2377.
- Trovato M, Sartorius R, D’-Apice L, et al. Viral emerging diseases: challenges in developing vaccination strategies. Front Immunol. 2020;11(2130). DOI:10.3389/fimmu.2020.02130.
- Roozendaal R, Hendriks J, van Effelterre T, et al. Nonhuman primate to human immunobridging to infer the protective effect of an Ebola virus vaccine candidate. NPJ Vaccines. 2020;5(1):112. DOI:10.1038/s41541-020-00261-9.
- Sarwar UN, Costner P, Enama ME, et al. Safety and immunogenicity of DNA vaccines encoding ebolavirus and marburgvirus wild-type glycoproteins in a phase I clinical trial. J Infect Dis. 2014;211(4):549–557. DOI:10.1093/infdis/jiu511.
- Kibuuka H, Berkowitz NM, Millard M, et al. Safety and immunogenicity of Ebola virus and Marburg virus glycoprotein DNA vaccines assessed separately and concomitantly in healthy ugandan adults: a phase 1b, randomised, double-blind, placebo-controlled clinical trial. Lancet. 2015;385(9977):1545–1554. DOI:10.1016/S0140-6736(14)62385-0.
- Mire CE, Geisbert JB, Agans KN, et al. Durability of a vesicular stomatitis virus-based Marburg virus vaccine in nonhuman primates. PLoS One. 2014;9(4):e94355. DOI:10.1371/journal.pone.0094355.
- Hargreaves A, Brady C, Mellors J, et al. Filovirus neutralising antibodies: mechanisms of action and therapeutic application. Pathogens. 2021;10(9):1201. DOI:10.3390/pathogens10091201.
- Dye JM, Warfield K, Wells J, et al. Virus-Like particle vaccination protects nonhuman primates from lethal aerosol exposure with marburgvirus (VLP vaccination protects macaques against aerosol challenges). Viruses. 2016;8(4):94. DOI:10.3390/v8040094.
- Swenson DL, Warfield KL, Larsen T, et al. Monovalent virus-like particle vaccine protects guinea pigs and nonhuman primates against infection with multiple Marburg viruses. Expert Rev Vaccines. 2008;7(4):417–429. DOI:10.1586/14760584.7.4.417.
- Preston KB, Wong TAS, To A, et al. Single-Vial filovirus glycoprotein vaccines: biophysical characteristics and immunogenicity after co-lyophilization with adjuvant. Vaccine. 2021;39(39):5650–5657. DOI:10.1016/j.vaccine.2021.08.003.
- Lehrer AT, Chuang E, Namekar M, et al. Recombinant protein filovirus vaccines protect cynomolgus macaques from Ebola, Sudan, and Marburg viruses. Front Immunol. 2021;12(3287). DOI:10.3389/fimmu.2021.703986.
- Cross RW, Bornholdt ZA, Prasad AN, et al. Combination therapy protects macaques against advanced Marburg virus disease. Nat Commun. 2021;12(1):1891. DOI:10.1038/s41467-021-22132-0.
- Mire CE, Geisbert JB, Borisevich V, et al. Therapeutic treatment of Marburg and Ravn virus infection in nonhuman primates with a human monoclonal antibody. Sci Transl Med. 2017;9(384):eaai8711. DOI:10.1126/scitranslmed.aai8711.
- Dye JM, Herbert AS, Kuehne AI, et al. Postexposure antibody prophylaxis protects nonhuman primates from filovirus disease. Proc Nat Acad Sci. 2012;109(13):5034–5039. DOI:10.1073/pnas.1200409109.
- Warren TK, Wells J, Panchal RG, et al. Protection against filovirus diseases by a novel broad-spectrum nucleoside analogue BCX4430. Nature. 2014;508(7496):402–405. DOI:10.1038/nature13027.
- Thi EP, Mire CE, Ursic-Bedoya R, et al. Marburg virus infection in nonhuman primates: therapeutic treatment by lipid-encapsulated siRNA. Sci Transl Med. 2014;6(250):250ra116–250ra116. DOI:10.1126/scitranslmed.3009706.
- Thi EP, Mire CE, Lee ACH, et al. siRNA rescues nonhuman primates from advanced Marburg and Ravn virus disease. J Clin Invest. 2017;127(12):4437–4448. DOI:10.1172/JCI96185.
- Warren TK, Warfield KL, Wells J, et al. Advanced antisense therapies for postexposure protection against lethal filovirus infections. Nat Med. 2010;16(9):991–994. DOI:10.1038/nm.2202.
- Warren TK, Whitehouse CA, Wells J, et al. Delayed time-to-treatment of an antisense morpholino oligomer is effective against lethal Marburg virus infection in cynomolgus macaques. PLoS Negl Trop Dis. 2016;10(2):e0004456. DOI:10.1371/journal.pntd.0004456.
- Daddario-DiCaprio KM, Geisbert TW, Ströher U, et al. Postexposure protection against Marburg haemorrhagic fever with recombinant vesicular stomatitis virus vectors in non-human primates: an efficacy assessment. Lancet. 2006;367(9520):1399–1404. DOI:10.1016/S0140-6736(06)68546-2.
- Geisbert TW, Hensley LE, Geisbert JB, et al. Postexposure treatment of Marburg virus infection. Emerg Infect Dis. 2010;16(7):1119–1122. DOI:10.3201/eid1607.100159.
- Jones SM, Feldmann H, Ströher U, et al. Live attenuated recombinant vaccine protects nonhuman primates against Ebola and Marburg viruses. Nat Med. 2005;11(7):786–790. DOI:10.1038/nm1258.
- Daddario-DiCaprio Kathleen M, Geisbert TW, Geisbert JB, et al. Cross-Protection against Marburg virus strains by using a live, attenuated recombinant vaccine. J Virol. 2006;80(19):9659–9666. DOI:10.1128/JVI.00959-06.
- Geisbert TW, Daddario-DiCaprio KM, Geisbert JB, et al. Vesicular stomatitis virus-based vaccines protect nonhuman primates against aerosol challenge with Ebola and Marburg viruses. Vaccine. 2008;26(52):6894–6900. DOI:10.1016/j.vaccine.2008.09.082.
- Geisbert Thomas W, Geisbert JB, Leung A, et al. Single-injection vaccine protects nonhuman primates against infection with Marburg virus and three species of Ebola virus. J Virol. 2009;83(14):7296–7304. DOI:10.1128/JVI.00561-09.
- Riemenschneider J, Garrison A, Geisbert J, et al. Comparison of individual and combination DNA vaccines for B. anthracis, Ebola virus, Marburg virus and Venezuelan equine encephalitis virus. Vaccine. 2003;21(25):4071–4080. DOI:10.1016/S0264-410X(03)00362-1.
- Geisbert TW, Bailey M, Geisbert JB, et al. Vector choice determines immunogenicity and potency of genetic vaccines against Angola Marburg virus in nonhuman primates. J Virol. 2010;84(19):10386–10394. DOI:10.1128/JVI.00594-10.
- Grant-Klein RJ, Altamura LA, Badger CV, et al. Codon-Optimized filovirus DNA vaccines delivered by intramuscular electroporation protect cynomolgus macaques from lethal Ebola and Marburg virus challenges. Hum Vaccines Immunother. 2015;11(8):1991–2004. DOI:10.1080/21645515.2015.1039757.
- Hevey M, Negley D, Pushko P, et al. Marburg virus vaccines based upon alphavirus replicons protect guinea pigs and nonhuman primates. Virology. 1998;251(1):28–37. DOI:10.1006/viro.1998.9367.
- Swenson DL, Wang D, Luo M, et al. Vaccine to confer to nonhuman primates complete protection against multistrain Ebola and Marburg virus Infections. Clin Vaccin Immunol. 2008;15(3):460–467. DOI:10.1128/CVI.00431-07.
- Ignatyev GM, Agafonov AP, Streltsova MA, et al. Inactivated Marburg virus elicits a nonprotective immune response in Rhesus monkeys. J Biotechnol. 1996;44(1):111–118. DOI:10.1016/0168-1656(95)00104-2.
- Smith LM, Hensley LE, Geisbert TW, et al. Interferon-β therapy prolongs survival in rhesus macaque models of Ebola and Marburg hemorrhagic fever. J Infect Dis. 2013;208(2):310–318. DOI:10.1093/infdis/jis921.