ABSTRACT
In pathogenic fungi, calcium-calmodulin-dependent serine-threonine-specific phosphatase calcineurin is involved in morphogenesis and virulence. Therefore, calcineurin and its tightly related protein complexes are attractive antifungal drug targets. However, there is limited knowledge available on the relationship between in vivo Ca2+-binding sites of calmodulin (CaM) and its functions in regulating stress responses, morphogenesis, and pathogenesis. In the current study, we demonstrated that calmodulin is required for hyphal growth, conidiation, and virulence in the human fungal pathogen, Aspergillus fumigatus. Site-directed mutations of calmodulin revealed that a single Ca2+-binding site mutation had no significant effect on A. fumigatus hyphal development, but multiple Ca2+-binding site mutations exhibited synergistic effects, especially when cultured at 42 °C, indicating that calmodulin function in response to temperature stress depends on its Ca2+-binding sites. Western blotting implied that mutations in Ca2+-binding sites caused highly degraded calmodulin fragments, suggesting that the loss of Ca2+-binding sites results in reduced protein stability. Moreover, normal intracellular calcium homeostasis and the nuclear translocation of the transcriptional factor CrzA are dependent on Ca2+-binding sites of AfCaM, demonstrating that Ca2+-binding sites of calmodulin are required for calcium signalling and its major transcription factor CrzA. Importantly, in situ mutations for four Ca2+-binding sites of calmodulin resulted in an almost complete loss of virulence in the Galleria mellonella wax moth model. This study shed more light on the functional characterization of putative calcium-binding sites of calmodulin in the morphogenesis and virulence of A. fumigatus, which enhances our understanding of calmodulin biological functions in cells of opportunistic fungal pathogens.
Introduction
As an intracellular secondary messenger in eukaryotic cells, Ca2+ plays a crucial role in regulating various cellular processes, including secretion, synaptic transmission, and cytokinesis [Citation1,Citation2]. The intracellular Ca2+ concentration is strictly and precisely controlled by a sophisticated calcium homeostasis system, which consists of various calcium channels, calcium pumps, and calcium antiporters [Citation2–4]. Calmodulin (CaM), a Ca2+-binding protein with predicted 3–4 EF-hands to bind calcium, interacts with many other proteins in the cell, and acts as a signalling molecule in a wide variety of cellular functions [Citation5–7]. In humans, CaM is encoded by three independent genes, is a 148 amino acid, highly conserved calcium sensor protein that contains four EF-hand Ca2+-binding motifs [Citation8]. In the model yeast Schizosaccharomyces pombe, Saccharomyces cerevisiae and the filamentous fungus Aspergillus nidulans, CaM is encoded by a single gene, and Ca2+ binding to CaM is required for activation in S. pombe and A. nidulans. However, only the Ca2+-independent functions of CaM are essential for growth in the budding yeast S. cerevisiae. CaM from S. cerevisiae binds only three Ca2+ ions, with the fourth EF-hand motif being not required for binding calcium [Citation5], whereas CaM from vertebrate systems binds four Ca2+ ions with each EF-hand motif [Citation9]. Although CaM is necessary for the development of budding yeast, high-affinity Ca2+ binding to CaM is not necessary because the yeast can thrive when CaM is mutated [Citation10]. In contrast to S. cerevisiae, high-affinity Ca2+ binding to CaM is required to support the growth of S. pombe and A. nidulans [Citation6,Citation11]. Crz1/CrzA, a calcineurin-responsive zinc finger 1 transcription factor, is the most well-studied downstream target of calcineurin [Citation12–15]. It has been demonstrated that once the concentration of intracellular calcium increases, CaM binds to calcium and activates calcineurin, leading to the dephosphorylation of Crz1 entering the nucleus in the cell [Citation14,Citation15]. Crz1 is involved in many biological processes in fungi, including hyphal growth, cell wall integrity, pathogenicity and stress response [Citation16–18]. Although great progress has been made in understanding CaM in model fungi, especially yeast, it provides an excellent roadmap but cannot substitute for studies on fungal pathogens. Fungal infections are becoming a very important concern for human health worldwide because an increasing number of immunocompromised patients are susceptible to such infections with a high mortality rate [Citation19]. Importantly, many lines of evidence have demonstrated that calcium signalling is involved in fungal virulence and antifungal resistance [Citation7,Citation13,Citation20–22]. Calcium signalling via CaM may be critical for stress responses in two non-pathogenic fungi, S. pombe and Aspergillus oryzae [Citation23,Citation24]. In the plant pathogenic fungi Colletotrichum trifolii and Pyricularia grisea, the development of a specialized infectious structure called the appressorium can be inhibited by the expression of CaM antisense RNA (C. trifolii) or by CaM antagonists and calcium chelators (M. grisea) [Citation25,Citation26]. Moreover, the cam gene from the human pathogen Paracoccidioides brasiliensis was investigated, and experiments using CaM antagonists revealed its role in the mycelial-to-yeast transition, which is essential for infection [Citation27]. Additionally, a Cryptococcus neoformans strain with a mutation in the cam gene (CaM1) has been obtained in vitro, which caused a marked reduction in CaM expression, leading to a growth defect at 37 °C [Citation28]. However, the role of CaM in the human pathogen Aspergillus fumigatus remains elusive.
Among species within the Aspergillus genus, A. fumigatus is the most ubiquitous aetiological factor of aspergillosis. Such evolutionary success is related to (1) small conidia size allows penetration to the lower respiratory tract system and escaping clearance by mucociliary forces; (2) the presence of melanin in the cell wall enables withstanding reactive oxygen species and phagocytosis; (3) abundance of negatively charged sialic acid on the surface permits A. fumigatus to effectively bind to the basal lamina proteins once inside the host lung [Citation29,Citation30]. More than 200,000 cases of invasive aspergillosis are thought to occur annually, with a 40–100% mortality rate [Citation30–32]. Furthermore, intrinsic and acquired resistance to antifungals in A. fumigatus results in reduced drug efficacy [Citation33]. To date, little is known about the function of A. fumigatus CaM. In this study, the functions of the four predicted A. fumigatus Ca2+-binding sites of CaM were verified. We discovered that there were no detectable differences in colony morphology between different single Ca2+-binding site mutants compared to their parental wild type, respectively, but mutations in multiple Ca2+-binding sites resulted in synergistic destructive effects. Ca2+-binding sites are especially required for A. fumigatus growth when cultured at 42 °C, implying that calcium binding to CaM is essential for fungal survival to deal with temperature stress. Notably, our study found that mutation of the Ca2+-binding sites in CaM results in reduced virulence. Collectively, this study provides direct evidence that Ca2+ binding-dependent CaM plays important roles in A. fumigatus development, temperature stress, and virulence performed in vivo on the model of Galleria mellonella.
Results
AfCaMis required for normal hyphal growth and conidiation
Based on a NCBI BLASTp search using the protein sequence of S. cerevisiae CaM as the query, we identified the predicted CaM in A. fumigatus, AfCaM (AFUB_067160). The predicted AfCaM open reading frame (ORF) includes 450 bp nucleotides with six introns and encodes a protein 149 amino acids in length. AfCaM is highly conserved as shown by its high identity with other fungal homologs such as A. nidulans (100%), S. cerevisiae (60%), and S. pombe (69%) (Fig. S1).
To explore the biological functions of CaM in A. fumigatus, we attempted to construct the full-length deletion strain Afcam many times using a homologous recombination strategy but were unsuccessful. This may be due to the essential role of CaM in A. fumigatus viability. Therefore, a conditional niiA-Afcam strain was generated, which conditionally induced Afcam under a nitrogen source of NO3− and repressed cam under NH4+ conditions. The correct construction was confirmed by diagnostic PCR analysis (Fig. S2). As shown in , the conditional niiA-Afcam strain displayed normal hyphal growth and conidiation on the inducing medium. Under repressing conditions, it exhibited no conidiation or hyphal growth when compared with wild type (WT) (). To verify that these defects are specifically caused by Afcam repression, we constructed an Afcam-complemented strain (Afcam-recon) by reintroducing a parental WT cam gene into conditional niiA-Afcam strain, allowing for genetic complementation when cultured on the repressing medium. Among transformants, the one that was able to show a comparable colony growth phenotype compared to that of the WT strain under repressing conditions was picked (), suggesting that the complemented cam gene can rescue the growth defects when cultured on cam repression conditions in A. fumigatus. The relative quantitative analysis to for colony growth and conidiation is shown in . These results suggest that cam is required for hyphal growth and conidiation in A. fumigatus.
Figure 1. CaM is essential for A. fumigatus hyphal growth and conidiation. (a) colony morphology of the indicated cam strains on the inducing or repressing medium at 37 °C for 2.5 days. Bar, 0.5 cm. (b) relative quantitative analysis for the colony diameter and (c) conidiation of the WT, niiA-Afcam and Afcam-recon strains under NO3− for niiA-induced condition and NH4+ for niiA-repressed condition. The data are presented as the mean ± standard deviation of three independent experiments. Statistical analysis was performed by Student’s t-test.
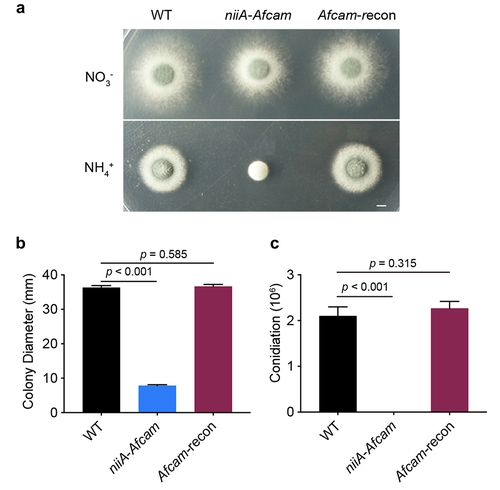
Four predicted Ca2+-binding EF-hands within CaM exert distinct functions in A. fumigatus
To dissect the structure of CaM, we used SMART protein analysis, which revealed that AfCaM contains four EF-hand motifs (). Each EF-hand provides an acidic loop, or “EF-loop,” for calcium coordination [Citation34,Citation35]. It has been reported that EF hands are required for calcium binding and CaM conformational changes [Citation36,Citation37]. To further explore the importance of four predicted Ca2+-binding loops for CaM function in A. fumigatus, we constructed the truncated mutant niiA-AfcamAfcam-T which removed the predicted EF-hand sequence encoding amino acids–21–32、57–68、94–105 and 130–141 in AfCaM. Then the Afcam-T gene was transferred to the conditional niiA-Afcam strain when cultured on the repressing medium. Therefore, there is one copy of niiA-Afcam and an additional copy of Afcam-T in transformants, referred to as niiA-AfcamAfcam-T. The defective phenotype of niiA-AfcamAfcam-T was similar to that of niiA-Afcam mutant under repressing conditions (), indicating that the lack of four EF-hands results in a complete loss of CaM functionality in A. fumigatus.
Figure 2. Four Ca2+-binding sites of A. fumigatus CaM exhibit different functions. (a) schematic diagram of the AfCaM and AfCaM-T. The Afcam-T gene was transferred to the conditional niiA-Afcam strain when cultured on the repressing medium. (b) colony morphology of the truncated mutant on YUU solid medium at 37 °C for 2.5 days. Bar, 0.5 cm. (c) the mutations in EF-loops of AfCaM. (d) colony morphology of the indicated cam mutants grown on YUU solid medium at 37 °C for 2.5 days. Bar, 0.5 cm. The residue numbering for AfCaM is based on the conserved CaM coding sequence. For example, CaMmut(1,2) is the simultaneous mutation of residues 1 and 2 of AfCaM Ca2+-binding sites. (e) relative quantitative analysis of the radial growth and (f) conidiation of the WT and cam mutants assessed after 2.5 days. The data are presented as the mean ± standard deviation of three independent experiments. Statistical analysis was performed using one-way ANOVA with multiple comparison tests. **p < 0.01; ns, not significant.
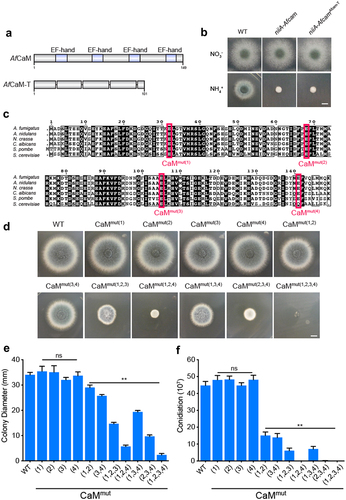
As the truncated mutant niiA-AfcamAfcam-T may lead to instability or conformational changes in AfCaM protein, we further investigated the functions of the Ca2+-binding sites in each EF-hand. Since previous studies in yeast have reported that the last amino acid of the EF-hand plays a key role in binding to calcium [Citation38], we constructed mutants in which the last conserved glutamic acid residue in each EF-hand was altered to alanine, while other residues remained unchanged by site-directed mutagenesis. These single mutations of E32A, E68A, E105A, and E141A in EF-loops are referred to as CaMmut(1), CaMmut(2), CaMmut(3), and CaMmut(4) based on the order of the four EF-hands (). As shown in , strains that embedded the individual mutations (CaMmut(1), CaMmut(2), CaMmut(3), CaMmut(4)) did not show any detectable differences compared to WT, whereas the double mutations CaMmut(1,2) and CaMmut(3,4) exhibited slight growth defects. Notably, the simultaneous mutations of residues 2 and 4 in AfCaM resulted in nearly no grown colonies of A. fumigatus (), implying that these two residues have key roles for colony growth and loss of both would significantly impair CaM function. In comparison, strains carrying CaMmut(1,2,3) and CaMmut(1,3,4) mutations showed less severe growth defects (). However, mutations in four Ca2+-binding sites of CaMmut(1,2,3,4) resulted in a serious defective phenotype of A. fumigatus (), suggesting that these combined four predicted Ca2+-binding sites of CaM are essential for A. fumigatus growth. Taken together, these data reveal that a single Ca2+-binding site mutation in AfCaM had no obvious influence on A. fumigatus hyphal development and conidiation, whereas multiple Ca2+-binding site mutations exhibited synergistic lethal effects.
Putative Ca2+-binding sites are important for CaM stabilization
To gain insight into the subcellular localization of CaM in A. fumigatus, we used niiA-Afcam as the background strain to construct niiA-AfcamGFP-CaM and niiA-AfcamGFP-CaM(1,2,3,4) which expressed Afcam and Afcam(1,2,3,4) fused with the N-terminal green fluorescent protein (GFP) under the control of its native promoter. Then we compared colony phenotypes of niiA-AfcamGFP-CaM and niiA-AfcamGFP-CaM(1,2,3,4) with the parental conditional strain under repressing conditions. Among transformants, the niiA-AfCaMGFP-CaM strain exhibited similar phenotypes to that of the WT strain under either expressing or repressing conditions (), implying that fused GFP with CaM could not affect CaM normal function. However, the colony phenotype of niiA-AfcamGFP-CaM(1,2,3,4) showed slight defects on the repressing medium (), suggesting that niiA-AfcamGFP-CaM (1,2,3,4) was unable to rescue functions of repressed CaM. This implies that mutations in CaM four EF-hands would affect CaM normal functions.
Figure 3. Four Ca2+-binding sites of CaM are required for its stabilization. (a) colony morphology of the WT、niiA-Afcam、niiA-CaMGFP-CaM(1,2,3,4) and niiA-CaMGFP-CaM strains on the inducing and repressing medium at 37 °C for 2.5 days. Bar, 0.5 cm. (b) localization of GFP-CaM strain at 6 h, 8 h, 10 h and 12 h, respectively. Bar, 5 μm. (c) western blotting analysis of the AfCaM conformational changes in the GFP-CaM and GFP-CaMmut(1,2,3,4) strains under 1 mM CaCl2 or 5 mM EGTA.
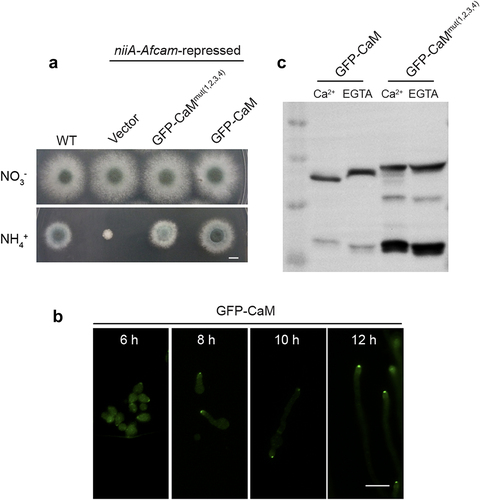
Notably, there was a discrepancy in colony phenotypes between CaMmut(1,2,3,4) in and niiA-AfcamGFP-CaM(1,2,3,4) in . Because the former one was generated by site-directed mutagenesis, resulting in nearly no grown colonies of A. fumigatus. However, the niiA-AfcamGFP-CaM(1,2,3,4) strain was constructed by reintroducing an Afcam(1,2,3,4) gene into the niiA-Afcam strain and then examined its function under repressing conditions, in which the niiA-Afcam expression could not be fully turn-off on the repressing medium (Fig. S2).
Fluorescence microscopy revealed that GFP-CaM was concentrated at the hyphal apex (). In addition, the protein expression of GFP-CaM and GFP-CaMmut(1,2,3,4) was detected using western blotting analysis. As shown in , GFP-CaM moved slightly faster by additional Ca2+ treatment compared to that without Ca2+ treatment, which reflects the conformational difference between the Ca2+-free and Ca2+-saturated forms of the protein. However, the mobilities of the mutant GFP-CaMmut(1,2,3,4) were almost identical in the presence or absence of Ca2+ (), implying that the mutations of the four Ca2+-binding sites resulted in a deficiency of calcium binding to CaM. This provides indirect evidence that mutations in all four Ca2+-binding sites could, to a great extent, affect Ca2+ affinity. However, the expression levels of GFP-CaMmut(1,2,3,4) were not significantly different from those of GFP-CaM (), indicating that the four Ca2+-binding mutations did not affect CaM expression. Notably, apart from the predicted mass of approximately 48 kDa (21.0 kDa of AfCaM plus 26.9 kDa of GFP), another band between 10 and 17 kDa was observed in the GFP- CaMmut(1,2,3,4) strain, but not in the GFP-CaM strain (), which was likely to be part of the degraded GFP-CaM fusion protein fragments. These findings suggest that the loss of key Ca2+-binding sites of CaM results in its destabilization.
The CaM function depends on its Ca2+-binding sites
Given that the activation of the calcium pathway through CaM plays an essential role in response to various environmental stresses [Citation21,Citation28], we assayed the phenotypes of relative Afcam mutants under high-temperature and osmotic stress. As shown in , when grown at 42 °C on the rich medium (YUU), CaMmut(1), CaMmut(3) and CaMmut(4) displayed similar phenotypes compared to those of WT. However, CaMmut(3,4) and CaMmut(1,3,4) exhibited relatively defective morphological phenotypes (). Interestingly, all mutants embedding the putative second calcium-binding loop, CaM2, including CaMmut(2), CaMmut(1,2), CaMmut(1,2,3), CaMmut(1,2,4), CaMmut(2,3,4) and CaMmut(1,2,3,4) showed hypersensitive phenotypes at 42 °C to some content (), suggesting that the second EF-hand plays a crucial role in adaptation to the heat stress. In comparison, sorbitol (1 M) did not cause significant changes in the colony phenotypes (Fig. S3). These data imply that the Afcam mutants that lacked Ca2+-binding sites would lead to the inability of A. fumigatus to grow at high temperatures. Collectively, these results indicate that CaM function in response to heat stress depends on Ca2+-binding sites.
Nuclear accumulation of CrzA induced by calcium relies on the Ca2+-binding ability of CaM
The transcriptional factor Crz1 is activated by the Ca2+/CaM/calcineurin pathway, and its nucleocytoplasmic trafficking requires fully functional calcineurin [Citation7]. To determine whether the binding of calcium in CaM affects the localization of CrzA (Crz1 ortholog in A. fumigatus), we constructed C-terminal GFP-tagged CrzA strains in the background of WT, CaMmut(1,2,4) and CaMmut(1,3,4) to visualize the trafficking of CrzA. As shown in , CrzA-GFP in the WT strain remained preferentially cytoplasmic in hyphal cells when cultured in normal minimal media (MM). As predicted, CrzA-GFP was predominantly induced by calcium (100 mM) from the cytosol to the nucleus (). However, nucleocytoplasmic trafficking of CrzA did not occur in either CaMmut(1,2,4) or CaMmut(1,3,4) strains, suggesting that Ca2+-binding sites are required for the trans-localization of CrzA from the cytoplasm to the nuclei in A. fumigatus.
Figure 5. Localization of CrzA-GFP in three indicated strains (WT、CaMmut(1,2,4)、CaMmut(1,3,4)). Strains were grown in minimal media (MM) for 10 h and observed by fluorescence microscopy with or without stimulation of 100 mM CaCl2. The nuclear localization of cells was visualized by DAPI staining. Bar, 10 μm.
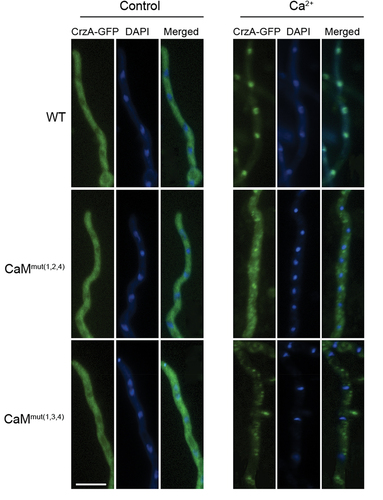
Mutations in Ca2+-binding sites lead to the disorder of cytoplasmic calcium homeostasis
As a well-characterized calcium sensor, CaM regulates cytoplasmic calcium homeostasis by activating various relevant targets, including calcium channels and transporters, in a Ca2+-dependent manner [Citation3,Citation12]. However, it is still unknown whether the disruption of Ca2+-binding sites influences intracellular calcium homeostasis in A. fumigatus. To examine this, we introduced codon-optimized aequorin into WT and CaMmut(1,2,4) strains to monitor extracellular calcium-induced [Ca2+]c transients in living cells. The strains that expressed codon-optimized aequorin [Citation39] were constructed by co-transforming the plasmid pAEQ containing aequorin and the selective marker hygromycin B phosphotransferase hyg gene into the indicated mutants. CaMmut(1,2,4) was chosen as a representative because it possesses a crucial second calcium-binding loop (CaM2), which allows it to exhibit a defective phenotype. As shown in , when a stimulus was treated with 100 mM CaCl2, the [Ca2+]c concentration in WT transiently increased from a resting level of approximately 0.07 μM to a peak of 0.74 μM. In comparison, the CaMmut(1,2,4) mutant showed a reduction of 42 ± 4% in the [Ca2+]c amplitude under the same stimulating conditions (), implying that mutated calcium-binding sites of CaM reduced extracellular calcium entry and also decreased calcium release from intracellular calcium stores to the cytosol. In contrast, the [Ca2+]c basal resting level in the CaMmut(1,2,4) mutant could not return to the normal basal level but instead showed a higher level of intracellular calcium concentration compared to that of WT (), suggesting that calcium binding of CaM affected calcium transport from intracellular to calcium stores. Thus, mutations in the Ca2+-binding site of CaM lead to a disorder of cytoplasmic calcium homeostasis.
Figure 6. Disorder of cytoplasmic calcium is caused by mutations in Ca2+-binding sites. (a) linear graphs indicating the real-time [Ca2+]c changes in response to calcium stimulus of WT and CaMmut(1,2,4) strains. [Ca2+]c, the free Ca2+ concentration in the cytosol. (b and c) the comparison of [Ca2+]c of WT and CaMmut(1,2,4) in dynamic and resting levels. The peak value is the peak level after the extracellular calcium stimulus. The basal level is the resting level prior to the extracellular calcium stimulus. Data are the average of three experiments. Error bars show the standard deviation. Statistical significance was determined by Student’s t-test.
![Figure 6. Disorder of cytoplasmic calcium is caused by mutations in Ca2+-binding sites. (a) linear graphs indicating the real-time [Ca2+]c changes in response to calcium stimulus of WT and CaMmut(1,2,4) strains. [Ca2+]c, the free Ca2+ concentration in the cytosol. (b and c) the comparison of [Ca2+]c of WT and CaMmut(1,2,4) in dynamic and resting levels. The peak value is the peak level after the extracellular calcium stimulus. The basal level is the resting level prior to the extracellular calcium stimulus. Data are the average of three experiments. Error bars show the standard deviation. Statistical significance was determined by Student’s t-test.](/cms/asset/57c6e23e-4a6e-466b-9311-f075be2a10a5/kvir_a_2290757_f0006_oc.jpg)
Ca2+-binding to CaM deficiency causes attenuated virulence in A. fumigatus
Given that mutations in Ca2+-binding sites of CaM resulted in defective phenotypes that are relevant to pathogenesis, such as reduced hyphal growth and disordered cytoplasmic calcium homeostasis, we wondered whether the loss of Ca2+-binding sites affects fungal virulence in A. fumigatus. To this end, we compared the virulence potential of the WT, CaMmut(1,2,4) and CaMmut(1,2,3,4) strains using a Galleria mellonella wax moth model. G. mellonella larvae were injected with conidia of the indicated strains and incubated at 37 °C for eight days. The reason for choosing these two mutants was that strains carrying the individual or double mutations did not show any detectable differences compared to WT at 37 °C, suggesting that they are not involved in fungal virulence. Simultaneous mutations in three or four residues resulted in seriously defective phenotypes in A. fumigatus. As shown in , CaMmut(1,2,4) exhibited lower mortality than WT, although statistical analysis showed no significant survival difference between WT and CaMmut(1,2,4). However, the survival curve analysis showed that the CaMmut(1,2,3,4) mutant was significantly less virulent than the parental WT (). The percentage of surviving larvae eight days after injection with the CaMmut(1,2,3,4) mutant was 75%. No mortality was observed in the PBS-injected group (). These data suggest that simultaneous mutation of the four Ca2+-binding sites causes loss of virulence of A. fumigatus in the G. mellonella insect model.
Figure 7. The CaM mutant exhibits attenuated virulence. (a and b) survival curves of G. mellonella larvae infected with WT, CaMmut(1,2,4), and CaMmut(1,2,3,4) strains. PBS-injected larvae were used as the negative control. Statistical differences between groups were determined using a log-rank test.
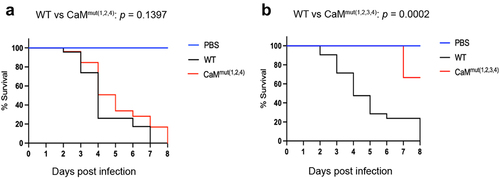
Discussion
CaM is a small, ubiquitous Ca2+-binding protein that regulates a wide variety of proteins and processes in eukaryotes [Citation38]. In this study, we characterized the role of an evolutionarily conserved CaM protein in the pathogenic fungus A. fumigatus. By establishing the conditional niiA-Afcam mutant, we demonstrated that turning off Afcam expression almost completely blocked hyphal growth and conidiation in A. fumigatus (). This suggests that AfCaM is important and might be essential, which is consistent with our previous findings in the model filamentous fungus A. nidulans [Citation40]. In fact, CaM from vertebrates and S. pombe (but not from budding yeast S. cerevisiae) can complement each other, indicating that they are relatively functionally conserved, but different species have varied characterization [Citation41–43]. Although bioinformatic analysis indicates that CaM contains four predicted Ca2+-binding motifs known as EF-hands that can bind one Ca2+ ion each [Citation1], in vivo examination of Ca2+-binding at individual sites has not been explored before this study in pathogenic fungi. Thus, for an essential gene, the conditional CaM mutants constructed in this study could be used to explore multiple distinct functions of AfCaM. Interestingly, strains expressing the individual CaM mutation did not affect hyphal growth and conidiation in A. fumigatus, whereas the double mutations exhibited slight growth defects. However, simultaneous mutations of three or four residues resulted in significantly decreased conidia production and colony diameter, suggesting that these four combined Ca2+-binding sites of CaM are essential for A. fumigatus growth and may have complementary and overlapping roles for Ca2+ binding. This finding is consistent with that of S. pombe [Citation6]. Notably, the simultaneous mutations of residues 1, 2, 3, and 4 in AfCaM resulted in nearly no growth (if any) of A. fumigatus colonies (). This is different from that in yeast, in which Ca2+-binding defective-CaM mutants only showed decreased growth [Citation10]. In addition, the intracellular localization of GFP-CaM was highly concentrated at the hyphal apex (), indicating that active growth was dependent on the proper trafficking of CaM at the hyphal tip, which explains why AfCaM is essential for colony growth. This localization pattern for AfCaM is also in line with a previous report on A. nidulans [Citation40]. Instead, four Ca2+ binding site mutations in AfCaM resulted in a diffuse distribution pattern. Nevertheless, in vitro biochemistry western blotting further showed that Ca2+-binding sites in AfCaM affected its protein stabilization. Collectively, these data clearly demonstrate that Ca2+-binding sites in AfCaM are required for the full functionality of this protein.
As successful environmental fungal pathogens, they must have a strong ability to adapt to various environmental stresses for survival. Accordingly, human pathogenic fungi should face a relative stress barrage in the environment and inside the host body, most notably the requirement for growth at in vivo temperatures. Previous studies on the human pathogens C. neoformans and Candida albicans revealed that the Ca2+/CaM-activated calcineurin-dependent pathway is required for adaptation to the stress response and that they are relatively conserved; however, the specific roles of CaM in these fungi have diverged [Citation21,Citation28]. The data in this study () showed that the Ca2+ binding mutations in AfCaM were hypersensitive to high temperatures, especially when residue 2 was mutated, suggesting that these mutated residues are required for the growth of fungal cells at high temperatures. Interestingly, the defective phenotypes of CaM mutants at 42 °C can be partially restored by high osmotic stress (Fig. S3). This indicates that osmotic pressure can compensate for the low extracellular calcium absorption caused by AfCaM Ca2+-binding site mutations to maintain calcium homeostasis.
It is well known that CaM plays a crucial role in regulating cellular responses to environmental changes, primarily by activating the CaM-dependent calcineurin-CrzA system, and Ca2+ is required for CaM activation [Citation44,Citation45]. The response of Aspergilli to elevated concentrations of extracellular calcium is mediated by CrzA [Citation13]. However, nucleocytoplasmic trafficking of CrzA did not occur in Ca2+-binding site mutations in CaM mutants (), indicating that these Ca2+-binding sites are required for the translocation of CrzA from the cytoplasm to the nucleus in A. fumigatus. Therefore, the hypersensitivity to high temperatures of AfCaM mutants might be due to nuclear mislocalization of CrzA induced by the absence of calcium binding of CaM. Moreover, in previous studies, multiple temperature-sensitive CaM mutants exhibited defects in actin organization, CaM localization, nuclear division, or bud formation [Citation46,Citation47], implying that loss of calcium binding in CaM could cause multiple cellular defects. Importantly, we also demonstrated that the four predicted Ca2+-binding sites of CaM are critical for virulence in the wax moth model, implying that the calcium-CaM signalling pathway plays a key role in fungal virulence.
In conclusion, the present study reveals the critical roles of calcium binding in AfCaM in regulating hyphal growth, subcellular localization, response to environmental stress, intracellular calcium homeostasis, and virulence in the human pathogen A. fumigatus. These findings can serve as a foundation for understanding CaM function in human pathogenic fungi.
Materials and methods
Strains and cultivation conditions
The A. fumigatus strains used in the present study are listed in the S1 Table. Strains were grown on MM containing 10 g/L glucose, 1 ml/L 1000 × trace elements (2.2 g/L ZnSO4·7 H2O, 1.1 g/L H3BO3, 0.5 g/L MnCl2·4 H2O, 0.5 g/L FeSO4·7 H2O, 0.16 g/L CoCl2·5 H2O, 0.16 g/L CuSO4, 0.11 g/L (NH4)6Mo7O24·4 H2O, 5 g/L EDTA, pH 6.5–6.8), 50 ml/L 20 × salt solution (60 g/L NaNO3, 5.2 g/L KCL, 15.2 g/L KH2PO4, 5.2 g/L MgSO4·7 H2O), and 20 g/L agar. MM was supplemented with 5 mM uridine and 10 mM uracil for the uridine and uracil auxotrophic strains. To induce and repress cam expression in the niiA-Afcam mutant, the medium was supplemented with a nitrogen source of NO3− or NH4+. All strains were cultured at 37 °C or 42 °C.
Construction of the conditional strain niiA-Afcam and the complementary strain Afcam-recon
The fusion PCR method was used to generate the indicated mutant strains as previously described [Citation48]. For niiA-Afcam mutant construction, the endogenous promoter of cam was replaced with a conditional nitrogen-inducible niiA promoter. Briefly, approximately 1 kb of the upstream and downstream flanking sequences of the cam promoter regions at positions −802 and + 1 were amplified with the primer pairs NA-P1/NA-P3 and NA-P4/NA-P6, respectively. Primers Pyr4-F/Pyr4-R and NA-F/NA-R were used to create the nutritional markers UU and niiA promoter fragments, respectively. These two fragments were fused using the primer pair Pyr4-F/NA-R to yield a cam deletion cassette. The three purified PCR products were used as templates to generate the niiA-Afcam cassette using primers NA-P1 and NA-P6. The resulting fusion product was cloned into the pEASY-Blunt Zero cloning kit (TransGen Biotech) and used to transform the WT recipient strain. Transformants were grown in media supplemented with 0.1 μg/ml pyrithiamine (Sigma) and verified by diagnostic PCR using the primer pairs NA-P1/F-Pyr4, R-Pyr4/NA-P6, and NA(S)/NA(A).
Reintroduction of a full-length cam gene into the niiA-Afcam mutant strain, including its native promoter, 5’-UTR, ORF, and 3’-UTR, allowing for genetic complementation. With the primers RE-F/RE-R, the cam gene fragment was amplified from WT A. fumigatus genomic DNA and then fused to a resistance marker, hygromycin B phosphotransferase (hyg), using the primers Hyg-F and Hyg-R. The hyg gene was amplified from the plasmid pAN7–1 using primers Hyg-F and Hyg-R. The resultant DNA fragment was fused with hyg and subsequently transferred to the conditional niiA-Afcam strain when cultured on repressing conditions to generate the complementary strain Afcam-recon.
Construction of the mutant niiA-AfcamAfcam-T
To construct the truncated strain lacking all four EF-loops of AfCaM, we used the WT A. fumigatus genomic DNA as the template to amplify two 1708 bp and 3468 bp DNA fragments with the primer pairs CaM-T-up/CaM-T1-R and CaM-T1-F/CaM-T-down, respectively. The two PCR products were fused to generate a 5176 bp DNA fragment lacking the first AfCaM EF-loop. Next, we used this 5176 bp DNA fragment as the template to amplify two 1780 bp and 3299 bp DNA fragments with the primer pairs CaM-T-up/CaM-T2-R and CaM-T2-F/CaM-T-down, respectively. We then fused these two PCR products to generate a 5079 bp DNA fragment lacking the first and second EF-loops of AfCaM. A similar strategy was used to construct strains by deleting all four EF loops. The resultant DNA fragment was subsequently transferred to the conditional niiA-Afcam strain to generate the truncated mutant niiA-AfcamAfcam-T strain.
Construction of the CaM point mutation strains
A cam mutant carrying a single mutation in the first EF-loop (E32A) was constructed as follows. The pyr4 selectable marker was amplified from plasmid pAL5 with the primer pair Pyr4-F/Pyr4-R. Next, the 5’ flanking sequence (1727 bp) and the 3’ flanking sequence (1653 bp) were amplified from the WT A. fumigatus genomic DNA using the primer pairs CaM-mutant-P1/CaM-mutant-P3 and CaM-mutant-P4/CaM-mutant-P6, respectively. The three PCR products were fused to generate a 5502 bp DNA fragment with the primers CaM-mutant-P2/CaM-mutant-P5, which was then cloned into the vector Blunt-Zero to generate the plasmid Pfr01. The 5’ flanking sequence (970 bp) and 3’ flanking sequence (4542 bp) were amplified from plasmid Pfr01 using the primer pairs CaM-mutant-P2/CaM(1)-DOWN and CaM(1)-UP/CaM-mutant-P5, respectively, and the complementary regions contained the desired mutation (glutamate32–alanine32). Using these two fragments as templates, the final 5502 bp fragment was generated by fusion PCR using primers CaM-mutant-P2 and CaM-mutant-P5, which was further cloned into the vector Blunt-Zero to generate plasmid Pfr02. We generated CaMmut(1) by transforming Pfr02 into the WT strain. A similar strategy was used to construct other CaM point mutation strains.
Construction of the recombinant GFP-CaM strains
To express the N-terminal of the recombinant niiA-AfcamGFP-CaM, we first amplified a 752-bp GFP fragment from plasmid pFNO3 with the primer pair GFP-F/GFP-R. The 5’ flanking sequence (1415 bp) and 3’ flanking sequence (3916 bp) were amplified from the above revertant plasmid using the primer pairs CaM-Q-F/CaM-Q-R and CaM-F/hyg(R), respectively. The three PCR products were fused using primers CaM-P2/hyg(R)-P2 to generate a fusion fragment (5783 bp), which was then transformed into the conditional strain niiA-Afcam to generate niiA-AfcamGFP-CaM. To construct a cam mutant containing four residue-mutated sites with GFP, the mutated cam ORF was amplified from the CaMmut(1,2,3,4) plasmid with the primers CaM-F/CaM-R. The purified PCR product was fused to the 3’-flanking sequence that was amplified from a revertant plasmid using the primers hyg(F)/hyg(R). The resulting fragments were fused to generate the niiA-AfcamGFP-CaM(1,2,3,4) mutant following the same steps as niiA-AfcamGFP-CaM.
Construction of the CrzA-GFP strains
To express the C-terminus of CrzA-GFP strains, the GFP-hyg fragment was first amplified from plasmid pFNO3 using the primers gfp+hyg-UP/gfp+hyg-DOWN. The 5’ flanking sequence (1619 bp) and 3’ flanking sequence (1751 bp) were then amplified from the WT A. fumigatus genomic DNA with the primer pairs CRZ-GFP-P1/CRZ-GFP-P3 and CRZ-GFP-P4/CRZ-GFP-P6, respectively. The three PCR products were fused with the primers CRZ-GFP-P2/CRZ-GFP-P5, and then the purified PCR products were transformed into WT, CaMmut(1,2,4) and CaMmut(1,3,4) strains, respectively.
Construction of the aequorin-expressing strains
To generate aequorin-expressing mutants, the plasmid pAEQS1–15 containing codon-optimized aequorin and the selective marker hyg were co-transformed into the indicated mutants. Transformants were screened for aequorin expression using described previously [Citation49].
Semi-quantitative PCR and RT-qPCR
Fresh A. fumigatus conidia were grown in liquid MM in a rotary shaker at 220 rpm at 37 °C for 24 h. Total RNA was extracted with the UNlQ-10 Column TRIzol total RNA isolation kit (Sangon Biotech, B511361) according to the manufacturer’s directions. The HiScript II Q RT SuperMix for qPCR kit (Vazyme, R223–01) was used to synthesize cDNA. For semi-quantitative PCR, with the primers Cam-up/Cam-down and Cam-T-up/Cam-T-down, the cam gene fragments were amplified from WT and niiA-AfcamAfcam-T A. fumigatus cDNA and genomic DNA, respectively. For RT-qPCR, independent assays were performed with three replicates, and transcript levels were calculated by the comparative threshold cycle (ΔCT) and normalized against the mRNA expression of cam in A. fumigatus. The 2−ΔΔCT method was used to determine the changes in mRNA expression. All the RT-qPCR primers are given in Table S2.
Fluorescence microscopy
A total of 1 × 106 conidia of the GFP-CaM strain were cultured on glass coverslips (Sangon Biotech) for cell culture in 1 mL liquid MM for 12 h to visualize the localization of the GFP-CaM fusion protein. The medium was removed and the samples were washed thrice with phosphate-buffered saline (PBS). The samples were fixed for 30 min at room temperature with 4% (v/v) formaldehyde and washed three times with PBS. After that, the nuclear dye 4,’ 6-diamidino-2-phenylindole (DAPI) dissolved in PBS was used at a final concentration of 1 μg/mL and incubated for 30 min at room temperature. DAPI solution was then removed and the glass coverslip was washed three times with PBS. A Zeiss Axio Imager A1 microscope (Carl Zeiss) was used to collect all images.
[Ca2+]c measurement
The strains Aeq-WT and Aeq-CaMmut(1,2,4) were grown on MM for 2.5 days to form fresh conidiation. Equal amounts of conidia in 100 μL of liquid media were distributed to each well of a 96-well microtiter plate. Six wells were used in parallel for each treatment. After the incubation at 37 °C for 18 h, the medium was removed from each well and washed twice with PGM (20 mM PIPES (pH 6.7), 1 mM MgCl2, 50 mM glucose). For aequorin reconstruction, we incubated mycelia in 100 μL PGM containing 2.5 μM coelenterazine for 4 h at 4 °C in the dark. After reconstruction, the mycelia were washed twice with PGM, and the plate was incubated at room temperature for 1 h. To chelate extracellular Ca2+, 1 mM EGTA was added to each well 10 min before stimulus injection. At the end of each experiment, active aequorin was removed by permeabilizing the cells with 20% ethanol in the presence of 3 M CaCl2 to examine the total aequorin luminescence of each well. Luminescence was measured using an LB 96P Microlumat Luminometer (Berthold Technologies). The relative light unit [RLU] values were converted into [Ca2+]c concentrations using the calibration formula: pCa = 0.332588 (−log k) +5.5593, where k is luminescence (RLU) s−1/total luminescence (in RLU) [Citation39].
Western blotting analysis
To extract proteins from A. fumigatus mycelia, conidia from related strains were incubated in liquid-inducing medium and then shaken at 220 rpm on a rotary shaker at 37 °C for 48 h. The mycelium was ground in liquid nitrogen using a mortar and pestle and suspended in ice-cold extraction buffer (50 mM HEPES (pH 7.4), 137 mM KCl, 10% glycerol, 1 mM EDTA, 1 μg/mL pepstatin A, 1 μg/mL leupeptin, and 1 mM PMSF). Equal amounts of proteins (40 μg) per lane were subjected to 10% SDS – PAGE and transferred to PVDF membranes (Immobilon-P, Millipore) in 384 mM glycine, 50 mM Tris (pH 8.4), and 20% methanol at 250 mA for 1.5 h, the membranes were blocked with PBS, 5% milk, and 0.1% Tween 20. Next, the membrane was probed sequentially with 1:3000 dilutions of anti-GFP primary antibody (Sigma) and goat anti-rabbit IgG-horseradish peroxidase secondary antibody (Abclonal, AS014) diluted in PBS, 5% milk, and 0.1% Tween 20. Blots were developed using Clarity ECL Western blotting detection reagents (Bio-Rad), and images were acquired with a Tanon 4200 Chemiluminescence Imaging System.
Virulence assay
G. mellonella larvae were purchased from Tianjin Huiyude Biotechnology Co., Ltd. In the G. mellonella model, 10 μL of the standardized conidia suspension in indicated A. fumigatus strains were injected into G. mellonella larvae (approximately 0.3 g) via the left prolegs. As a control group, the larvae were injected with PBS. All the larvae were incubated for up to 8 days at 37 °C with humidity levels around 29-33 %, and their survival was evaluated every 24 h. The procedure was conducted three times with groups of 20 larvae per sample.
Author contributions
Conceptualization, Y.Z. and L.L.; methodology, X.L. and R.F.; validation, X.L.; formal analysis, X.L. and R.F.; investigation, X.L., R.F., and P.L.; resources, L.L.; data curation, X.L., R.F., and P.L.; writing – original draft preparation, X.L. and R.F.; writing – review and editing, Y.Z. and L.L.; visualization, X.L. and R.F.; supervision, L.L.; project administration, L.L.; funding acquisition, L.L. All authors have read and agreed to the published version of the manuscript.
Fig. S3.pdf
Download PDF (890.9 KB)Table S1.docx
Download MS Word (17.1 KB)Fig. S1.pdf
Download PDF (47.4 KB)Table S2.docx
Download MS Word (19.5 KB)Fig. S4.pdf
Download PDF (477.2 KB)Figure S2-revised.pdf
Download PDF (194.2 KB)Acknowledgements
This work was financially supported by the National Natural Science Foundation of China (NSFC) (grants 82172292 and 31861133014 to L.L.), and the National Key R&D Program of China (2019YFA0904900), and the Priority Academic Program Development (PAPD) of Jiangsu Higher Education Institutions.
Disclosure statement
No potential conflict of interest was reported by the author(s).
Data Availability statement
The authors confirm that data supporting the findings of this study are available within the article.
Supplementary material
Supplemental data for this article can be accessed online at https://doi.org/10.1080/21505594.2023.2290757
Additional information
Funding
References
- Islam MS. Calcium signaling: from basic to bedside. Calcium Signaling. 2020;1131:1–15.
- Rosendo-Pineda MJ, Moreno CM, Vaca L. Role of ion channels during cell division. Cell Calcium. 2020;91:102258. doi: 10.1016/j.ceca.2020.102258
- Carafoli E. Intracellular calcium homeostasis. Annual review of biochemistry. Annu Revi Biochem. 1987;56(1):395–433. doi: 10.1146/annurev.bi.56.070187.002143
- Cyert MS, Philpott CC. Regulation of cation balance in Saccharomyces cerevisiae. Genetics. 2013;193(3):677–713. doi: 10.1534/genetics.112.147207
- LUAN Y, MATSUURA I, YAZAWA M, et al. Yeast calmodulin: structural and functional differences compared with vertebrate calmodulin. J Biochem. 1987;102(6):1531–1537.
- Moser MJ, Lee SY, Klevit RE, et al. Ca2+ binding to calmodulin and its role in schizosaccharomyces pombe as revealed by mutagenesis and NMR spectroscopy (*). J Biol Chem. 1995;270(35):20643–20652.
- Park H-S, Lee SC, Cardenas ME, et al. Calcium-calmodulin-calcineurin signaling: a globally conserved virulence cascade in eukaryotic microbial pathogens. Cell Host Microbe. 2019;26(4):453–462.
- Chin D, Means AR. Calmodulin: a prototypical calcium sensor. Trends in cell biology. Trends Cell Biol. 2000;10(8):322–328. doi: 10.1016/S0962-8924(00)01800-6
- Davis T N, Thorner J. Vertebrate and yeast calmodulin, despite significant sequence divergence, are functionally interchangeable. Proc. Natl. Acad. Sci. U.S.A. 1989;86(20):7909–7913. doi: 10.1073/pnas.86.20.7909
- Geiser JR, van Tuinen D, Brockerhoff SE, et al. Can calmodulin function without binding calcium? Cell. 1991;65(6):949–959.
- Flory MR, Morphew M, Joseph JD, et al. Pcp1p, an Spc110p-related calmodulin target at the centrosome of the fission yeast schizosaccharomyces pombe. Cell Growth Differ. 2002;13(2):47–58.
- Thewes S. Calcineurin-Crz1 signaling in lower eukaryotes. Eukaryotic Cell. 2014;13(6):694–705. doi: 10.1128/EC.00038-14
- Cramer RA Jr, Perfect BZ, Pinchai N, et al. Calcineurin target CrzA regulates conidial germination, hyphal growth, and pathogenesis of Aspergillus fumigatus. Eukaryot Cell. 2008;7(7):1085–1097.
- Yang Y, Xie PD, Li YC, et al. Updating insights into the regulatory mechanisms of calcineurin-activated transcription factor Crz1 in pathogenic fungi. J Fungi (Basel). 2022;8(10):1082.
- Gupta S, Kumar A, Tamuli R. CRZ1 transcription factor is involved in cell survival, stress tolerance, and virulence in fungi. J Biosci. 2022;47(4). doi: 10.1007/s12038-022-00294-3
- Yang Y, Xie P, Yuan J, et al. The calcineurin-responsive transcription factor Crz1 is required for regulation of infection structure differentiation, calcium homeostasis and cell wall integrity in Alternaria alternata. Postharvest Biol Technol. 2022;194:194. doi: 10.1016/j.postharvbio.2022.112064
- Jiang Q, Mao R, Li Y, et al. AaCaM is required for infection structure differentiation and secondary metabolites in pear fungal pathogen Alternaria alternata. J Appl Microbiol. 2022;133(4):2631–2641.
- Martin N, Bernard D. Calcium signaling and cellular senescence. Cell Calcium. 2018;70:16–23. doi: 10.1016/j.ceca.2017.04.001
- Brown GD, Denning DW, Gow NAR, et al. Hidden killers: human fungal infections. Science translational medicine. Sci, trans med. 2012;4(165):165rv13–165rv13.
- Brown NA, Goldman GH. The contribution of Aspergillus fumigatus stress responses to virulence and antifungal resistance. J Microbiol. 2016;54(3):243–253. doi: 10.1007/s12275-016-5510-4
- Kraus PR, Heitman J. Coping with stress: calmodulin and calcineurin in model and pathogenic fungi. Biochem Biophys Res Commun. 2003;311(4):1151–1157. doi: 10.1016/S0006-291X(03)01528-6
- Juvvadi PR, Lee SC, Heitman J, et al. Calcineurin in fungal virulence and drug resistance: prospects for harnessing targeted inhibition of calcineurin for an antifungal therapeutic approach. Virulence. 2017;8(2):186–197.
- Sánchez-Piris M, Posas F, Alemany V, et al. The serine/threonine kinase Cmk2 is required for oxidative stress response in fission yeast. J Biol Chem. 2002;277(20):17722–17727.
- Juvvadi PR, Kuroki Y, Arioka M, et al. Functional analysis of the calcineurin-encoding gene cnaA from Aspergillus oryzae: evidence for its putative role in stress adaptation. Arch Microbiol. 2003;179(6):416–422.
- Warwar V, Oved S, Dickman MB. Antisense expression of the calmodulin gene from Colletotrichum trifolii impairs prepenetration development. FEMS Microbiol Lett. 2000;191(2):213–219. doi: 10.1111/j.1574-6968.2000.tb09342.x
- Lee S-C, Lee Y-H. Calcium/calmodulin-dependent signaling for appressorium formation in the plant pathogenic fungus Magnaporthe grisea. Molecules & Cells (Springer Science & Business Media BV). 1998;8(6):698–704.
- de Carvalho MJA, Amorim Jesuino RS, Daher BS, et al. Functional and genetic characterization of calmodulin from the dimorphic and pathogenic fungus paracoccidioides brasiliensis. Fungal Genet Biol. 2003;39(3):204–210.
- Kraus PR, Nichols CB, Heitman J. Calcium- and calcineurin-independent roles for calmodulin in Cryptococcus neoformans morphogenesis and high-temperature growth. Eukaryot Cell. 2005;4(6):1079–1087. doi: 10.1128/EC.4.6.1079-1087.2005
- O’Gorman CM. Airborne Aspergillus fumigatus conidia: a risk factor for aspergillosis. Fungal Biol Rev. 2011;25(3):151–157. doi: 10.1016/j.fbr.2011.07.002
- Arastehfar A, Carvalho A, Houbraken J, et al. Aspergillus fumigatus and aspergillosis: from basics to clinics. studies in mycology. Stud Mycol. 2021;100(1):100115–100115.
- Balloy V, Chignard M. The innate immune response to Aspergillus fumigatus. Microbes Infect. 2009;11(12):919–927. doi: 10.1016/j.micinf.2009.07.002
- Montoya JG, Giraldo LF, Efron B, et al. Infectious complications among 620 consecutive heart transplant patients at Stanford University Medical Center. clinical infectious diseases. Clinl Infect Dis. 2001;33(5):629–640.
- Howard SJ, Arendrup MC. Acquired antifungal drug resistance in Aspergillus fumigatus: epidemiology and detection. Med Mycol. 2011;49(Supplement_1):S90–S95. doi: 10.3109/13693786.2010.508469
- Gifford JL, Walsh MP, Vogel HJ. Structures and metal-ion-binding properties of the Ca2±binding helix–loop–helix EF-hand motifs. Biochem J. 2007;405(2):199–221. doi: 10.1042/BJ20070255
- Moncrief ND, Kretsinger RH, Goodman M. Evolution of EF-hand calcium-modulated proteins. I. Relationships based on amino acid sequences. J Mol Evol. 1990;30(6):522–562. doi: 10.1007/BF02101108
- Kuboniwa H, Tjandra N, Grzesiek S, et al. Solution structure of calcium-free calmodulin. Nat Struct Biol. 1995;2(9):768–776.
- Zhang M, Tanaka T, Ikura M. Calcium-induced conformational transition revealed by the solution structure of apo calmodulin. Nat Struct Biol. 1995;2(9):758–767. doi: 10.1038/nsb0995-758
- Cyert MS. Genetic analysis of calmodulin and its targets in Saccharomyces cerevisiae. annual review of genetics. Annu Rev Genet. 2001;35(1):647–672. doi: 10.1146/annurev.genet.35.102401.091302
- Nelson G, Kozlova‐Zwinderman O, Collis AJ, et al. Calcium measurement in living filamentous fungi expressing codon‐optimized aequorin. Mol Microbiol. 2004;52(5):1437–1450.
- Chen S, Song Y, Cao J, et al. Localization and function of calmodulin in live-cells of Aspergillus nidulans. Fungal Genet Biol. 2010;47(3):268–278.
- Davis TN, Thorner J, Vertebrate and yeast calmodulin, despite significant sequence divergence, are functionally interchangeable. Proceedings of the National Academy of Sciences, 1989. 86 (20): p. 7909–7913.
- Harris E, Watterson DM, Thorner J. Functional consequences in yeast of single-residue alterations in a consensus calmodulin. J Cell Sci. 1994;107(11):3235–3249. doi: 10.1242/jcs.107.11.3235
- Takeda T, Yamamoto M, Analysis and in vivo disruption of the gene coding for calmodulin in schizosaccharomyces pombe. Proceedings of the National Academy of Sciences, 1987. 84 (11): p. 3580–3584.
- Rasmussen C, Garen C, Brining S, et al. The calmodulin‐dependent protein phosphatase catalytic subunit (calcineurin A) is an essential gene in Aspergillus nidulans. EMBO J. 1994;13(16):3917–3924.
- Dayton JS, Means AR. Ca (2+)/calmodulin-dependent kinase is essential for both growth and nuclear division in Aspergillus nidulans. molecular biology of the cell. ?Mol Biol Cell. 1996;7(10):1511–1519. doi: 10.1091/mbc.7.10.1511
- Ohya Y, Botstein D. Diverse essential functions revealed by complementing yeast calmodulin mutants. Science. 1994;263(5149):963–966. doi: 10.1126/science.8310294
- Peng CA, Altamirano S, Paladugu N, et al. Role of the anillin-like protein in growth of Cryptococcus neoformans at human host temperature. Fungal Genet Biol. 2022;160:103697. doi: 10.1016/j.fgb.2022.103697
- Szewczyk E, Nayak T, Oakley CE, et al. Fusion PCR and gene targeting in Aspergillus nidulans. Nat Protoc. 2006;1(6):3111–3120.
- Zhang Y, Zheng Q, Sun C, et al. Palmitoylation of the cysteine residue in the DHHC motif of a palmitoyl transferase mediates Ca2+ homeostasis in Aspergillus. PLoS Genet. 2016;12(4):e1005977.