ABSTRACT
Enterohemorrhagic Escherichia coli (EHEC) is an important zoonotic pathogen that is a major cause of foodborne diseases in most developed and developing countries and can cause uncomplicated diarrhoea, haemorrhagic colitis, and haemolytic uraemic syndrome. O islands (OIs), which are unique genomic islands in EHEC O157:H7, are composed of 177 isolated genomic features and harbour 26% of the total genes that are absent in the non-pathogenic E. coli K-12 genome. In the last twenty years, many OI-encoded proteins have been characterized, including proteins regulating virulence, motility, and acid resistance. Given the critical role of regulatory proteins in the systematic and hierarchical regulation of bacterial biological processes, this review summarizes the OI-encoded regulatory proteins in EHEC O157:H7 characterized to date, emphasizing OI-encoded regulatory proteins for bacterial virulence, motility, and acid resistance. This summary will be significant for further exploration and understanding of the virulence and pathogenesis of EHEC O157:H7.
Introduction
Enterohemorrhagic Escherichia coli (EHEC) O157:H7 causes a wide range of diseases, among which uncomplicated diarrhoea, haemorrhagic colitis, and haemolytic uraemic syndrome are common clinical features [Citation1]. Adult cattle and other farm animals are the main reservoirs of EHEC O157:H7 [Citation2], and humans can be infected through contaminated food or water [Citation3]. Many outbreaks of EHEC O157:H7 infection have been associated with contaminated beef products, milk, vegetables, and fruits [Citation4,Citation5]. Notably, the infectious dose of EHEC O157:H7 is extremely low (approximately 10–100 cells), leading to an increased occurrence of such infections [Citation6]. The pathogenesis of EHEC O157:H7 is characterized by the formation of attachment and elimination (A/E) lesions, involving the effacement of intestinal microvilli, the intimate attachment of bacteria to host intestinal cell membranes, the accumulation of polymeric actin and other cytoskeletal components, and pedestal structure formation beneath the attached bacteria [Citation3].
The ability of EHEC O157:H7 to form A/E lesions is attributed to the locus of enterocyte effacement (LEE), which is a large pathogenicity island consisting of five polycistronic operons (LEE1–LEE5) [Citation7]. Genes encoding a type III secretion system (T3SS), the adhesion intimin and its receptor Tir, and effector proteins are present within the LEE operons, and the encoded proteins are needed for effector molecule export, intimate adherence to host epithelial cells, translocation, and modification of host cell signal transduction during the formation of A/E lesions [Citation8]. Deletion of LEE leads to complete abolishment of cellular adhesion and A/E lesion formation by EHEC O157:H7 [Citation9]. In addition to the LEE pathogenicity island, the genomic islands termed O islands (OIs), which are not retained in the non-pathogenic E. coli K-12 strain MG1655, are harboured in the EHEC O157:H7 genome [Citation10]. The prevalence of OIs varies among different EHEC O157:H7 strain clades (clades 1–9), among which the isolate EHEC O157:H7 strain EDL933, belonging to clade 9, contains the entire set (n = 177) of OIs [Citation11]. A total of 1387 genes (26% of the total) accumulate in these OIs, most of which encode hypothetical proteins with unclear functions, together with newly identified sRNAs [Citation10,Citation11].
In recent years, great efforts have been made to understand the functions of the proteins encoded by OIs, including the identification of key virulence factors (adhesins and Shiga toxins), effectors, virulence regulatory proteins and sRNAs, and stress adaptation factors [Citation12]. In this review, to gain a comprehensive understanding of the regulatory functions of OIs, we summarize the currently characterized OI-associated genes that encode proteins that function in virulence, motility, and acid resistance regulation in EHEC O157:H7. Furthermore, in this review, we suggest future research directions and propose possible methodologies for the in-depth functional characterization of OI-associated genes.
OI-encoded virulence regulatory proteins
During evolution, EHEC O157:H7 has acquired an elaborate regulatory network for virulence and pathogenicity, in which regulatory proteins are pivotal for coordinating virulence-related gene expression through direct or indirect gene activation or repression [Citation13]. Elaborate regulation allows bacteria to precisely control virulence gene expression under relevant environmental conditions with no metabolic costs and without alerting the host immune system [Citation14,Citation15]. In total, 16 OI-encoded virulence regulatory proteins (10 activators and 6 repressors) with distinct DNA-binding domains have been characterized in EHEC O157:H7, namely, the activators Ler (encoded by the LEE pathogenic island (PAI) OI-148), OvrB (encoded by OI-9), OvrA (encoded by OI-19), Cro (encoded by OI-45), GrvA (encoded by OI-47), RgdR (encoded by OI-51), EtrB (encoded by OI-115), LmiA (encoded by OI-119), AirA (encoded by OI-134), the activator-repressor system GrlA-GrlR (encoded by OI-148), and the repressors PatE (encoded by OI-8), PsrA (encoded by OI-50), PsrB (encoded by OI-57), EtrA (encoded by OI-115), and EivF (encoded by OI-115) ( and ).
Figure 1. Circle plot showing the locations of OIs and OI genes encoding regulatory proteins in the EHEC O157:H7 EDL933 genome. from the outside in, circle 1 represents the size of the base pairs, circle 2 shows the positions of the OI genes encoding regulatory proteins mentioned in this review, and circle 3 shows the positions of the corresponding OIs.
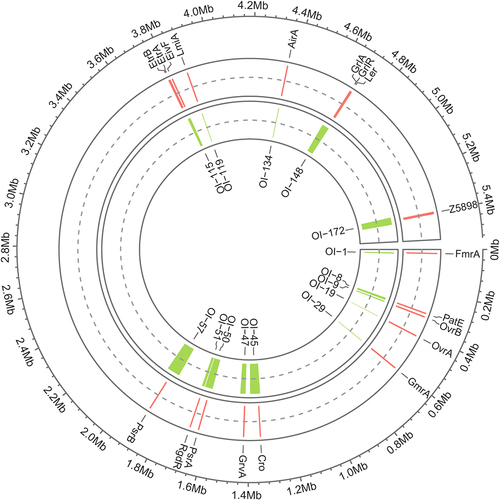
Figure 2. Pie chart showing the functional classification of OI-encoded regulatory proteins. each pie slice represents a major functional group of regulatory proteins. The values represent the number of regulatory proteins in a particular category.
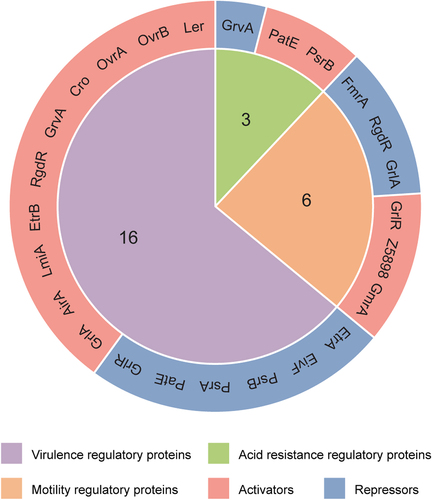
Figure 3. Domain structure of OI-encoded regulatory proteins. conserved domains were predicted using the InterPro protein families and domains database (https://www.Ebi.ac.uk/interpro). The numbers indicate the amino acid positions relative to the N-terminal end.
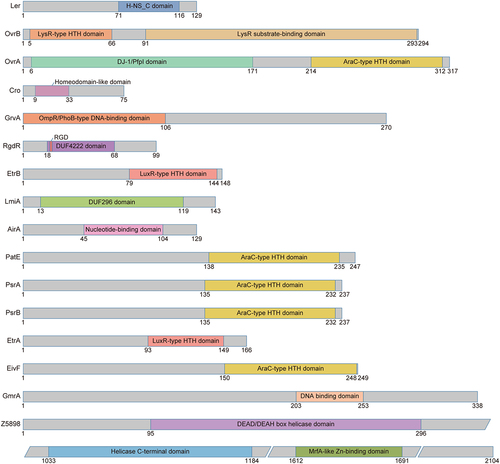
Figure 4. Regulation of virulence gene expression by OI-encoded regulatory proteins in EHEC O157:H7. OI-encoded regulatory proteins, including ler, OvrB, OvrA, Cro, GrvA, RgdR, EtrB, LmiA, AirA, GrlA, GrlR, PatE, PsrA, PsrB, EtrA, and EivF, affect EHEC O157:H7 virulence and LEE gene expression.
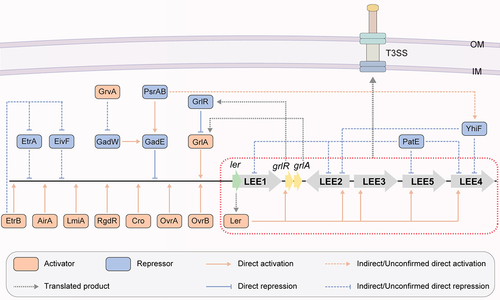
Table 1. OI-encoded regulatory proteins in EHEC O157:H7.
Ler (OI-148)
Ler, encoded by the first gene (z5140) of the LEE1 operon in LEE PAI OI-148 of the EHEC O157:H7 EDL933 genome (), is the master activator of LEE operons (LEE2 to LEE5) in EHEC O157:H7 [Citation14,Citation16]. Ler contains an H-NS C-terminal domain and is a member of the H-NS protein family (), which is a family of transcriptional regulators with high affinity for AT-rich DNA sequences that have been best characterized in E. coli and Salmonella. H-NS proteins often mediate bacterial environmental adaptation and pathogenicity through transcriptional repression and maintenance of gene silencing [Citation40]. Ler activates virulence-related genes by antagonizing H-NS repression, and the unique DNA-binding activity of Ler during this process is conferred by arginine in its side chain [Citation40]. A range of global and specific regulatory proteins outside LEE contribute to the control of LEE gene expression, mostly in a Ler-dependent manner [Citation7], including the regulatory proteins OvrB, OvrA, Cro, RgdR, EtrB, LmiA, AirA, and GrlA () [Citation11]. Therefore, Ler is a central regulator of EHEC O157:H7 virulence and participates in a complex regulatory network that regulates LEE genes at the transcriptional and post-transcriptional levels [Citation7]. Notably, LEE PAIs with similar sequences are generally conserved among various enterobacterial pathogens, including enteropathogenic E. coli (EPEC) and EHEC. However, the function of LEE PAIs in EHEC requires the coordination of additional environmental or regulatory factors compared to those in EPEC [Citation41,Citation42], underscoring the complexity of the regulatory role of Ler in bacterial virulence.
OvrB (OI-9)
O island-encoded virulence regulator B (OvrB) is encoded by the gene z0346 located in OI-9 of the EHEC O157:H7 EDL933 genome (). The ovrB-encoding protein is predicted to be a putative transcriptional regulator that contains an N-terminal helix-turn-helix (HTH) DNA-binding domain and a C-terminal coinducer-binding domain, which is a classic conserved LysR-type transcriptional regulator (LTTR) family domain () [Citation43]. Recently, we demonstrated that OvrB is needed for bacterial adherence to host cells and for LEE gene expression regulation in EHEC O157:H7. Upon ovrB deletion, significantly reduced bacterial adherence was observed in both HeLa and Caco-2 cells, as was the downregulation of LEE genes and intestinal colonization in a mouse model. We also detected the direct binding signal of OvrB at the promoter region of LEE1, which activated ler transcription and in turn promoted the expression of the LEE2-LEE5 genes (). Similar virulence regulatory functions of orthologous ovrB genes have also been characterized in the EPEC O55:H7 and EHEC O145:H28 strains, indicating that OvrB is a ubiquitous LEE activator in different E. coli pathotypes [Citation17]. Finally, we also found that OvrB plays no role in bacterial motility regulation [Citation17], similar to other LTTR family factors [Citation44]. However, whether OvrB plays regulatory roles in other biological processes remains unclear.
OvrA (OI-19)
OI-encoded virulence regulator A (OvrA) is encoded by z0442 in OI-19 of the EHEC O157:H7 EDL933 genome (). OI-19 contains two ORFs, z0442 (ovrA) and z0443, which encode a putative transcription factor and an unidentified protein, respectively. OvrA contains a C-terminal AraC-type HTH DNA-binding domain () and was recently demonstrated to be involved in virulence regulation in EHEC O157:H7 by our group. OvrA positively regulates EHEC O157:H7 adherence and intestinal colonization through LEE gene expression activation. Mechanistically, OvrA directly binds the motif 5'-GACATTTAATGATAATG-3' at the LEE1 promoter to activate the expression of ler, which in turn activates the expression of all LEE operon genes () [Citation18]. Moreover, ovrA orthologs in the EHEC O145:H28 and O157:H16 strains and in the EPEC O55:H7 strain were also shown to play positive regulatory roles in bacterial adherence and LEE gene expression [Citation18], indicating that OvrA-mediated virulence promotion is a general mechanism that is conserved among other non-O157 pathogenic E. coli strains.
Cro (OI-45)
The phage transcription factor Cro is encoded by cro (z1448), which is located at OI-45 in the EHEC O157:H7 EDL933 genome (). Cro contains a Homeodomain-like domain () and was demonstrated to activate the T3SS in EHEC O157:H7 [Citation19]. Deletion of cro in the EHEC O157:H7 strain 86–24 led to decreased LEE gene expression (including espA, espB, espD, and espF) and A/E lesion formation. Mechanistically, Cro from the EHEC O157:H7 strain 86–24 (Cro-86–24) directly binds the LEE1 promoter to activate the expression of ler, which in turn activates the expression of other LEE genes (). Additionally, infecting mice with the related pathogen C. rodentium harbouring the cro from the EHEC O157:H7 strain 86–24 resulted in greater LEE gene expression and enhanced virulence. Interestingly, the amino acid sequence of Cro varies among phages carried by different EHEC outbreak strains, and these changes affect Cro-dependent T3SS regulation [Citation19,Citation45]. Cro from the EHEC O157:H7 strain EDL933 (Cro-EDL933) was able to bind to the ler promoter, whereas Cro from the EHEC O157:H7 strain Sakai (Cro-Sakai) was not able to bind to this promoter. Consistent with the EMSA results, Cro-EDL933 but not Cro-Sakai was able to restore the expression of ler in the EHEC 86–24 Δcro mutant. These results indicated that Cro-EDL933 can function as a transcriptional activator of LEE genes in a similar way to Cro-86–24. Although the regulatory effects of Cro on virulence factors differ across EHEC strains, the underlying mechanisms remain unclear.
GrvA (OI-47)
The global regulator of virulence A (GrvA) is encoded by grvA (z1531), which is located in OI-47 of the EHEC O157:H7 EDL933 genome () [Citation11]. GrvA contains an N-terminal OmpR/PhoB-type DNA-binding domain and shares homology with ToxR family transcriptional regulatory proteins (), such as MarT in Salmonella enterica, with 30% amino acid identity, and CadC in E. coli, with 13% amino acid identity [Citation46,Citation47]. Increased transcription from the ler promoter and induced adherence to Caco-2 cells were detected in grvA-overexpressing cells [Citation20]. Mechanistically, analysis of the transcription profile showed that ΔgrvA in the EHEC O157:H7 mutant strain TW14359 downregulated twenty-one LEE genes, including the type III secretion translocon genes espA, espB, espD, and eae (encoding intimin) and the secreted effector-encoding gene tir [Citation39]. GrvA-mediated LEE activation requires RcsB, which is an Rcs phosphorelay response regulator that is sensitive to physiologically relevant concentrations of bicarbonate [Citation20,Citation48]. In addition, GrvA-dependent regulation of LEE requires GadE, a known direct repressor of ler. In the regulatory cascade, GrvA indirectly represses gadE transcription from the P3 promoter during exponential phase growth, during which the GadE activator GadW is needed () [Citation39]. Bioinformatics analysis revealed that OI-47 and grvA are highly conserved and exclusively distributed in EHEC O157:H7 [Citation11] and the progenitor EPEC O55:H7 [Citation49], together with their reported existence in EHEC O145:H28 [Citation50], suggesting a pathotype-specific regulatory role of GrvA in regulating virulence in pathogenic E. coli.
RgdR (OI-51)
RgdR is encoded by ECs1581 in the genome of the EHEC O157:H7 Sakai strain but was not annotated in the genome of the EDL933 strain until the recent mapping of rgdR to an identical intergenic region between z1841 and z1842 (genomic loci: 1682140–1682440) in OI-51 of the EHEC O157:H7 EDL933 genome () [Citation21]. RgdR, which contains a DUF4222 domain and an EHEC-conserved tripeptide arginine-glycine-aspartate (RGD) motif () [Citation22], has the capacity to activate the T3SS. Deletion of either the entire OI-51 or rgdR resulted in a significant reduction in the T3SS, which could be complemented by the in-trans expression of rgdR from a low-copy-number plasmid [Citation21], demonstrating the significance of RgdR in T3SS regulation. Mechanistically, RgdR activates the Ler-mediated autoregulatory cascade, thus promoting the expression of the whole LEE operon and facilitating type III secretion () [Citation21]. RgdR was also shown to be involved in regulating bacterial motility (see below for details) [Citation21].
Notably, OI-51 was present in the whole clade 9 EHEC O157:H7 strains and three strains of clade 5 (strains TB21–1, SS TX 754–1, and F8092B) [Citation11], and RgdR orthologs with high sequence similarities have been identified in many other E. coli serotypes; for example, the protein ECED1_1787 showed 94% sequence similarity in the UPEC strain ED1a [Citation51]. However, ECED1_1787 harbours an R20C mutation in the RGD motif and lacks T3SS activation activity, demonstrating the necessity of a conserved RGD motif for orthologous RgdR protein function [Citation21,Citation51]. Therefore, characterizing the conservation of the RGD motif in all EHEC O157:H7 clade strains is fundamental for understanding the functional conservation and diversity of RgdR.
EtrB (OI-115)
EtrB is encoded by etrB (z4176), which is located at OI-115 of the EHEC O157:H7 EDL933 genome (). OI-115 contains 21 open reading frames (ORFs) (from z4180 to z4200) harbouring the second cryptic T3SS-encoding genes, named the E. coli T3SS 2 (ETT2) genes [Citation11]. ETT2 closely resembles the T3SS in S. enterica and is encoded by Salmonella pathogenicity island 1 (Spi 1) [Citation52]. The function of ETT2 in E. coli virulence is controversial because the ETT2 cluster is fully or partially conserved in the majority of E. coli strains, especially K-12, irrespective of pathogenicity [Citation33]. ETT2 has been characterized to be functional in bacterial invasion, virulence, and intracellular survival, and several ETT2 cluster-located transcription regulators, including YgeH, YgeK/EtrB, EtrA, and EivF, have been identified [Citation34].
EtrB is homologous to SsrB in Salmonella [Citation33] and belongs to the NarL family of transcription factors that contain a C-terminal LuxR-type HTH DNA-binding domain () [Citation53]. Unlike etrA and eivF, etrB is not co-transcribed with its upstream or downstream genes but is expressed as a monocistronic transcript whose expression is autoregulated by EtrB and positively regulated by the LysR-type regulator QseA [Citation23,Citation24]. The ΔetrB isolate exhibited a significant reduction in the expression of ler, grlA, and the operons LEE2 to LEE5 (i.e. escC, escV, eae, and espA), as well as in pedestal formation [Citation24]. Furthermore, through microarray data collection and analysis, the same study showed that EtrB activated not only LEE genes but also non-LEE-encoded effectors, adhesins, and ETT2-located genes. Moreover, the mechanism by which EtrB activates LEE gene expression and A/E lesion formation was shown to involve direct interaction with the Ler regulatory region, as well as by repressing the expression of eivF and etrA () [Citation24]. However, the internal molecular mechanism by which eivF and etrA are regulated by EtrB remains unclear.
LmiA (OI-119)
Low magnesium-induced regulator A (LmiA) is encoded by z4267, which is located in OI-119 of the EHEC O157:H7 EDL933 genome () [Citation11]. LmiA contains a DUF296 domain (), which specifically binds AT-rich DNA sequences and has been detected in many transcriptional regulators. DNA sequence analysis revealed that the LmiA orthologs were highly conserved among different E. coli pathotype strains. Recently, we found that LmiA could enhance the adherence capacity of EHEC O157:H7 and activate LEE gene expression. The ΔlmiA mutant exhibited significantly reduced bacterial adherence and fluorescein actin staining, together with a decreased LEE gene expression compared to that in wild-type EHEC O157:H7 [Citation25]. The defects caused by lmiA deletion were restored after complementation with a low-copy-number plasmid harbouring lmiA in the ΔlmiA mutant. Furthermore, a 17-base pair motif (5'-TTAAAGTCGTTTGTTAA-3') within the LEE1 promoter region was characterized to be directly bound by LmiA under magnesium-depleted conditions, leading to ler activation and downstream activation of other LEE genes (). The signal sensing of LmiA under low-magnesium conditions in the virulence regulation process is mediated by the PhoQ/PhoP two-component system [Citation25,Citation54]. The LmiA-mediated virulence-regulating pathway is a highly conserved and widespread pathway that mediates bacterial virulence in a range of EHEC and EPEC strains. Furthermore, disruption of this regulatory pathway by deleting lmiA or phoPQ significantly decreased EHEC O157:H7 adherence in the mouse intestinal tract [Citation25], suggesting the potential significance of these genes as new therapeutic targets in the treatment of EHEC O157:H7 infection.
AirA (OI-134)
AirA, also known as adherence-inducible regulator A, is encoded by z4802 (airA), located at OI-134 of the EHEC O157:H7 EDL933 genome () [Citation26]. The airA gene is co-transcribed with four other ORFs located at OI-134, and the resulting gene product is derived from an ATP-dependent helicase RecQ truncation fragment that contains a predicted nucleotide-binding region (). Our group recently characterized AirA as a positive virulence regulatory protein in EHEC O157:H7. The ΔairA mutant reduced the adherence capability to cultured HeLa and Caco-2 cells, decreased A/E lesion formation and the intestinal colonization number in infant rabbits and mice, and decreased LEE gene expression at the transcriptional and translational levels. Mechanistically, AirA promotes initial adherence and colonization through ler activation by directly binding to a 17-bp sequence (5'-TGCAATGAGATCTATCT-3') located −302 to −286 from the proximal TSS region of ler, which in turn activates all LEE operon genes (). The expression of airA was induced by a mechanical cue generated from initial host adherence. Further investigation revealed that the outer membrane lipoprotein NlpE senses mechanical cues and activates the BaeS/BaeR two-component regulatory system, which in turn directly activates the expression of airA, leading to the activation of ler and other LEE genes. Finally, the AirA-mediated virulence-regulating pathway is specific to EHEC O157:H7 and is not widely distributed in other E. coli strains. Disruption of the AirA-mediated pathway by airA deletion severely attenuates EHEC O157:H7 virulence both in vitro and in vivo, indicating that airA could be a potential target for the development of new therapeutics for EHEC O157:H7 infection [Citation26].
GrlA and GrlR (OI-148)
Systematic analysis of LEE gene deletion mutants revealed that, in addition to Ler, the global regulators of LEE activator (GrlA) and LEE repressor (GrlR), encoded by z5128 and z5129, located at OI-148 of the EHEC O157:H7 EDL933 genome (), are involved in the transcriptional regulation of LEE [Citation55]. The ΔgrlA mutant exhibited drastically reduced expression levels of EspB and Tir, as well as a decreased LEE1 transcription level, indicating that GrlA is a positive regulator of LEE. In contrast, the ΔgrlR mutant exhibited an increased transcription level of LEE1; conversely, when grlR was introduced into a multicopy plasmid, repressed Tir and EspB expression levels were detected, indicating that GrlR acts as a negative LEE regulator. GrlA likely activates ler expression via direct binding to the suboptimal 18-base pair spacer between positions −10 and −35 in the distal LEE1 promoter via its HTH DNA-binding motif; subsequently, Ler activates the expression of other LEE genes [Citation27]. Conversely, GrlR antagonizes this system by binding directly to GrlA, preventing its interaction with the LEE1 promoter and hence leading to the transcriptional inhibition of LEE genes () [Citation28]. The ClpXP protease positively controls LEE gene expression through direct degradation of the repressor GrlR, thus freeing GrlA to activate the expression of ler and other LEE genes during the stationary growth phase [Citation56]. In addition to its effect on LEE gene expression, the GrlR/GrlA complex is involved in the regulation of other pathways determined for bacterial pathogenicity, such as flagella and enterohemolysin expression in EHEC O157:H7 (see below for details) [Citation36,Citation57].
Notably, a small number of GrlA homologs have been found in different bacterial species [Citation58], whereas GrlR orthologs with unassigned biological functions are ubiquitous in other bacterial species, such as Klebsiella and Salmonella species. GrlR was shown to play an alternative repressive role in LEE genes, independent of its interaction with the cognate partner GrlA in EPEC [Citation59], suggesting that additional regulatory mechanisms are involved in GrlR regulation. However, whether the repressive activity of GrlR depends on its interaction with DNA, RNA, or other proteins remains unclear.
PatE (OI-8)
PatE is encoded by z0321, which is located on OI-8 of the EHEC O157:H7 EDL933 genome (). As a member of the AraC/XylS family of regulators, PatE contains a characteristic C-terminal AraC-type HTH DNA-binding domain () [Citation60]. Microarray data analysis revealed that 18 genes were negatively regulated by PatE, including six LEE genes: espA, espB, espZ, tir, z5137 and z5138 [Citation29]. Therefore, PatE, which acts as a negative regulator, is involved in the transcriptional regulation of LEE genes (). However, the mechanism underlying the regulatory role of PatE in LEE gene expression remains unclear, and whether this regulatory protein mediates A/E formation, bacterial adherence, or other virulence-related biological processes remains unknown.
PsrA and PsrB (OI-50 and OI-57)
Prophage-encoded secretion regulator A (PsrA) is encoded by z1789, which is located on OI-50 of the EHEC O157:H7 EDL933 genome (). Prophage-encoded secretion regulator B (PsrB), encoded by z2104 in OI-57, shares 90% identity with PsrA and is the closest homolog of PsrA. Both psrA and psrB are found exclusively in prophages and are associated with effector loci and the T3S-activating Pch family of regulators. Like PatE, PsrA and PsrB are putative AraC/XylS family regulators [Citation60] comprising a C-terminal AraC-type HTH DNA-binding domain () that recognizes AT-rich DNA sequences and derepresses H-NS-repressed genes [Citation61]. The deletion of psrA increased T3SS activity, and this effect could be complemented by the introduction of psrA in trans. In contrast, the absence of PsrB has little effect on T3SS secretion, but when psrB is replenished in trans in the ΔpsrA mutant, it has a secretion inhibition effect [Citation30]. Both PsrA and PsrB hijacked the conserved GAD acid stress resistance system to regulate the T3SS by inducing the expression of GadE (YhiE) and YhiF (). Furthermore, the same study demonstrated that OI-50 is needed for persistence in an established ovine model of colonization and that the effector proteins on this cryptic prophage and the OI-50-encoded regulator PsrA both contribute to this persistence phenotype [Citation30]. PsrA and PsrB are generally coretained by the majority of clades of EHEC O157:H7 strains, except for their absence in clade 1 strains [Citation11], and six additional Psr homologs have been identified by pangenome analysis of EHEC, EPEC, and neonatal meningitis-associated E. coli (NMEC) isolates [Citation30], suggesting that these Psr regulatory proteins may play crucial roles in the evolution of non-pathogenic E. coli into pathogenic strains.
EtrA and EivF (OI-115)
EtrA and EivF, which are functional repressors of the LEE secretion systems in EHEC O157:H7 (), are encoded by etrA (z4184) and eivF (z4198), respectively, residing in the ETT2 gene cluster located in OI-115 of the EHEC O157:H7 EDL933 genome (). EviF is a homolog of the Salmonella Spi-1 regulator InvF [Citation31]. ETT2 regulator A (EtrA) [Citation33] contains an HTH DNA-binding motif that is 40% identical to the LuxR superfamily regulator GerE () [Citation34]. Mutational inhibition of etrA and eivF from the ETT2 cluster in the EHEC O157:H7 Sakai strain led to similar increases in the secretion of LEE-encoded proteins (i.e. EspP, TagA, Tir, EspB, and EspA) and increased adhesion to human intestinal cells [Citation32], demonstrating the cooperative or hierarchical regulation of the two regulators, regardless of whether they are located in different parts of the ETT2 cluster. Furthermore, upon introducing EtrA into the high-secretion EHEC O26:H- strain lacking eivF, a protein secretion suppression effect could still be detected under LEE-inducing conditions [Citation32], ruling out the possibility that EtrA functions via EivF. However, the molecular mechanisms by which these two regulators affect LEE expression and type III secretion profiles have not been elucidated and require further investigation.
OI-encoded motility regulatory proteins
Flagella facilitate swimming and swarming motility and function in multiple bacterial growth biology and virulence processes, including adhesion, biofilm formation, and host invasion [Citation62]. The flagellum of EHEC O157:H7 acts as an adhesion factor in the intestinal epithelium and is involved in a crucial initiation step for colonization [Citation63]. Flagellum synthesis and assembly are coordinated by three classes of genes under the control of hierarchical regulation. The Class I genes flhD and flhC located in one operon encode FlhD and FlhC, which form a complex and play global regulatory roles [Citation64] by binding upstream to the promoter of Class II genes [Citation65,Citation66]. Class II genes encode proteins for the basal flagellar apparatus and hook assembly as well as the regulator FliA for Class III gene control. Class III genes encode proteins for the remaining flagellum structure, among which the fliC gene encodes the major flagellin [Citation12]. FlhD and FlhC form a FlhD4C2 complex and boost the expression of flagellar genes via direct binding to FliA, an RNA polymerase sigma factor () [Citation64,Citation67]. Six flagellar regulatory proteins encoded by OIs have been characterized, including two activators (GmrA (encoded by OI-29) and Z5898 (encoded by OI-172)), two repressors (FmrA (encoded by OI-1) and RgdR (encoded by OI-51)), and the activator – repressor complex GrlR-GrlA (encoded by OI-148) ( and ).
Figure 5. Regulation of flagellar gene expression by OI-encoded regulatory proteins in EHEC O157:H7. OI-encoded regulatory proteins, including GmrA, Z5898, FmrA, RgdR and GrlA-GrlR, affect EHEC O157:H7 motility and flagellar biosynthesis.
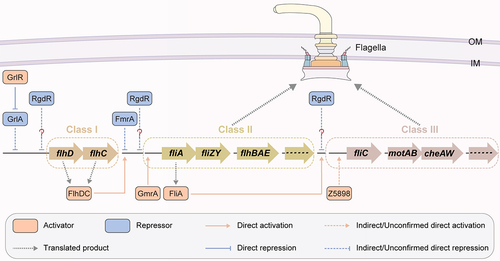
GmrA (OI-29)
Genomic island-encoded motility regulator A (GmrA) is encoded by z0639 in OI-29 of the EHEC O157:H7 EDL933 genome () [Citation35]. GmrA is a predicted transcription factor with putative DNA-binding activity (), and its binding to flagellar biosynthesis genes was recently characterized by our group [Citation35]. We found that deletion of gmrA or the entire OI-29 reduced bacterial motility compared with that of wild-type EHEC O157:H7, but lesion formation and Shiga toxin production were comparable [Citation35]. Both the transcription and translation levels of flagellar production genes (fliC and motA) were repressed upon OI-29 or gmrA deletion, and the mutated bacterial cells exhibited an aflagellar phenotype, demonstrating the activation of flagellar biosynthesis by GmrA. Furthermore, GmrA was shown to function by binding directly to the promoter region of the Class II flagellar gene fliA through in vitro EMSA and in vivo ChIP‒qPCR analysis, indicating that the regulatory effect of GmrA on fliC expression is mediated by its upstream regulator FliA () [Citation35].
GmrA was found to be widely distributed and highly conserved in various E. coli lineage isolates through analysis of 231 available genome sequences. Moreover, the functional conservation of GmrA in motility and flagellar synthesis regulation was identified in eight representative E. coli strains, including EHEC O157:H7, EPEC O55:H7, O127:H7, and APEC O2, and neonatal meningitis-associated O18 isolates, in which a mutant with an orthologous gmrA gene deletion exhibited a significantly reduced growth radius on motility plates and decreased fliC expression [Citation35]. Therefore, GmrA plays a conserved role in flagellar synthesis activation and pathogenesis regulation during the evolution of pathogenic E. coli.
Z5898 (OI-172)
Z5898, encoded by z5898 in OI-172 of the EHEC O157:H7 EDL933 genome (), is an RNA helicase containing a DEAD/DEAH box helicase domain () and is a positive regulator of flagellar synthesis and motility in EHEC O157:H7 () [Citation12]. The z5898 mutant showed decreased migration on semisolid agar plates and no flagellum formation, demonstrating the activating role of Z5898 in bacterial motility. Z5898 was demonstrated to function in promoting fliC gene expression by increasing its promoter activity, whereas it had no effect on the expression of fliA, the fliC upstream regulator-encoding gene () [Citation12]. However, whether the regulation of fliC by Z5898 occurs in a direct or indirect manner is still unclear, and whether the regulatory process involves the interaction of Z5898 with other regulatory proteins remains to be further investigated.
FmrA (OI-1)
Fimbrial-operon-encoded motility regulator A (FmrA) is a flagellar synthesis repressor encoded by z0021 located in OI-1 of the EHEC O157:H7 EDL933 genome (). OI-1 contains six ORFs from z0020 to z0025 and is a putative fimbrial genomic island that contains the fimbrial protein-encoding genes z0022 and z0024 [Citation37]. A previous study showed that fmrA or the entire OI-1 deletion mutant was highly motile and had increased flagellin production in comparison to that of wild-type EHEC O157:H7, whereas overexpression of fmrA could completely abolish swimming motility [Citation37], indicating the repressive function of FmrA in EHEC O157:H7 motility. Furthermore, the activity of all the Class II and III flagellar gene promoters was substantially greater in the ΔfmrA mutant strain than in the wild-type strain according to the transcriptional reporter activity. The significant increase in Class II (flgM) and III (fliC and motA) flagellar gene transcripts in the fmrA mutant strain was also confirmed by qRT‒PCR analysis [Citation37], demonstrating the repressive function of FmrA in flagellar genes (). However, flhDC promoter activity and expression levels were not affected by FmrA [Citation37]. Since the ClpXP protease has FlhD4C2 complex degradation activity [Citation68], the lack of interference by FmrA in FlhDC expression at the transcriptional and translational levels indicates that FmrA functions in a ClpXP-independent manner. Moreover, the repression function of FmrA in EHEC O157:H7 motility could be restored by the overexpression of flhDC, indicating that FmrA represses flagellar synthesis by preventing the global regulator complex FlhD4C2 from binding to Class II promoters () [Citation37]. However, the mechanism through which the FmrA-FlhD4C2 complex recognizes each component remains unclear.
RgdR (OI-51)
In addition to acting as an A/E formation promoter and T3SS activation factor, RgdR is also involved in the regulation of EHEC O157:H7 motility [Citation21]. The rgdR mutant exhibited induced motility, while the in-trans expression of rgdR from a low-copy-number plasmid repressed the motility level [Citation21], indicating the repressive role of RgdR in bacterial motility. Transcript expression of the rgdR variant C1493 from the UPEC CFT073 strain, which shares 83% sequence similarity with rgdR, in both the EHEC O157:H7 ΔrgdR and UPEC ΔrgdR deletion backgrounds significantly downregulated bacterial motility levels compared to those in the wild-type and deletion mutant strains [Citation21], demonstrating the conservation of the function of RgdR in motility repression in different E. coli pathotypes. However, whether RgdR controls flagellar synthesis-related gene expression remains unknown, and the underlying mechanism requires further characterization.
GrlA and GrlR (OI-148)
In addition to the direct activation of the LEE1 regulator, the OI-148 gene encoding GrlA was identified as a negative regulator of flagellar biosynthesis [Citation36]. The protein level of FliC, the main component of flagellar filaments, was highly increased upon deletion of clpXP, which encodes the GrlR-degrading protease ClpXP [Citation56], and the FliC level was significantly decreased without protein signal detection upon deletion of grlR [Citation36], suggesting a positive regulatory function of GrlR in flagellar protein synthesis. Furthermore, GrlR regulation in FliC was demonstrated to be mediated by the repressive function of GrlA, in which grlA overexpression via a multicopy plasmid significantly reduced the FliC protein level. Consistent with this, the grlR deletion mutant, but not the grlA or grlA/grlR deletion mutant, displayed a nonmotile phenotype compared with the wild-type EHEC O157:H7, demonstrating the repressive role of GrlA in flagellar synthesis and motility in the absence of GrlR. This study further characterized the regulatory mechanism of GrlA through β-galactosidase activity measurements and revealed that GrlA negatively regulates the expression of Class II and Class III flagellar genes by reducing the transcription of the flhDC operon (). However, the specific molecular mechanisms underlying the transcriptional control of flagellar genes by GrlA have not been fully elucidated. Since constitutive FlhD/FlhC expression inhibits EHEC adherence to HeLa cells [Citation36], it is hypothesized that GrlA-mediated flagellar regulon repression is pivotal for EHEC adhesion to host cells.
OI-encoded acid resistance regulatory proteins
As a food-borne pathogen, EHEC O157:H7 must survive in the acidic gastrointestinal tract to exert its pathogenesis, and pathogens have evolved elaborate acid resistance (AR) systems to enable survival [Citation69]. Four AR systems, namely, the oxidative or amino acid-independent acid resistance pathway 1 (AR1) and amino acid-dependent acid resistance pathways AR2 (i.e. glutamate dependent), AR3 (i.e. arginine dependent), and AR4 (i.e. lysine dependent), have been best characterized in E. coli [Citation70,Citation71]. AR1 mediates bacterial acid resistance when grown in the stationary phase and requires the stationary phase alternative sigma factor RpoS [Citation72]. AR2 can protect bacterial cells under acidic conditions with a pH as low as pH 2 and is characterized as the most efficient acid stress response system in E. coli [Citation70]. GadA and GadB, two decarboxylase isozymes, and the antiporter GadC are the main components of the AR2 system () [Citation73]. In addition, E. coli strains carry the acid stress chaperones encoded by the operon hdeAB (), which prevent the aggregation of periplasmic proteins at low pH [Citation74,Citation75]. Although equally remarkable levels of acid resistance have been detected in pathogenic and non-pathogenic E. coli isolates, EHEC O157:H7 is more acid resistant than non-pathogenic E. coli K-12 [Citation76]. Three regulatory proteins encoded by the unique OI genes of EHEC O157:H7 have been characterized as being involved in the regulation of bacterial AR, including the activators PatE (encoded by OI-8) and PsrB (encoded by OI-57) and the repressor GrvA (encoded by OI-47) ( and ).
Figure 6. Regulation of acid resistance-related gene expression by OI-encoded regulatory proteins in EHEC O157:H7. OI-encoded regulatory proteins, including PatE, PsrB and GrvA, affect the acid resistance and survival in acidic environments of EHEC O157:H7.
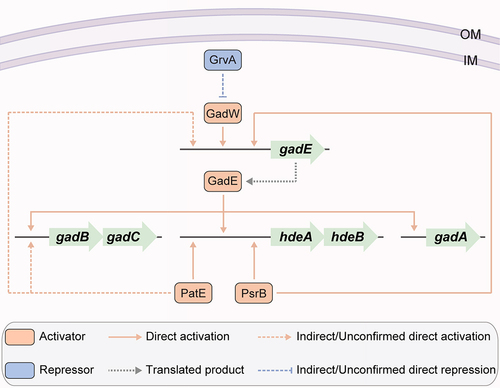
PatE (OI-8)
The OI-8-encoded PatE is an AR transcriptional activator [Citation29]. The patE-null mutant strain showed significant downregulation of AR genes, including the AR2 essential component-encoding genes gadB, gadC and gadE, as well as the AR chaperone gene hdeAB, compared to the wild-type EHEC O157:H7. The PatE activation locus at hdeA was further characterized as a cis-element between positions −132 and −45 of the hdeA promoter region () [Citation29]. Moreover, both patE and its activator protein-encoding gene rpoS were consistently expressed at higher levels in the stationary phase than in the exponential growth stage [Citation77], indicating the involvement of AR1 in PatE-regulated AR. Therefore, PatE-mediated AR cooperates with AR1, AR2, and AR chaperone regulation, affording EHEC O157:H7 the ability to adapt to acidic host environments such as the stomach [Citation29].
PsrB (OI-57)
The OI-57 gene encoding PsrB was also demonstrated to play a role in enhancing the acid resistance of EHEC O157:H7 under various acidic growth conditions, including in LB cultures at pH 2.5, 3.0, and 3.5 [Citation38]. It has been demonstrated that PsrB also contributes to bacterial survival in low-pH environments [Citation38], and PsrB-mediated acid resistance activation occurs through its direct binding activity to the cis-elements located at positions −132 and −45 of the hdeA promoter region [Citation38], the same binding region as that characterized for PatE () [Citation29,Citation38]. PsrB also activates gadE expression by directly binding to its promoter, which in turn activates the expression of AR2 genes via GadE () [Citation30,Citation38]. Furthermore, induction of PsrB expression in EHEC O157:H7 EDL933 significantly enhanced survival in mice, which allowed them to withstand the acidic barrier of the mouse stomach [Citation38], highlighting the significance of PsrB in enhancing acid resistance in EHEC O157:H7.
GrvA (OI-47)
The OI-47 gene, encoding GrvA, is also involved in the AR2 acid resistance regulation pathway. The grvA-null mutant strain exhibited upregulated expression of AR2 acid resistance genes, including gadA, gadBC, gadE, gadW and gadX, as well as a marked increase in AR capability during the exponential phase of growth [Citation39]. The mechanical repression function of GrvA in the AR2 central regulator GadE was further elucidated through the repression function of GrvA in GadW, which is an activator of gadE and directly controls gadE transcription from the P3 promoter () [Citation39]. Since the expression of AR2 acid resistance genes is tightly controlled in the exponential phase, whereas their expression is markedly increased in the stationary phase in wild-type EHEC O157:H7, resulting in the AR of stationary-phase cultures [Citation72], the repression of GrvA for AR is relegated to the exponential phase of growth [Citation39].
Conclusions and future perspectives
In conclusion, OIs play an important role in the regulation of EHEC O157 virulence, motility, and AR. Notably, in addition to the aforementioned regulatory proteins encoded by OI genes, additional OIs have been found to play potential roles in the T3SS, adhesion, motility, and other pathways. For example, deletion of OI-47, OI-76, and OI-151 in the EHEC strain TUV93–0, a Shiga toxin-negative derivative strain of EDL933, led to elevated T3S levels, whereas deletion of OI-70 and OI-133 reduced T3S levels [Citation78]. The OI-14, OI-15, and OI-52 deletion mutant strains displayed lower motility, whereas deletion of OI-1 and OI-44 in EHEC O57:H7 led to an enhanced motility phenotype [Citation37,Citation78]. Therefore, in future work, additional efforts are needed to functionally elucidate these OIs and experimentally characterize OI genes encoding regulatory proteins. These explorations are expected to be highly important for expanding the contribution of OIs to EHEC O157:H7 virulence during evolution.
Compared to those of the characterized OI-encoded virulence regulatory proteins, the discovery of regulatory proteins involved in motility, flagellum formation, and other environmental signalling adaptation regulatory pathways in EHEC O157:H7 is still relatively limited. Since the majority of OI genes are hereto-uncharacterized, there is great potential for functional exploration of these as-yet-uncharacterized OI-encoding proteins that may affect host colonization, signal sensing, virulence, and other biological pathways. To better understand the pathogenesis of EHEC O157:H7, additional efforts should be made to identify versatile biological pathways, and a more systematic and comprehensive study of regulators encoded by OIs is needed, such as by applying high-throughput experimental technologies, including chromatin immunoprecipitation followed by sequencing (ChIP‒Seq), protein‒protein interaction analysis, and transposon mutation library sequencing at specific growth stages. Functional characterization of additional OIs is critical not only for broadening the understanding of EHEC O157:H7 virulence regulatory mechanisms but also for understanding the phylogenetic evolution of pathogenic E. coli and developing strategies to diminish or eliminate pathogenic E. coli during host infection.
Shiga toxins (Stxs) are also important virulence factors of EHEC O157:H7 that cause damage to a variety of cell types and are often associated with exacerbated disease [Citation79,Citation80]. Stxs are divided into two groups, termed Stx1 and Stx2, which are 56% homologous at the amino acid level [Citation81]. Stxs are potent cytotoxins that bind to host globotriaosylceramide (Gb3) or globotetraosylceramide (Gb4) receptors, leading to the inhibition of protein synthesis through the specific removal of a single adenine residue from the 28S rRNA of the 60S ribosomal subunit [Citation80,Citation82]. However, the detailed mechanisms of Stx transcription, translation and translocation to various tissues are not fully understood. To our knowledge, there are currently no reports showing that OI-encoded regulatory proteins are involved in the regulation of Stxs or Stx prophages. Therefore, additional studies are needed to elucidate the regulatory effects of these OI-encoded regulatory proteins on Stxs.
Different biological pathways often interconnect spatially or temporally, triggered by a group of genes, proteins, and metabolites [Citation83]. In E. coli, it is well known that flagellum production is associated with the virulence of EHEC O157:H7, and it has been shown that flagellum production varies among different infection stages, with flagella being produced at early stages for attachment of bacterial cells but not expressed in bacterial cells at later time points [Citation63]. Consistent with these findings, the regulatory proteins exhibited differences in expression levels among the different infection stages. For example, the expression of the flagellum production activator GmrA increased in the first 2 h of cocultivation with epithelial cells [Citation35], and a dramatic reduction in the level was detected at 3 h post infection, while the expression of the flagellum production repressor FmrA was not detected, suggesting that GmrA and FmrA play roles in the initial infection phase and post early infection stage, respectively [Citation84]. In addition, the use of one regulatory protein to coordinate multiple biological pathways, such as the involvement of GrvA in both LEE gene expression activation and AR repression, is a common regulatory strategy in cells () [Citation39]. However, how these regulatory proteins temporally coordinate relevant biological pathways and how these different pathway regulatory factors interact with each other at the same infection stages remain unclear and await further elucidation. A high-throughput functional understanding of multiple putative regulators under different growth conditions or infection stages and revealing their regulatory networks are potential directions for future research.
Acknowledgements
This work was supported by the National Natural Science Foundation of China (NSFC) Program (grant nos. 32270191, 32200024 and 82072212) and Fundamental Research Funds for the Central Universities (grant no. 63233172).
Data availability statement
The data that support the findings of this study are openly available in the National Library of Medicine at https://www.ncbi.nlm.nih.gov/.
Disclosure statement
No potential conflict of interest was reported by the author(s).
Additional information
Funding
References
- Karmali MA. Infection by verocytotoxin-producing Escherichia coli. Clin Microbiol Rev. 1989;2(1):15‒38. doi: 10.1128/CMR.2.1.15
- Caprioli A, Morabito S, Brugere H, et al. Enterohaemorrhagic Escherichia coli: emerging issues on virulence and modes of transmission. Vet Res. 2005;36(3):289‒311. doi: 10.1051/vetres:2005002
- Watanabe H. Pathogenesis of enterohaemorrhagic Escherichia coli infection. Nihon Rinsho. 2012;70:1318‒1322.
- Berger CN, Sodha SV, Shaw RK, et al. Fresh fruit and vegetables as vehicles for the transmission of human pathogens. Environ Microbiol. 2010;12(9):2385‒2397. doi: 10.1111/j.1462-2920.2010.02297.x
- Rangel JM, Sparling PH, Crowe C, et al. Epidemiology of Escherichia coli O157:H7 Outbreaks, United States, 1982–2002. Emerg Infect Dis. 2005;11(4):603‒609. doi: 10.3201/eid1104.040739
- Schmid-Hempel P, Frank SA, Manchester M. Pathogenesis, virulence, and infective dose. PLoS Pathog. 2007;3(10):1372‒1373. doi: 10.1371/journal.ppat.0030147
- Franzin FM, Sircili MP. Locus of enterocyte effacement: a pathogenicity island involved in the virulence of enteropathogenic and enterohemorragic Escherichia coli subjected to a complex network of gene regulation. Biomed Res Int. 2015;2015:534738. doi: 10.1155/2015/534738
- Slater SL, Sågfors AM, Pollard DJ, et al. The type III secretion system of pathogenic Escherichia coli. Curr Top Microbiol Immunol. 2018:51‒72. doi: 10.1007/82_2018_116
- McDaniel TK, Jarvis KG, Donnenberg MS, et al. A genetic locus of enterocyte effacement conserved among diverse enterobacterial pathogens. Proc Natl Acad Sci U S A. 1995;92(5):1664‒1668. doi: 10.1073/pnas.92.5.1664
- Hayashi T, Makino K, Ohnishi M, et al. Complete genome sequence of enterohemorrhagic Escherichia coli O157: H7 and genomic comparison with a laboratory strain K-12. DNA Res. 2001;8(1):11‒22. doi: 10.1093/dnares/8.1.11
- Jiang L, Yang W, Jiang X, et al. Virulence-related O islands in enterohemorrhagic Escherichia coli O157: H7. Gut Microbes. 2021;13(1):1992237. doi: 10.1080/19490976.2021.1992237
- Xu Y, Xu X, Lan R, et al. An O island 172 encoded RNA helicase regulates the motility of Escherichia coli O157: H7. PLoS One. 2013;8(6):e64211. doi: 10.1371/journal.pone.0064211
- Connolly JPR, O’Boyle N, Turner NCA, et al. Distinct intraspecies virulence mechanisms regulated by a conserved transcription factor. Proc Natl Acad Sci U S A. 2019;116(39):19695‒19704. doi: 10.1073/pnas.1903461116
- Mellies JL, Barron AM, Carmona AM. Enteropathogenic and enterohemorrhagic Escherichia coli virulence gene regulation. Infect Immun. 2007;75(9):4199‒4210. doi: 10.1128/IAI.01927-06
- Yang B, Feng L, Wang F, et al. Enterohemorrhagic Escherichia coli senses low biotin status in the large intestine for colonization and infection. Nat Commun. 2015;6(1):6592. doi: 10.1038/ncomms7592
- Sharma VK, Zuerner RL. Role of hha and ler in transcriptional regulation of the esp operon of enterohemorrhagic Escherichia coli O157: H7. J Bacteriol. 2004;186(21):7290‒7301. doi: 10.1128/JB.186.21.7290-7301.2004
- Liu Y, Liu B, Yang P, et al. LysR-type transcriptional regulator OvrB encoded in O island 9 drives enterohemorrhagic Escherichia coli O157:H7 virulence. Virulence. 2019;10(1):783‒792. doi: 10.1080/21505594.2019.1661721
- Liu B, Wang J, Wang L, et al. Transcriptional activator OvrA encoded in O island 19 modulates virulence gene expression in enterohemorrhagic Escherichia coli O157:H7. J Infect Dis. 2020;221:820‒829. doi: 10.1093/infdis/jiz458
- Hernandez-Doria JD, Sperandio V. Bacteriophage transcription factor Cro regulates virulence gene expression in enterohemorrhagic Escherichia coli. Cell Host Microbe. 2018;23(5):607‒617.e6. doi: 10.1016/j.chom.2018.04.007
- Tobe T, Ando H, Ishikawa H, et al. Dual regulatory pathways integrating the RcsC–RcsD–RcsB signalling system control enterohaemorrhagic Escherichia coli pathogenicity. Mol Microbiol. 2005;58(1):320‒333. doi: 10.1111/j.1365-2958.2005.04828.x
- Flockhart AF, Tree JJ, Xu X, et al. Identification of a novel prophage regulator in Escherichia coli controlling the expression of type III secretion. Mol Microbiol. 2012;83(1):208‒223. doi: 10.1111/j.1365-2958.2011.07927.x
- D’Souza SE, Ginsberg MH, Plow EF. Arginyl-glycyl-aspartic acid (RGD): A cell adhesion motif. Trends Biochem Sci. 1991;16:246‒250. doi: 10.1016/0968-0004(91)90096-e
- Kendall MM, Rasko DA, Sperandio V. The LysR-type regulator QseA regulates both characterized and putative virulence genes in enterohaemorrhagic Escherichia coli O157: H7. Mol Microbiol. 2010;76(5):1306‒1321. doi: 10.1111/j.1365-2958.2010.07174.x
- Luzader DH, Willsey GG, Wargo MJ, et al. The type three secretion system 2-encoded regulator EtrB modulates enterohemorrhagic Escherichia coli virulence gene expression. Infect Immun. 2016;84(9):2555‒2565. doi: 10.1128/IAI.00407-16
- Liu Y, Han R, Wang J, et al. Magnesium sensing regulates intestinal colonization of enterohemorrhagic Escherichia coli O157:H7. MBio. 2020;11(6):11. doi: 10.1128/mBio.02470-20
- Feng L, Yang B, Xu Y, et al. Elucidation of a complete mechanical signaling and virulence activation pathway in enterohemorrhagic Escherichia coli. Cell Rep. 2022;39(1):110614. doi: 10.1016/j.celrep.2022.110614
- Islam MS, Bingle LE, Pallen MJ, et al. Organization of the LEE1 operon regulatory region of enterohaemorrhagic Escherichia coli O157: H7 and activation by GrlA. Mol Microbiol. 2011;79(2):468‒483. doi: 10.1111/j.1365-2958.2010.07460.x
- Padavannil A, Jobichen C, Mills E, et al. Structure of GrlR–GrlA complex that prevents GrlA activation of virulence genes. Nat Commun. 2013;4(1):2546. doi: 10.1038/ncomms3546
- Bender JK, Praszkier J, Wakefield MJ, et al. Involvement of PatE, a prophage-encoded AraC-like regulator, in the transcriptional activation of acid resistance pathways of enterohemorrhagic Escherichia coli strain EDL933. Appl Environ Microbiol. 2012;78(15):5083‒5092. doi: 10.1128/AEM.00617-12
- Tree JJ, Roe AJ, Flockhart A, et al. Transcriptional regulators of the GAD acid stress island are carried by effector protein-encoding prophages and indirectly control type III secretion in enterohemorrhagic Escherichia coli O157: H7. Mol Microbiol. 2011;80(5):1349‒1365. doi: 10.1111/j.1365-2958.2011.07650.x
- Darwin KH, Miller VL. InvF is required for expression of genes encoding proteins secreted by the SPI1 type III secretion apparatus in salmonella typhimurium. J Bacteriol. 1999;181(16):4949‒4954. doi: 10.1128/JB.181.16.4949-4954.1999
- Zhang L, Chaudhuri RR, Constantinidou C, et al. Regulators encoded in the Escherichia coli type III secretion system 2 gene cluster influence expression of genes within the locus for enterocyte effacement in enterohemorrhagic E. coli O157: H7. Infect Immun. 2004;72(12):7282‒7293. doi: 10.1128/IAI.72.12.7282-7293.2004
- Ren CP, Chaudhuri RR, Fivian A, et al. The ETT2 gene cluster, encoding a second type III secretion system from Escherichia coli, is present in the majority of strains but has undergone widespread mutational attrition. J Bacteriol. 2004;186(11):3547‒3560. doi: 10.1128/JB.186.11.3547-3560.2004
- Wang S, Xu X, Liu X, et al. Escherichia coli type III secretion system 2 regulator EtrA promotes virulence of avian pathogenic Escherichia coli. Microbiol (Reading). 2017;163(10):1515‒1524. doi: 10.1099/mic.0.000525
- Yang B, Wang S, Huang J, et al. Transcriptional activator GmrA, encoded in genomic island OI-29, controls the motility of enterohemorrhagic Escherichia coli O157: H7. Front Microbiol. 2018;9:338. doi: 10.3389/fmicb.2018.00338
- Iyoda S, Koizumi N, Satou H, et al. The GrlR-GrlA regulatory system coordinately controls the expression of flagellar and LEE-encoded type III protein secretion systems in enterohemorrhagic Escherichia coli. J Bacteriol. 2006;188(16):5682‒5692. doi: 10.1128/JB.00352-06
- Allison SE, Silphaduang U, Mascarenhas M, et al. Novel repressor of Escherichia coli O157: H7 motility encoded in the putative fimbrial cluster OI-1. J Bacteriol. 2012;194(19):5343‒5352. doi: 10.1128/JB.01025-12
- Yang J, Russell TW, Hocking DM, et al. Control of acid resistance pathways of enterohemorrhagic Escherichia coli strain EDL933 by PsrB, a prophage-encoded AraC-like regulator. Infect Immun. 2015;83(1):346‒353. doi: 10.1128/IAI.02758-14
- Morgan JK, Carroll RK, Harro CM, et al. Global regulator of virulence a (GrvA) coordinates expression of discrete pathogenic mechanisms in enterohemorrhagic Escherichia coli through interactions with GadW-GadE. J Bacteriol. 2016;198(3):394‒409. doi: 10.1128/JB.00556-15
- Cordeiro TN, Schmidt H, Madrid C, et al. Indirect DNA readout by an H-NS related protein: Structure of the DNA complex of the C-terminal domain of Ler. PLoS Pathog. 2011;7(11):e1002380. doi: 10.1371/journal.ppat.1002380
- Elliott SJ, Yu J, Kaper JB, et al. The cloned locus of enterocyte effacement from enterohemorrhagic Escherichia coli O157: H7 is unable to confer the attaching and effacing phenotype upon E. coli K-12. Infect Immun. 1999;67(8):4260‒4263. doi: 10.1128/IAI.67.8.4260-4263.1999
- McDaniel TK, Kaper JB. A cloned pathogenicity island from enteropathogenic Escherichia coli confers the attaching and effacing phenotype on E. coli K-12. Mol Microbiol. 1997;23(2):399‒407. doi: 10.1046/j.1365-2958.1997.2311591.x
- Habdas BJ, Smart J, Kaper JB, et al. The LysR-type transcriptional regulator QseD alters type three secretion in enterohemorrhagic Escherichia coli and motility in K-12 Escherichia coli. J Bacteriol. 2010;192(14):3699‒3712. doi: 10.1128/JB.00382-10
- Maddocks SE, Oyston PCF. Structure and function of the LysR-type transcriptional regulator (LTTR) family proteins. Microbiol (Reading). 2008;154(12):3609‒3623. doi: 10.1099/mic.0.2008/022772-0
- Hall BM, Vaughn EE, Begaye AR, et al. Reengineering Cro protein functional specificity with an evolutionary code. J Mol Biol. 2011;413(5):914‒928. doi: 10.1016/j.jmb.2011.08.056
- Kuper C, Jung K. CadC-mediated activation of the cadBA promoter in Escherichia coli. J Mol Microbiol Biotechnol. 2005;10:26‒39. doi: 10.1159/000090346
- Tukel C, Akcelik M, de Jong MF, et al. MarT activates expression of the MisL autotransporter protein of Salmonella enterica serotype Typhimurium. J Bacteriol. 2007;189(10):3922‒3926. doi: 10.1128/JB.01746-06
- Morgan JK, Vendura KW, Stevens SM, et al. RcsB determines the locus of enterocyte effacement (LEE) expression and adherence phenotype of Escherichia coli O157: H7 spinach outbreak strain TW14359 and coordinates bicarbonate-dependent LEE activation with repression of motility. Microbiol (Reading). 2013;159(Pt_11):2342‒2353. doi: 10.1099/mic.0.070201-0
- Shen S, Mascarenhas M, Morgan R, et al. Identification of four fimbria-encoding genomic islands that are highly specific for verocytotoxin-producing Escherichia coli serotype O157 strains. J Clin Microbiol. 2005;43(8):3840‒3850. doi: 10.1128/JCM.43.8.3840-3850.2005
- Cooper KK, Mandrell RE, Louie JW, et al. Comparative genomics of enterohemorrhagic Escherichia coli O145: H28 demonstrates a common evolutionary lineage with Escherichia coli O157: H7. BMC Genomics. 2014;15(1):17. doi: 10.1186/1471-2164-15-17
- Jaureguy F, Landraud L, Passet V, et al. Phylogenetic and genomic diversity of human bacteremic Escherichia coli strains. BMC Genomics. 2008;9(1):560. doi: 10.1186/1471-2164-9-560
- Lostroh CP, Lee CA. The Salmonella pathogenicity island-1 type III secretion system. Microbes Infect. 2001;3(14–15):1281‒1291. doi: 10.1016/s1286-4579(01)01488-5
- Baikalov I, Schroder I, Kaczor-Grzeskowiak M, et al. Structure of the Escherichia coli response regulator NarL. Biochemistry. 1996;35(34):11053‒11061. doi: 10.1021/bi960919o
- Yuan J, Jin F, Glatter T, et al. Osmosensing by the bacterial PhoQ/PhoP two-component system. Proc Natl Acad Sci U S A. 2017;114(50):E10792–E10798. doi: 10.1073/pnas.1717272114
- Deng W, Puente JL, Gruenheid S, et al. Dissecting virulence: systematic and functional analyses of a pathogenicity island. Proc Natl Acad Sci U S A. 2004;101(10):3597‒3602. doi: 10.1073/pnas.0400326101
- Iyoda S, Watanabe H. ClpXP protease controls expression of the type III protein secretion system through regulation of RpoS and GrlR levels in enterohemorrhagic Escherichia coli. J Bacteriol. 2005;187(12):4086‒4094. doi: 10.1128/JB.187.12.4086-4094.2005
- Saitoh T, Iyoda S, Yamamoto S, et al. Transcription of the ehx enterohemolysin gene is positively regulated by GrlA, a global regulator encoded within the locus of enterocyte effacement in enterohemorrhagic Escherichia coli. J Bacteriol. 2008;190(14):4822‒4830. doi: 10.1128/JB.00231-08
- Jimenez R, Cruz-Migoni SB, Huerta-Saquero A, et al. Molecular characterization of GrlA, a specific positive regulator of ler expression in enteropathogenic Escherichia coli. J Bacteriol. 2010;192(18):4627‒4642. doi: 10.1128/JB.00307-10
- Lara-Ochoa C, Huerta-Saquero A, Medrano-Lopez A, et al. GrlR, a negative regulator in enteropathogenic E. coli, also represses the expression of LEE virulence genes independently of its interaction with its cognate partner GrlA. Front Microbiol. 2023;14:1063368. doi: 10.3389/fmicb.2023.1063368
- Gallegos MT, Schleif R, Bairoch A, et al. Arac/XylS family of transcriptional regulators. Microbiol Mol Biol Rev. 1997;61(4):393‒410. doi: 10.1128/mmbr.61.4.393-410.1997
- Yang J, Hart E, Tauschek M, et al. Bicarbonate-mediated transcriptional activation of divergent operons by the virulence regulatory protein, RegA, from citrobacter rodentium. Mol Microbiol. 2008;68(2):314‒327. doi: 10.1111/j.1365-2958.2008.06171.x
- Liu R, Ochman H. Stepwise formation of the bacterial flagellar system. Proc Natl Acad Sci U S A. 2007;104(17):7116‒7121. doi: 10.1073/pnas.0700266104
- Mahajan A, Currie CG, Mackie S, et al. An investigation of the expression and adhesion function of H7 flagella in the interaction of Escherichia coli O157: H7 with bovine intestinal epithelium. Cell Microbiol. 2009;11(1):121‒137. doi: 10.1111/j.1462-5822.2008.01244.x
- Claret L, Hughes C. Functions of the subunits in the FlhD(2)C(2) transcriptional master regulator of bacterial flagellum biogenesis and swarming. J Mol Biol. 2000;303(4):467‒478. doi: 10.1006/jmbi.2000.4149
- Liu X, Matsumura P. The FlhD/FlhC complex, a transcriptional activator of the Escherichia coli flagellar class II operons. J Bacteriol. 1994;176(23):7345‒7351. doi: 10.1128/jb.176.23.7345-7351.1994
- Wang S, Fleming RT, Westbrook EM, et al. Structure of the Escherichia coli FlhDC complex, a prokaryotic heteromeric regulator of transcription. J Mol Biol. 2006;355(4):798‒808. doi: 10.1016/j.jmb.2005.11.020
- Chevance FF, Hughes KT. Coordinating assembly of a bacterial macromolecular machine. Nat Rev Microbiol. 2008;6(6):455‒465. doi: 10.1038/nrmicro1887
- Kitagawa R, Takaya A, Yamamoto T. Dual regulatory pathways of flagellar gene expression by ClpXP protease in enterohaemorrhagic Escherichia coli. Microbiol (Reading). 2011;157(11):3094‒3103. doi: 10.1099/mic.0.051151-0
- Foster JW. Escherichia coli acid resistance: tales of an amateur acidophile. Nat Rev Microbiol. 2004;2(11):898‒907. doi: 10.1038/nrmicro1021
- Zhao B, Houry WA. Acid stress response in enteropathogenic gammaproteobacteria: an aptitude for survival. This paper is one of a selection of papers published in this special issue entitled “Canadian Society of Biochemistry, molecular & cellular biology 52nd annual meeting — protein folding: principles and diseases” and has undergone the Journal’s usual peer review process. Biochem Cell Biol. 2010;88(2):301–17. doi: 10.1139/o09-182
- Foster JW. When protons attack: microbial strategies of acid adaptation. Curr Opin Microbiol. 1999;2(2):170‒174. doi: 10.1016/S1369-5274(99)80030-7
- Castanie-Cornet MP, Penfound TA, Smith D, et al. Control of acid resistance in Escherichia coli. J Bacteriol. 1999;181(11):3525‒3535. doi: 10.1128/JB.181.11.3525-3535.1999
- Bearson S, Bearson B, Foster JW. Acid stress responses in enterobacteria. FEMS Microbiol Lett. 1997;147(2):173‒180. doi: 10.1111/j.1574-6968.1997.tb10238.x
- Gajiwala KS, Burley SK. HDEA, a periplasmic protein that supports acid resistance in pathogenic enteric bacteria 1 1Edited by P. E. Wright. J Mol Biol. 2000;295(3):605‒612. doi: 10.1006/jmbi.1999.3347
- Kern R, Malki A, Abdallah J, et al. Escherichia coli HdeB is an acid stress chaperone. J Bacteriol. 2007;189(2):603‒610. doi: 10.1128/JB.01522-06
- McClure PJ, Hall S. Survival of Escherichia coli in foods. Symp Ser Soc Appl Microbiol. 2000;88(S1):61S‒70S. doi: 10.1111/j.1365-2672.2000.tb05333.x
- Dong T, Schellhorn HE. Global effect of RpoS on gene expression in pathogenic Escherichia coli O157: H7 strain EDL933. BMC Genomics. 2009;10(1):349. doi: 10.1186/1471-2164-10-349
- Flockhart AF Analysis of O-island deletions in Escherichia coli O157:H7. Thesis presented for the degree of Doctor of Philosophy. 2012.
- Dos Santos GF, de Sousa FG, Beier SL, et al. Escherichia coli O157: H7 strains in bovine carcasses and the impact on the animal production chain. Braz J Microbiol. 2023;54(3):2243‒2251. doi: 10.1007/s42770-023-01034-x
- Hall G, Kurosawa S, Stearns-Kurosawa DJ. Shiga toxin therapeutics: beyond neutralization. Toxins (Basel). 2017;9(9):291. doi: 10.3390/toxins9090291
- Shimizu T, Ohta Y, Tsutsuki H, et al. Construction of a novel bioluminescent reporter system for investigating shiga toxin expression of enterohemorrhagic Escherichia coli. Gene. 2011;478(1–2):1‒10. doi: 10.1016/j.gene.2011.01.006
- Detzner J, Pohlentz G, Muthing J. Enterohemorrhagic Escherichia coli and a fresh view on shiga toxin-binding glycosphingolipids of primary human kidney and colon epithelial cells and their toxin susceptibility. Int J Mol Sci. 2022;23(13):6884. doi: 10.3390/ijms23136884
- Minadakis G, Spyrou GM. A systems bioinformatics approach to interconnect biological pathways. Methods Mol Biol. 2021;2189:231‒249. doi: 10.1007/978-1-0716-0822-7_17
- Low AS, Holden N, Rosser T, et al. Analysis of fimbrial gene clusters and their expression in enterohaemorrhagic Escherichia coli O157: H7. Environ Microbiol. 2006;8(6):1033‒1047. doi: 10.1111/j.1462-2920.2006.00995.x