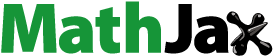
ABSTRACT
Animal models that can replicate clinical and pathologic features of severe human coronavirus infections have been instrumental in the development of novel vaccines and therapeutics. The goal of this review is to summarize our current understanding of the pathogenesis of coronavirus disease 2019 (COVID-19) and the pathologic features that can be observed in several currently available animal models. Knowledge gained from studying these animal models of SARS-CoV-2 infection can help inform appropriate model selection for disease modelling as well as for vaccine and therapeutic developments.
Introduction
The family Coronaviridae contains viruses that infect a wide range of mammals with varying pathogenicity. The family is classified into the subfamilies Orthocoronavirinae and Letovirinae, the latter of which contains a single virus isolated from the ornamented pygmy frog (Microhyla fissipes) [Citation1]. The subfamily Orthocoronavirinae is further classified into the genera Alphacoronavirus, Betacoronavirus, Deltacoronavirus, and Gammacoronavirus. Alphacoronaviruses include human coronavirus 229E (HCoV-229E) and HCoV-NL63 that typically cause common cold symptoms, and viruses infecting bats, pigs, cats, and dogs [Citation2]. Of greater concern are the new human betacoronaviruses that have caused three outbreaks in the last 20 years: severe acute respiratory syndrome coronavirus (SARS-CoV), SARS-CoV-2, and Middle East respiratory syndrome coronavirus (MERS-CoV). These viruses are primary respiratory pathogens transmitted by inhalation of respiratory droplets and aerosols, direct contact with respiratory secretions [Citation3–5], and possibly via faecal-oral route [Citation6,Citation7]. Gamma- and deltacoronaviruses infect birds, pigs, and other mammals but have not been documented in people [Citation2,Citation8].
Coronaviruses are enveloped, positive and single-stranded RNA viruses with a large, approximately 30 kilobase genome. The genome encodes for structural, non-structural, and accessory proteins. The four structural proteins, the spike (S), envelope (E), membrane (M), and nucleocapsid (N), form the virus particle (). A large portion of the genome encodes for either 15 or 16 non-structural proteins (nsp) that are largely involved in replication and transcription [Citation10]. Major nsps like the highly conserved RNA-dependent RNA polymerase nsp12 and the main protease nsp5 are targets of antiviral drug development [Citation11]. Finally, coronaviruses encode for up to 11 accessory proteins that play a role in host–pathogen interactions, including immune evasion [Citation12].
Figure 1. Structure and genomic organization of SARS-CoV-2. The virion (a) consists of an unsegmented, positive ssRNA genome complexed with the nucleocapsid protein (n). Envelope-associated structural proteins include the trimeric spike (s), membrane (m), and envelope (e) proteins. Open reading frames (ORF) 1a and 1b are directly translated into the numerous nonstructural proteins (nsps) that form the replicase-transcriptase complex, including the RdRp. The remaining structural and accessory proteins (3a through 10) are derived from subgenomic mRnas (b). For additional information on coronavirus replication strategies, please see ref [Citation9].
![Figure 1. Structure and genomic organization of SARS-CoV-2. The virion (a) consists of an unsegmented, positive ssRNA genome complexed with the nucleocapsid protein (n). Envelope-associated structural proteins include the trimeric spike (s), membrane (m), and envelope (e) proteins. Open reading frames (ORF) 1a and 1b are directly translated into the numerous nonstructural proteins (nsps) that form the replicase-transcriptase complex, including the RdRp. The remaining structural and accessory proteins (3a through 10) are derived from subgenomic mRnas (b). For additional information on coronavirus replication strategies, please see ref [Citation9].](/cms/asset/b2d6243c-2d37-4cc4-a0ef-c2675963df75/kvir_a_2316438_f0001_oc.jpg)
There are many documented instances of cross-s pecies jumping and zoonotic transmission of coronaviruses (reviewed in ref [Citation13]). It is generally accepted that most human coronaviruses originated in related bat coronaviruses [Citation14–16] with infection of several mammalian intermediate hosts [Citation17–20] leading to zoonotic spillover. Factors such as climate change, land management practices, wildlife trade, and urbanization bring humans and animals into ever-increasing contact [Citation21], which all but guarantees the future emergence of zoonotic coronaviruses or other viruses of pandemic potential.
Non-pharmaceutical interventions, including stay-at-home orders, masking, and social distancing, were widely adopted during the coronavirus disease 2019 (COVID-19) pandemic, and contributed to significantly reduced transmission [Citation22]. As with other infectious diseases, vaccination is the most effective prevention for severe COVID-19 disease. It is estimated that in the first year of immunization, COVID-19 vaccines reduced the total number of deaths worldwide by 63% [Citation23]. Currently available vaccines use the S protein as an immunogen as it is the major target for neutralizing antibodies [Citation24,Citation25] that are a highly predictive correlate of immune protection for SARS-CoV-2 [Citation26]. Treatments for COVID-19 include monoclonal antibodies and the antivirals remdesivir (a nucleotide analog available only as an intravenous infusion) and nirmatrelvir-ritonavir (Paxlovid®), among others (reviewed in ref [Citation27]). As new variants of SARS-CoV-2 arise and the threat of newly emerging coronaviruses continues, development of pan-coronavirus vaccines and antivirals is greatly needed.
The purpose of this review is to describe important animal models of COVID-19 with an emphasis on comparative pathology. Where applicable, salient features of SARS-CoV and MERS-CoV will also be discussed.
Symptoms of human coronavirus infections
Patients infected with SARS-CoV, MERS-CoV, or SARS-CoV-2 typically present with flu-like symptoms, including fever, non-productive cough, myalgia, and lethargy, although infections may be asymptomatic [Citation28–33]. Diarrhea, vomiting, and abdominal pain have also been reported [Citation30,Citation31]. Gastrointestinal symptoms are particularly common with COVID-19 and may occur prior to respiratory symptoms [Citation34]. Alterations in smell and/or taste have been reported in up to 47% of people infected with SARS-CoV-2 [Citation35]. Olfactory dysfunction may persist as a part of long COVID, a post-infection condition consisting of non-specific symptoms affecting multiple organs [Citation36]. While symptoms of these three coronavirus infections are similar, case fatality rates are drastically different at approximately 36% [Citation37], 10% [Citation38], and 0.5–4% [Citation39] for SARS-CoV, MERS-CoV, and SARS-CoV-2, respectively.
Patients with COVID-19 develop dyspnoea and tachypnoea with worsening respiratory disease, which typically occurs about 1 week after the initial onset of symptoms [Citation31,Citation32]. Pneumonia may progress to acute respiratory distress syndrome (ARDS), which is defined clinically as acute respiratory symptoms with bilateral opacities on chest imaging, hypoxaemia, and oedema with no evidence of cardiac failure or fluid overload [Citation40]. Comorbidities such as hypertension, cardiovascular disease, chronic obstructive pulmonary disease, chronic renal disease, and diabetes are common in severe coronavirus disease [Citation30–32,Citation41].
Molecular mechanisms of coronavirus infections
Coronaviruses attach to host cells through interactions between the S protein and various cellular receptors, which dictates the species and tissue specificity of the virus. The receptor-binding domain (RBD) of the S1 subunit of the S protein binds to the host cell, leading to conformational changes and fusion with the cellular membrane mediated by the S2 subunit of S [Citation2,Citation37]. The human viruses SARS-CoV [Citation38], SARS-CoV-2 [Citation39,Citation42], and HCoV-NL63 [Citation43] bind to angiotensin converting enzyme 2 (ACE2), a transmembrane protein involved in blood pressure and cardiac regulation [Citation44]. ACE2 receptors are found on epithelial cells of the respiratory and gastrointestinal tracts [Citation45] as well as the endothelium [Citation45], heart, kidneys, thyroid, liver, and brain [Citation46,Citation47]. In contrast, MERS-CoV binds dipeptidyl peptidase 4 [Citation48], a cell surface enzyme with widespread tissue distribution [Citation49–51]. Other coronaviruses bind aminopeptidase N (HCoV-229E, porcine coronaviruses, and some feline coronaviruses) or sialic acid residues (HCoV-OC43 and bovine coronavirus) [Citation52–55]. Further descriptions will mainly focus on SARS-CoV-2.
SARS-CoV-2 initially infects ciliated respiratory epithelial cells [Citation56] and/or sustentacular olfactory cells [Citation57] of the upper respiratory tract. In the face of inadequate immune control, the virus most likely reaches the lung by inhalation of viral particles from upper respiratory and oropharyngeal secretions [Citation58]. Once within the cell, coronaviruses replicate within double-membrane vesicles thatmay shield the virus from double-stranded RNA innate immune sensors [Citation59,Citation60]. SARS-CoV-2, like SARS-CoV and MERS-CoV, evades host antiviral responses by avoiding innate recognition [Citation59–61] and suppressing production of interferons (IFN) and IFN-stimulated genes (ISGs) [Citation62–65], which allows for continued viral replication.
Host recognition of SARS-CoV-2 by the innate immune system and downstream inflammatory responses play a significant role in the pathogenesis of COVID-19. As this topic has been reviewed elsewhere [Citation66,Citation67], it will only be briefly discussed here. Interferons have been studied extensively, although their precise role in the mechanisms of severe disease remains somewhat unclear. Zhang et al. [Citation68] detected genetic defects in TLR3- and IRF7-dependent type I IFN pathways in 3.5% of patients with life-threatening COVID-19 pneumonia. Autoantibodies against type I IFN were also found in 10.2% of patients with severe COVID-19 pneumonia but not in those with asymptomatic or mild disease [Citation69]. These studies support an essential antiviral role of type I IFN in SARS-CoV-2 infection. In corroboration, early reports showed blunted type I [Citation70,Citation71] and type III [Citation70] IFN responses from post-mortem lung samples [Citation70] and serum samples [Citation70] in patients with severe disease. In both studies, this was juxtaposed with excess proinflammatory chemokines and cytokines, especially IL-6. However, several subsequent reports show elevated type I IFN and their downstream ISGs in severe cases of COVID-19 [Citation72–74] that could contribute to excess inflammation. These discrepancies could be explained by the different tissue types, methodologies, and/or differences in disease stage at time of analysis. Interferons have been investigated as potential treatment modalities for COVID-19 with varying levels of success and some instances of worse outcomes [Citation75–78]. A recent phase 3 clinical trial found that early administration (within 7 days of symptom onset) of a single dose of pegylated IFN led to a 51% reduction in the risk of hospitalization or visit to an emergency department [Citation79]. Because of varying clinical trial results, use of IFNλ and IFNβ is currently not recommended, while use of IFN
is still under investigation and not available for clinical use [Citation80].
SARS-CoV-2 pathology
Viral pneumonia due to SARS-CoV-2 is typified by diffuse alveolar damage (DAD) that leads to acute respiratory distress syndrom (ARDS) [Citation28,Citation29,Citation81–87]. Specifically, the lungs are heavy and oedematous with patchy to confluent areas of consolidation. Acute exudative DAD, characterized by hyaline membranes, loss of pneumocytes, interstitial and alveolar oedema, congestion, and interstitial mononuclear inflammation, is present in cases of shorter duration (less than approximately 10 days) [Citation81,Citation86]. As the pneumonia progresses, type II pneumocytes begin to proliferate to cover denuded alveolar basement membranes. These hyperplastic type II pneumocytes may display features of atypia, such as cytomegaly and enlarged nuclei with prominent nucleoli or multinucleation [Citation81,Citation82,Citation84,Citation86,Citation87]. With longer duration of disease, hyaline membranes are organized into granulation tissue and there is both interstitial and alveolar fibrosis [Citation81,Citation86,Citation87]. The airways are less severely affected and may have loss of cilia, denuded epithelium, mild lymphocytic inflammation, and squamous metaplasia [Citation28,Citation81,Citation82,Citation86,Citation87]. Multinucleated syncytial cells of epithelial or macrophage origin are found regardless of the aetiology and most likely reflect viral cytopathic change [Citation28,Citation29,Citation81,Citation86,Citation87]. There are additional reports of acute fibrinous and organizing pneumonia that have a characteristic pattern of fibrin balls within the alveoli rather than membranes in addition to organizing fibrosis [Citation83,Citation88]. Secondary bacterial or fungal infections causing acute necrotizing pneumonia may obscure the underlying interstitial disease. Viral nucleic acids, viral particles, and/or proteins are detected predominantly in type I and II pneumocytes but can also be found in alveolar macrophages, endothelial cells, and the bronchiolar epithelium [Citation28,Citation82,Citation87].
Vascular injury is an essential underpinning of the pathogenesis of ARDS; as such, fibrin and platelet thrombi are frequently identified in patients with coronavirus-related pneumonia [Citation81–83,Citation85,Citation86]. Pulmonary thromboemboli [Citation81,Citation83] and infarction [Citation85,Citation86] are less frequent findings. While vascular injury is not unique to coronavirus-related ARDS, some studies have proposed that thrombosis, endothelial injury, and capillary proliferations are more severe in COVID patients than in patients with non-COVID ARDS [Citation88,Citation89]. Ackermann et al. [Citation88] found that thrombi in alveolar capillaries and capillary proliferations were 9 times and 2.7 times as common, respectively, in COVID-19 patients compared to influenza patients with ARDS. Bryce et al. [Citation81] also noted an increase in capillary thrombi in COVID patients compared to non-COVID DAD whereas Konopka et al. [Citation90] did not find significant differences between COVID and non-COVID DAD. Larger case-controlled studies may help to clarify these discrepancies. Microthrombi and vascular compromise have been identified in the brain [Citation81,Citation91], intestine [Citation92], kidney [Citation93,Citation94], and skin [Citation95] in COVID patients, further supporting a key role for coagulopathy and thrombosis in disease pathogenesis.
Animal models of COVID-19
Mouse models
Owing to differences in orthologous receptor genes, human coronaviruses either do not replicate in laboratory mice (Mus musculus) in the case of MERS-CoV [Citation96] and ancestral strains of SARS-CoV-2 [Citation97,Citation98] or replicate transiently but do not produce disease in the case of SARS-CoV [Citation99]. Strategies to overcome this obstacle include development of mice transgenic for human ACE2 [Citation100–103], the receptor for SARS-CoV and SARS-CoV-2, and for DPP4 [Citation104], the receptor for MERS-CoV; generation of mouse-adapted viruses [Citation105–108]; and using adenovirus or adeno-associated virus (AAV) vectors to transduce transient expression of hACE2 [Citation98,Citation109,Citation110]. As SARS-CoV-2 expanded across the globe, new and highly transmissible variants arose that harboured, among others, mutations in the RBD of the spike protein (reviewed in ref [Citation111]). These mutations allow for increased binding affinity to murine Ace2 [Citation112] and efficient replication in the lungs of wild-type laboratory mice leading to pneumonia [Citation112,Citation113]. Thus, wild-type laboratory mice like C57BL/6 and BALB/c can be used to study new variants, although other models are still more popular.
Mice expressing hACE2 under the general human epithelial promoter cytokeratin 18 (Krt18) (K18-hACE2) were developed by McCray et al. [Citation100] to model lethal SARS-CoV infection. Mice had detectable hACE2 mRNA in the lung, colon, liver, and kidney and low levels in the brain with a similar distribution to the endogenous murine Ace2 gene. Mice inoculated intranasally with 2.3 × 104 plaque forming units (PFU) of SARS-CoV developed weight loss, lethargy, and laboured breathing by 3–5 days post infection (dpi) and died by 7 dpi. Infectious virus was detected only in the lungs and brain. Histologically, there were perivascular and peribronchiolar infiltrates of mononuclear cells, alveolar thickening, and haemorrhage. Compared to wild-type C57BL/6 mice, K18-hACE2 mice had more severe and prolonged pulmonary inflammation but did not exhibit DAD that is seen in COVID-19 patients. Virus was detected using immunohistochemistry in neurons throughout the brain, but notably was absent in the olfactory bulb, suggesting that the virus does not enter the brain via olfactory neurons but rather via systemic spread. SARS-CoV has been detected in the central nervous system in fatal human cases [Citation114,Citation115] but it is not considered a primary site of infection or cause of death. The authors of the study [Citation100] concluded that CNS infection likely contributes to the fatal outcome in K18-hACE2 mice. Two other transgenic hACE2 models were developed around this time using the murine Ace2 promoter [Citation116] and a composite universal chicken b-actin and cytomegalovirus promoter [Citation117]. Viral distribution in the lungs and brain were similar for all three mouse models, although infection of transgenic hACE2 mice under the endogenous mouse promoter was not lethal [Citation116].
At the start of the COVID-19 pandemic, the K18-hACE2 mouse was investigated as a model for severe SARS-CoV-2 infection by several groups [Citation101,Citation102,Citation118–120]. Moreau et al. [Citation118] infected mice intranasally with 8 × 104 TCID50 SARS-CoV-2 strain Hong Kong/VM20001061/2020 and showed mild weight loss and varying clinical signs. Virus was detected in the respiratory tract, but other organs were not assessed. Histologically, mice had mild alveolar thickening with neutrophils and proteinaceous debris in alveolar spaces. Using a similar infectious dose of SARS-CoV-2 strain USA-WA1/2020, Rathnasinghe et al. [Citation119] showed progressive weight loss in K18-hACE2 mice with a single animal dying on 8 dpi. The predominant histologic finding in these mice was interstitial pneumonia with no lesions in the brain. The virus was detected in nasal turbinates and lungs at high titres on 2 dpi, which decreased by 5 dpi. In contrast, viral titres in the brain were low on 2 dpi and increased by 5 dpi. A similar trend of increasing viral titres in the brain as lung titres decrease was shown in other studies in K18-hACE2 mice [Citation101,Citation102,Citation121]. This suggests that virus reaches the brain after establishing infection in the lung, possibly through a leaky blood–brain barrier. In support of this hypothesis, viral antigen is present in neurons throughout the brain [Citation101,Citation119,Citation120] rather than being primarily confined to the olfactory bulb, as would be expected if the virus were introduced during intranasal inoculation. It is also possible that a combination of systemic spread and local invasion from the nasal cavity contribute to brain infection. Regardless, oedema, cerebral microthrombi, and lymphocytic meningoencephalitis of varying severity [Citation101,Citation102,Citation120–122] are features of this model that are not typically seen in COVID patients [Citation81].
At higher doses, infection of K18-hACE2 mice with SARS-CoV-2 is lethal by 5–7 dpi. Histologic findings in the lung at high doses include largely mononuclear interstitial inflammation, alveolar histiocytosis, intra-alveolar oedema and fibrin accumulation, type II pneumocyte hyperplasia, occasional vasculitis, and rare syncytial cells [Citation101,Citation102,Citation120]. Examples of histologic findings are shown in . These findings are consistent with many features of DAD in COVID-19 patients with the notable exception of hyaline membranes. Viral antigen can be demonstrated in type I and II pneumocytes, macrophages, and neurons throughout the brain [Citation101,Citation119,Citation120]. The pulmonary distribution is comparable to people, but virus is usually absent [Citation123] or only detected in single cells of the brainstem and in cranial nerves [Citation91] of human patients. While neurologic symptoms do occur in COVID-19 patients, neuropathology is more likely due to hypoxic changes or neuroimmune activation rather than direct damage caused by viral infection [Citation91,Citation123]. In this regard, because K18-hACE2 mice frequently develop viral encephalitis, this model may not accurately reflect disease pathogenesis.
Figure 2. Lung pathology in mice infected with SARS-CoV-2. a-c K18-hACE2 mouse infected with 5 × 104 pfu SARS-CoV-2 WA10 that died on day 7. At low magnification (a), approximately 70% of the lung is hypercellular and there are multiple discrete foci of inflammation (arrows). The normal pulmonary architecture is obscured due to haemorrhage (arrow), oedema (stars), and inflammation (b). The alveolar and bronchiolar exudate is largely composed of neutrophils and there is rare type II pneumocyte hyperplasia (arrow) (c). (d-f) 6-week-old BALB/c mouse infected with 1 × 104 pfu SARS-CoV-2 MA10 that was euthanized on day 4. There is minimal change at low magnification (d). There are occasional areas of hypercellularity (e) where septa are mildly thickened due to congestion and increased lymphocytes and macrophages (f).
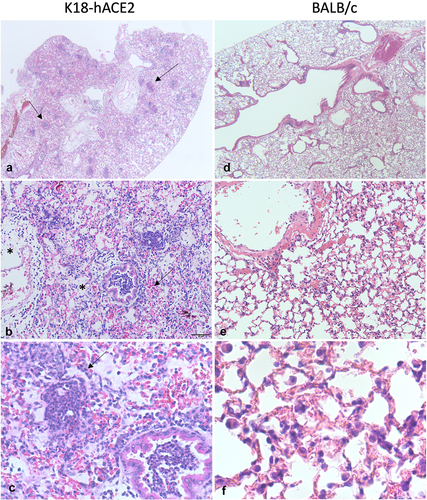
Like people, SARS-CoV-2 infection in K18-hACE2 mice also causes anosmia. Using a series of behavioural tests, Zheng et al. [Citation120] showed that anosmia occurred in K18-hACE2 mice prior to weight loss and brain infection, indicating that altered smell was not due to central nervous system dysfunction. Antigen was detected in the olfactory epithelium, including sustentacular cells. Sustentacular cells express high levels of ACE2 [Citation124] and are the main target of infection in the olfactory epithelium [Citation57,Citation125]. Loss of smell is accompanied by focal areas of degeneration and necrosis of the olfactory epithelium [Citation126], implicating direct epithelial damage in the pathogenesis of anosmia.
Several additional mouse models have been generated that enable expression of hACE2 under the control of other promoters. Mice transgenic for hACE2 with expression driven by the endogenous mAce2 promoter developed typical mild-to-moderate interstitial pneumonia with occasional bronchiolar epithelial degeneration and sloughing. Notably, virus was not detected in the brain of these mice [Citation97]. Infected C3B6 mice transgenic for hACE under the lung ciliated epithelium-specific HFH4/FOXJ1 promoter [Citation107,Citation127,Citation128] were either asymptomatic or displayed varying degrees of weight loss, dyspnoea, and neurologic signs prior to death [Citation128]. Correspondingly, interstitial pneumonia varied from mild to severe with hyaline membranes and fibrin thrombi. Virus was detected in the brains only of mice that died, suggesting encephalitis plays a major role in mortality [Citation128]. In another strategy, Sun et al. [Citation103] used CRISPR/Cas9 to knock in the hACE2 gene, replacing mAce2, while maintaining control under the endogenous mAce2 promoter. A benefit of this humanized model is that targeted gene insertion has the potential for more physiologic gene expression. Mice exhibited no clinical signs and did not die, although older mice lost approximately 10% of their starting weight by 3 dpi. Lung lesions were mild and included interstitial pneumonia and focal haemorrhage. High levels of viral mRNA and antigen were also detected in the brain, but histologic analysis of the brain was not reported, so the significance of brain infection is unclear.
To better replicate human ACE2 expression dynamics in mice, Zhang et al. [Citation129] recently reported a novel genome writing method to generate humanized ACE2 mice. These mice express the regulatory components and splice isoforms of human ACE2 at levels that mimic expression in people, so are potentially a more physiologically relevant mouse model of COVID-19. Following intranasal challenge with 105 PFU SARS-CoV-2, viral RNA and infectious virus could only be detected in the lungs but not in other organs of ACE2 mice. Mice experienced no clinical signs of disease and had mild interstitial pneumonia at 3 dpi.
One drawback to transgenic and humanized mouse models is that their use is limited to the background mouse strain. There are currently two ways to overcome this: by infecting with a virus adapted to bind to the murine Ace2 receptor or by transducing mice using a viral vector that allows for transient expression of hACE2. Mouse adapted viruses have been developed to model SARS-CoV [Citation105], SARS-CoV-2 [Citation106,Citation107], and MERS-CoV [Citation108]. Dinnon et al. [Citation107] used reverse genetics to engineer specific mutations into the SARS-CoV-2 S gene that were predicted to increase binding between S and mAce2. Young BALB/c mice infected with this virus (SARS CoV-2 MA) supported viral replication in the upper and lower respiratory tract and had mild-to-moderate interstitial pneumonia without clinical signs or weight loss. Aged BALB/c mice experienced transient weight loss, delayed viral clearance, and increased inflammation, haemorrhage, and damage to bronchiolar epithelium. To build upon this model, Leist et al. [Citation106] serially passaged SARS-CoV-2 MA through BALB/c mouse lungs to obtain the more pathogenic SARS-CoV-2 MA10. Using this new virus, young BALB/c mice experienced dose dependent weight loss and mortality. Histologic lesions were more severe when compared to SARS-CoV-2 MA and included bronchial epithelial necrosis, increased alveolar septal inflammation, pneumocyte necrosis, alveolar oedema and fibrin accumulation, and occasional hyaline membranes. Old BALB/c mice (1 year old) infected with SARS-CoV-2 MA10 had about 100% mortality at high doses and higher pathology scores for acute lung injury. Example histologic lesions in a young (6-week-old) BALB/c mouse infected with SARS-CoV-2 MA10 is shown in . Disease is less severe in C57BL/6 mice, demonstrating that differences in host genetics can alter the outcome of infection.
Similarly, transduction of hACE2 expression using non-replicating viral vectors [Citation109,Citation110,Citation119] is a technique that can be applied to multiple mouse strains. Advantages of this strategy include the use of wildtype virus and cost-efficiency compared to generating transgenic mice. At early time points, pulmonary inflammation in SARS-CoV-2 infected mice is mild and mainly peribronchial to perivascular, which is similar to inflammation induced by the viral vector alone [Citation109,Citation110]. Pneumonia is more severe at later time points, however, consisting of intra-alveolar neutrophils and fibrin with congestion [Citation110]. Ectopic expression of hACE2 may be a confounding factor, however, as demonstrated by Rai et al. [Citation130] In this study, diet-induced obese and lean C57BL/6N mice that were transduced with AdV-hACE2 experienced weight loss irrespective of SARS-CoV-2 infection, indicating likely off-target effects by the viral vector.
As previously mentioned, transgenic and wild-type laboratory mice support replication of new SARS-CoV-2 variants. One of the earliest recognized virus variants harboured a D614G mutation in the spike protein that was associated with increased infectivity [Citation131,Citation132]. Infection of hACE2 transgenic [Citation133] and knock-in [Citation134] mice with this variant resulted in high viral loads in the respiratory tract [Citation133,Citation134] and lung pathology that was similar to mice infected with wild-type virus [Citation133]. The subsequent variants Alpha (B.1.1.7), Beta (B.1.351), Gamma (P.1), and Omicron (B.1.1.529) bear the spike protein mutation N501Y that is associated with mouse adaptation and allows infection of wild-type mice [Citation135,Citation136]. These variants replicate efficiently in the upper and lower respiratory tracts of C57BL/6, BALB/c, 129, and hACE2 transgenic mice [Citation112,Citation113,Citation137–139] and, notably, do not appear to infect the brain of wild-type mice [Citation112,Citation139]. Omicron sublineages are currently the dominant circulating strains of SARS-CoV-2 [Citation140] these infections result in generally milder symptoms with fewer hospitalizations, ICU admissions, and deaths [Citation141]. Similarly, mice infected with Omicron variants have less weight loss, lower viral loads in the upper and lower respiratory tract, and decreased levels of inflammatory cytokines compared to ancestral strains and previous variants [Citation138,Citation142]. The pathology in Omicron-infected mice is overall milder with less inflammation and epithelial damage and fewer antigen-positive cells in the respiratory tract [Citation142,Citation143].
In summary, mouse models of COVID-19 span the spectrum of disease from mild to severe, some with features of diffuse alveolar damage [Citation101,Citation102,Citation120] and age-related increases in severity [Citation103,Citation106] that are observed in COVID-19 patients. These mice have been indispensable in advancing the rapid development of novel vaccines [Citation144–146] and therapeutics [Citation107,Citation147] to combat the COVID-19 pandemic. K18-hACE2 mice are the most frequently used model and the K18-hACE2 transgene has been introduced to multiple well-characterized mouse strains to expand their use [Citation148]. With the advent of new genomic techniques, the mouse model of COVID-19 continues to evolve to better replicate the human disease.
Ferret Model
Ferrets (Mustela putorius furo) are susceptible to experimental infection intranasally or intratracheally with SARS-CoV [Citation149,Citation150] and SARS-CoV-2 [Citation151] due to favourable interactions between ferret ACE2 and the RBD of the spike protein [Citation152]. They do not, however, support replication of MERS-CoV, likely due to differences in ferret DPP4 leading to poor virus binding [Citation153].
Clinical signs are absent or mild in ferrets infected with SARS-CoV and SARS-CoV-2, including variants of concern like Delta (B.1.617.2) [Citation154,Citation155] and Omicron [Citation155], and may include varying degrees of sneezing, cough, and reduced activity [Citation150,Citation151,Citation156,Citation157]. Infected ferrets may also have mildly increased body temperatures but maintain stable weight [Citation150,Citation151]. Lung lesions due to SARS-CoV-2 are less severe than SARS-CoV [Citation149,Citation157] and may be absent [Citation154]. When present, they consist of acute, multifocal bronchiolitis and/or peribronchiolitis with intraluminal accumulations of necrotic debris and neutrophils and less frequent mild lymphohistiocytic interstitial pneumonia with occasional type II pneumocyte hyperplasia [Citation151,Citation156–158]. An interesting feature in ferrets is varying degrees of bronchus-associated lymphoid tissue (BALT) hyperplasia that increases with time and may lead to bronchiolar restriction [Citation156,Citation157], especially in young ferrets inoculated intranasally [Citation156]. Pathology in the nasal cavity ranges from mild, consisting of occasional epithelial cell loss and infiltration of neutrophils [Citation157], to severe with mucosal thickening due to hyperplasia, squamous metaplasia, and neutrophilic and lymphohistiocytic rhinitis. An exudate of similar inflammation mixed with mucus is present in severe cases [Citation156]. Similar findings may be present in the trachea [Citation156]. High loads of viral RNA and infectious virus can be isolated from the upper respiratory tract and low to sometimes undetectable amounts in the lung [Citation149–151,Citation156,Citation158]. Similarly, Ryan et al. [Citation157] used in situ hybridization to show that large amounts of viral RNA was present in the epithelial cells and sustentacular cells of the nasal mucosa but only occasional type I and II pneumocytes and alveolar macrophages were positive in the lung. Viral RNA can also be detected in the intestine and brain, but infectious virus is generally not present in these extrapulmonary sites [Citation151,Citation156]. Thus, SARS-CoV-2 is largely an upper respiratory tract infection in ferrets. An important feature of the ferret model is the ability to shed and transmit both ancestral SARS-CoV-2 strains and variants of concern to uninfected contact and non-contact animals [Citation151,Citation154,Citation159]. This aligns with the consistent detection of viral RNA and infectious virus from nasal and oral fluids [Citation151,Citation156–158].
Overall, the ferret is a mild infection model for COVID-19. Pathology is largely restricted to the upper respiratory tract and the conducting airways of the lung (bronchi and bronchioles), although there is occasional involvement of alveoli as the disease progresses. Ferrets are a naturally susceptible small animal model that mimics mild, mainly upper respiratory tract disease and transmission in people. Because of these features, ferrets are an ideal model for testing mucosal vaccines and therapeutics [Citation160–163].
Hamster Model
Originally defined as a model for SARS-CoV infection [Citation164], Syrian or golden hamsters (Mesocricetus auratus) are a popular alternative model for COVID-19 research. Like ferrets, they are naturally susceptible to infection with SARS-CoV [Citation164] and SARS-CoV-2 [Citation152,Citation165–167]. Four other hamster species have been investigated with varying results: Roborovski dwarf hamsters (Phodopsus roborovskii) [Citation168,Citation169], Chinese hamsters (Cricetulus griseus) [Citation170], Cambell’s dwarf hamsters (P. campbelli) [Citation168], and Djungarian dwarf hamsters (P. sungorus) [Citation168]. Chinese hamsters support replication of SARS-CoV-2 in the upper and lower respiratory tract and develop weight loss, suppurative bronchitis, and pneumonia with a disease course that is milder but more prolonged compared to Syrian hamsters [Citation170]. Campbell’s and Djungarian dwarf hamsters develop disease that is similar or milder than Chinese and Syrian hamsters [Citation168]. In contrast, infection in Roborovski dwarf hamsters yields fulminant and often lethal disease [Citation168,Citation169]. A detailed review of the pathologic findings in hamster models of COVID-19 was recently published [Citation171], so findings in only the two more popular models, the Syrian and Roborovski dwarf hamsters, will be described here.
In Syrian hamsters, clinical signs including lethargy, hunching, ruffled fur, and increased respiratory rate begin at 2 dpi and peak around 5–6 dpi before beginning to resolve [Citation165,Citation167]. Similarly, animals gradually lose weight with a maximum of approximately 10% weight loss around day 6, followed by recovery [Citation165,Citation167,Citation172]. The earliest lesions appear in the nasal turbinates, where loss of cilia [Citation172] and epithelial cell necrosis and loss is accompanied by an influx of mononuclear cells at 2–4 dpi [Citation165,Citation173]. Viral antigen is present in the nasal epithelium, including olfactory sensory neurons and sustentacular cells of the olfactory mucosa [Citation172,Citation173]. Like people, Syrian hamsters experience ageusia and anosmia that spontaneously resolves by 14 dpi [Citation172]. Outside of the upper respiratory tract, virus is detected in the lungs, brain, and intestines [Citation165,Citation166,Citation173]. Lesions are largely restricted to the nasal mucosa and lungs, although myocardial degeneration [Citation165,Citation174], intestinal epithelial necrosis [Citation165], and adrenal inflammation [Citation174] have been described, especially at higher doses [Citation174]. Early pulmonary lesions consist of suppurative bronchiolitis and bronchitis starting at 2 dpi [Citation171]. As the pneumonia progresses, alveolar spaces are flooded with protein-rich oedema, fibrin, neutrophils, and macrophages while the interstitium is expanded by mononuclear cells and oedema [Citation165–167]. Syncytial cells are found in both bronchioles and alveoli [Citation165,Citation167,Citation171].
Resolution begins by 7 dpi when the predominant lesion is interstitial pneumonia with evidence of repair, including bronchiolar epithelial hyperplasia, type II pneumocyte hyperplasia, and mild septal fibrosis [Citation165,Citation167,Citation171]. Viral antigen is present in the bronchiolar epithelium, type I and II pneumocytes, and alveolar macrophages early in the infection and is undetectable by 7–10 dpi [Citation165,Citation167,Citation173,Citation175]. A notable feature of the Syrian hamster model is a vascular lesion characterized by subendothelial accumulations of lymphocytes and neutrophils [Citation167,Citation175] consistent with the endothelialitis described in human patients [Citation88,Citation176]. However, microvascular thrombosis has not been demonstrated in hamsters, nor is the virus detected in the endothelium [Citation175]. Syrian hamsters are also a good model for sex- and age-dependent disease severity as male animals experience greater weight loss and pneumonia [Citation177] and aged animals have greater weight loss, weaker immune responses, and prolonged pulmonary inflammation [Citation175,Citation178].
Syrian hamsters have been used to evaluate the pathogenicity and transmissibility of SARS-CoV-2 variants of concern, including Delta, Omicron, and dominant Omicron sublineages [Citation138,Citation179–183]. In concordance with the milder symptoms experienced by people infected with Omicron strains [Citation141], Omicron-infected Syrian hamsters experience lower clinical scores, less weight loss, and milder interstitial pulmonary inflammation with fewer antigen-positive epithelial cells when compared to hamsters infected with ancestral [Citation138,Citation184] and Delta strains [Citation138,Citation179–181,Citation184]. Yuan et al. [Citation180] showed that despite Omicron’s decreased pathogenicity and lower viral loads, transmission rates were often higher than Delta in both contact and non-contact animals, suggesting other factors may contribute to the high transmissibility of Omicron variants.
At the standard dose of 1 × 105 pfu of SARS-CoV-2, Roborovski dwarf hamsters rapidly develop clinical signs including dyspnoea, snuffling, sneezing, hunched posture, weight loss, decreased activity, and fever followed by hypothermia [Citation168,Citation169]. Animals either die or meet euthanasia criteria by 3–4 dpi. As early as 2 dpi, infected animals have evidence of diffuse alveolar damage, including necrosis of alveolar epithelium, hyaline membranes, oedema, haemorrhage, and necrotic debris within alveolar spaces [Citation168,Citation169]. Zhai et al. [Citation169] also documented a right lung lobe predominance, which is a feature of human disease [Citation185]. There is evidence of coagulation abnormalities, including elevated D-dimers and fibrin degradation products [Citation169] and hyaline microthrombi in alveolar septal capillaries [Citation168] although, unlike Syrian hamsters, endothelialitis is absent [Citation168]. Virus is detected in type II pneumocytes, bronchial and bronchiolar epithelial cells, and macrophages [Citation168,Citation169]. The virus appears to spread systemically, as low levels of viral RNA are detected in whole blood, the gastrointestinal tract, kidney, liver, and brain [Citation168,Citation169]. There are discrepancies on whether the brain is involved, as Trimpert et al. [Citation168] described no histologic lesions or viral RNA by in situ hybridization, whereas Zhai et al. [Citation169] showed mild subarachnoid haemorrhage and inflammation with occasional cells positive by IHC. However, infectious virus was not quantified in the brain, so whether these lesions are a direct consequence of neuroinvasion remains unanswered. Interestingly, Trimpert et al. [Citation168] also showed dose dependency where low doses of 5 × 104 pfu resulted in disease similar to Syrian hamsters consisting of suppurative pneumonia and bronchitis with occasional endothelialitis.
Hamsters are naturally susceptible to SARS-CoV-2 and are a convenient small animal model of COVID-19. Syrian hamsters experience mild to moderate, non-lethal disease and exhibit certain features of the human disease, such as age-dependent disease severity [Citation175,Citation178], pulmonary endotheliitis [Citation167,Citation175], and anosmia and ageusia [Citation172]. They are also useful for transmission studies [Citation165,Citation173,Citation180] and to evaluate the pathogenic potential of SARS-CoV-2 variants [Citation179–183]. Roborovski dwarf hamsters can reliably model both moderate and severe disease with several features of human disease, including fever, diffuse alveolar damage, hyaline membranes, and microthrombi [Citation168,Citation169]. To date, this is the best model of severe disease and additional work to characterize transmission in this species is needed. Other advantages include their small size and overall friendly disposition when compared to other hamster species [Citation168]. The full genome of P. roborovski was recently characterized [Citation186] and an inbred strain (SH101) is available [Citation169]. Despite these advantages, use of Roborovski dwarf hamsters will likely still be limited due to lack of reagents and molecular tools.
Non-human Primate models
Non-human primates (NHPs) are susceptible to infection with human coronaviruses and a variety of NHP species have been used. Clinical illness and pathology are mild in macaque (Macaca spp.) models of SARS [Citation187–191] and MERS [Citation192,Citation193]. Infection with SARS-CoV-2 has been described in rhesus (Macaca mulatta) [Citation194–200] and cynomolgus (Macaca fascicularis) [Citation193,Citation194,Citation199] macaques, African green monkeys (Chlorocebus sebaeus) [Citation199,Citation201–203], common marmosets [Citation197], and baboons (Papio spp.) [Citation197].
Like NHPs infected with SARS-CoV, infection with SARS-CoV-2 generally results in mild to moderate clinical disease and resolution within 2 weeks [Citation193–197,Citation199,Citation200,Citation202,Citation203]. Rhesus and cynomolgus macaques are the most common NHPs used for research [Citation204,Citation205] and have been extensively studied as models for COVID-19. Routes of infection for NHPs include intratracheal, intranasal, oral, and conjunctival, with several studies using multiroute inoculation [Citation193,Citation194,Citation196,Citation197,Citation200]. High viral loads can be detected in the respiratory tract and oral cavity regardless of the inoculating dose and route [Citation199–201]. One benefit of NHPs is that various types of imaging can be used to evaluate disease, including chest radiographs, computed tomography (CT), and positron emission tomography (PET) scans [Citation194,Citation195,Citation197,Citation203]. NHPs have detectable interstitial pneumonia and ground glass opacities using these modalities, which provides a relevant antemortem readout for assessing infection and response to therapeutics and vaccines. Clinical signs are generally absent or mild and may include decreased appetite, piloerection, hunching, and changes in respiratory rate and/or effort [Citation196,Citation200,Citation202]. Mild weight loss is possible and increased body temperature is typically transient [Citation194–196]. Viral RNA shedding peaks at 2–3 dpi in nasopharyngeal and oropharyngeal swabs and bronchoalveolar lavage fluid and is low to undetectable by 14–28 days [Citation194,Citation197,Citation199,Citation200], indicating viral clearance. Similar to human patients [Citation206], rectal shedding also occurs in African green monkeys [Citation201,Citation202], baboons [Citation197], and cynomolgus macaques [Citation193].
Postmortem lesions include foci of dark red pulmonary consolidation of varying severity, pulmonary oedema, and swollen lymph nodes [Citation196,Citation197,Citation201]. Histologically, areas of consolidation at early time points correspond with thickened alveolar septa due to accumulations of macrophages, lymphocytes, neutrophils, oedema, and fibrin. This interstitial pneumonia is centred on terminal bronchioles and, in more severe cases, is associated with flooding of alveoli with protein-rich oedema fluid, haemorrhage, fibrin, macrophages and neutrophils. Damaged alveoli are occasionally lined by hyaline membranes and hyperplastic type II pneumocytes with minimal septal fibrosis. There is multifocal bronchiolar epithelial necrosis with associated submucosal macrophages, neutrophils, and eosinophils [Citation196]. Perivascular spaces contain lymphocytes and plasma cells and, like in ferrets, BALT tissue is often hyperplastic. Alveoli and bronchioles occasionally contain syncytial cells that stain positively with pancytokeratin [Citation202] indicating their epithelial origin. The pneumonia typically resolves by 2–3 weeks [Citation197]. SARS-CoV-2 antigen can be detected in type I and II pneumocytes, ciliated epithelium, and alveolar macrophages [Citation193,Citation196,Citation202]. Antigen is not present in the brain or intestine [Citation193,Citation196]. Older NHPs have worse interstitial pneumonia and prolonged viral shedding [Citation193,Citation194,Citation197,Citation198], although age does not typically affect disease outcome [Citation193]. Severe disease has only been shown in one study of aged African green monkeys where 2 of 4 infected animals rapidly deteriorated and had radiographic and histologic features of ARDS [Citation201]. Taken together, the clinical and pathologic features of SARS-CoV-2 infection in NHPs reflects non-lethal, mild-to-moderate COVID-19 in people.
NHPs are susceptible to infection with several strains of SARS-CoV-2 and have been used to evaluate their pathogenicity [Citation207–209] and to test the efficacy of vaccine candidates against variants of concern [Citation210–212]. Like mice and hamsters, infection of macaques with the currently circulating (dominant) Omicron subvariants yields less severe clinical disease compared to Delta variants [Citation208]. While the features of pneumonia described previously can be observed in Omicron-infected macaques, the lesions are milder and associated with lower titres of infectious virus [Citation208], which is consistent with the milder clinical presentation of these infections in people [Citation141].
Some differences have been described between NHP species, although the low number of animals used in these studies makes drawing definitive conclusions difficult. Weight loss, changes in body temperature, and presence of clinical signs are variable across studies within the same species of NHP, likely reflecting differences in virus strain, dose, route of infection, and animal monitoring. Using a multiroute mucosal infection, Lu et al. [Citation194] compared species of NHP and found that rhesus macaques had increased pulmonary infiltrates on chest radiographs compared to cynomolgus macaques although both experienced weight loss and increased body temperature. Johnston et al. [Citation199] used an aerosol infection model in rhesus macaques, cynomolgus macaques, and African green monkeys (AGM). Cynomolgus macaques had higher clinical disease scores than both rhesus macaques and AGMs and were the only species to develop a fever. The aerosol model elicited disease similar to mild COVID-19 and, in cynomolgus macaques, appears to result in more clinically relevant disease compared to multiroute mucosal inoculation [Citation193,Citation194]. Common marmosets, a species of new world monkey, show no clinical signs, shed less virus, and clear the infection faster than old world monkeys [Citation194,Citation197]. Baboons have been less commonly studied, although Singh et al. [Citation197] found that they had more severe and persistent pneumonia than macaques. It has been suggested that baboons should be considered as an alternative to macaques to lessen the burden on macaque supply for other types of biomedical research [Citation213].
Like other naturally susceptible laboratory animal species, NHPs infected with pathogenic human coronaviruses generally develop mild-to-moderate disease. Disease is worse in baboons [Citation197] and aged African green monkeys [Citation201] so these species may be better suited for investigating severe COVID-19. Although they are costly and have strigent husbandry requirements, NHPs have proven an invaluable resource for the discovery of novel vaccines and therapeutics during the COVID-19 pandemic [Citation144–146,Citation214,Citation215].
Conclusions
This review describes the features of commonly used animal models of COVID-19, including mice, ferrets, hamsters, and NHPs with a particular focus on pathology. Their main features and uses are highlighted in . Mice are the most frequently used model as they are cost efficient, genetically malleable, and immunologic tools and reagents are abundantly available. However, mice are not susceptible to ancestral strains of SARS-CoV-2, so several strategies were used to overcome this limitation, including infection with mouse-adapted virus [Citation105–108], transient induction of hACE2 expression using viral vectors [Citation98,Citation109,Citation110], and development of transgenic and humanized hACE2 mice [Citation100–103]. Wildtype laboratory mice are susceptible to new, highly transmissible SARS-CoV-2 variants of concern [Citation112,Citation113], although their use in developing new vaccines and therapeutics for these variants has been limited thus far. As infection is predominantly upper respiratory and they display clinical signs like coughing and sneezing, ferrets play a unique role in studying transmission [Citation149,Citation151] and may be a good model for mild or asymptomatic infection [Citation243]. Syrian hamsters also efficiently transmit SARS-CoV-2 and develop more severe, although non-lethal, pneumonia compared to ferrets [Citation165,Citation173,Citation180]. The Roborovski dwarf hamster is probably the best model of severe disease, although their use is limited by lack of available laboratory tools and reagents. Finally, owing to their close genetic relationship with humans, NHPs are a popular model for testing vaccines and therapeutics despite having an overall mild phenotype.
Table 1. Summary of animal models of COVID-19.
The animal models described above have been developed at a rapid pace, largely due to work done during previous coronavirus outbreaks. They have been instrumental in developing life-saving vaccines and therapeutics, although knowledge gaps remain. Hematologic and coagulation abnormalities that occur in human disease have been documented in only a few of the animal models available thus far [Citation122,Citation169,Citation196,Citation202,Citation244], although these readouts are generally not included in most studies. Likewise, there are other comorbid factors that contribute to disease severity, such as hypertension and chronic pulmonary disease, that have yet to be adequately reproduced in animal models. Finally, an animal model that can recreate the myriad symptoms of long COVID is greatly needed to address the underlying mechanisms and to discover novel treatment modalities.
Acknowledgements
We would like to thank the anonymous reviewers and the journal editor for their suggestions that improved the overall quality of this manuscript.
Disclosure statement
No potential conflict of interest was reported by the author(s).
Data Availability statement
Data reported in the figure and table in this article have been deposited in a recognized data repository (Figshare.com) with a digital object identifier: 10.6084/m9.figshare.24348958
Additional information
Funding
References
- Bukhari K, Mulley G, Gulyaeva AA, et al. Description and initial characterization of metatranscriptomic nidovirus-like genomes from the proposed new family abyssoviridae, and from a sister group to the coronavirinae, the proposed genus alphaletovirus. Virology. 2018;524:160–22. doi: 10.1016/j.virol.2018.08.010
- Knipe DM, Howley P. Fields virology. United States: Wolters Kluwer Health; 2013.
- Booth TF, Kournikakis B, Bastien N, et al. Detection of airborne severe acute respiratory syndrome (SARS) coronavirus and environmental contamination in SARS outbreak units. J Infect Dis. 2005;191(9):1472–1477. doi: 10.1086/429634
- Kim S-H, Chang SY, Sung M, et al. Extensive viable middle east respiratory syndrome (MERS) coronavirus contamination in air and surrounding environment in MERS Isolation wards. Clin Infect Dis. 2016;63(3):363–369. doi: 10.1093/cid/ciw239
- Santarpia JL, Rivera DN, Herrera VL, et al. Aerosol and surface contamination of SARS-CoV-2 observed in quarantine and isolation care. Sci Rep. 2020;10(1):12732. doi: 10.1038/s41598-020-69286-3
- Zhou J, Li C, Zhao G, et al. Human intestinal tract serves as an alternative infection route for middle east respiratory syndrome coronavirus. Sci Adv. 2017;3(11):eaao4966. doi: 10.1126/sciadv.aao4966
- Jiao L, Li H, Xu J, et al. The gastrointestinal tract is an alternative route for sars-cov-2 infection in a nonhuman primate model. Gastroenterology. 2021;160(5):1647–1661. doi: 10.1053/j.gastro.2020.12.001
- Vlasova AN, Kenney SP, Jung K, et al. Deltacoronavirus evolution and transmission: Current scenario and evolutionary perspectives. Front Vet Sci. 2021;7. doi: 10.3389/fvets.2020.626785
- Malone B, Urakova N, Snijder EJ, et al. Structures and functions of coronavirus replication–transcription complexes and their relevance for SARS-CoV-2 drug design. Nat Rev Mol Cell Biol. 2022;23(1):21–39. doi: 10.1038/s41580-021-00432-z
- Payne S. Family coronaviridae. Viruses. 2017;149–158. doi: 10.1016/B978-0-12-803109-4.00017-9
- Liu X, Zhang X, Lu Z, et al. Potential molecular targets of nonstructural proteins for the development of antiviral drugs against SARS-CoV-2 infection. Biomed Pharmacother. 2021;133:111035. doi:10.1016/j.biopha.2020.111035
- Redondo N, Zaldívar-López S, Garrido JJ, et al. SARS-CoV-2 accessory proteins in viral pathogenesis: knowns and unknowns. Front Immunol. 2021;12. doi: 10.3389/fimmu.2021.708264
- Sparrer MN, Hodges NF, Sherman T, et al. Role of spillover and spillback in SARS-CoV-2 transmission and the importance of one health in understanding the dynamics of the COVID-19 pandemic. J Clin Microbiol. 2023;61(7):e0161022. doi: 10.1128/jcm.01610-22
- Cui J, Li F, Shi Z-L. Origin and evolution of pathogenic coronaviruses. Nat Rev Microbiol. 2019;17(3):181–192. doi: 10.1038/s41579-018-0118-9
- Corman VM, Baldwin HJ, Tateno AF, et al. Evidence for an ancestral association of human coronavirus 229E with bats. J Virol. 2015;89(23):11858–11870. doi: 10.1128/JVI.01755-15
- Tao Y, Shi M, Chommanard C, et al. Surveillance of bat coronaviruses in Kenya identifies relatives of human coronaviruses NL63 and 229E and their recombination history. J Virol. 2017;91(5):e01953–16. doi: 10.1128/JVI.01953-16
- Guan Y, Zheng BJ, He YQ, et al. Isolation and characterization of viruses related to the SARS coronavirus from animals in southern China. Science. 2003;302(5643):276–278. doi: 10.1126/science.1087139
- Tu C, Crameri G, Kong X, et al. Antibodies to SARS coronavirus in civets. Emerg Infect Dis. 2004;10(12):2244–2248. doi: 10.3201/eid1012.040520
- Corman VM, Eckerle I, Memish ZA, et al. Link of a ubiquitous human coronavirus to dromedary camels. Proc Natl Acad Sci U S A. 2016;113(35):9864–9869. doi: 10.1073/pnas.1604472113
- Lam TT-Y, et al. Identifying SARS-CoV-2-related coronaviruses in Malayan pangolins. Nature. 2020;583(7815):282–285. doi: 10.1038/s41586-020-2169-0
- Ellwanger JH, Chies JAB. Zoonotic spillover: understanding basic aspects for better prevention. Genet Mol Biol. 2021;44(1 suppl 1):e20200355. doi: 10.1590/1678-4685-gmb-2020-0355
- Flaxman S, Mishra S, Gandy A, et al. Estimating the effects of non-pharmaceutical interventions on COVID-19 in Europe. Nature. 2020;584(7820):257–261. doi: 10.1038/s41586-020-2405-7
- Watson OJ, Barnsley G, Toor J, et al. Global impact of the first year of COVID-19 vaccination: a mathematical modelling study. Lancet Infect Dis. 2022;22(9):1293–1302. doi: 10.1016/S1473-3099(22)00320-6
- He Y, Lu H, Siddiqui P, et al. Receptor-binding domain of severe acute respiratory syndrome coronavirus spike protein contains multiple conformation-dependent epitopes that induce highly potent neutralizing antibodies. J Immunol. 2005;174(8):4908–4915. doi: 10.4049/jimmunol.174.8.4908
- Ju B, Zhang Q, Ge J, et al. Human neutralizing antibodies elicited by SARS-CoV-2 infection. Nature. 2020;584(7819):115–119. doi: 10.1038/s41586-020-2380-z
- Khoury DS, Cromer D, Reynaldi A, et al. Neutralizing antibody levels are highly predictive of immune protection from symptomatic SARS-CoV-2 infection. Nat Med. 2021;27(7):1205–1211. doi: 10.1038/s41591-021-01377-8
- Toussi SS, Hammond JL, Gerstenberger BS, et al. Therapeutics for COVID-19. Nat Microbiol. 2023;8(5):771–786. doi: 10.1038/s41564-023-01356-4
- Ng DL, Al Hosani F, Keating MK, et al. Clinicopathologic, immunohistochemical, and ultrastructural findings of a fatal case of middle east respiratory syndrome coronavirus infection in the United Arab Emirates, April 2014. Am J Pathol. 2016;186(3):652–658. doi: 10.1016/j.ajpath.2015.10.024
- Alsaad KO, Hajeer AH, Al Balwi M, et al. Histopathology of Middle East respiratory syndrome coronovirus (MERS -CoV) infection – clinicopathological and ultrastructural study. Histopathology. 2018;72(3):516–524. doi: 10.1111/his.13379
- Booth CM, Matukas LM, Tomlinson GA, et al. Clinical features and short-term outcomes of 144 patients with SARS in the greater Toronto area. JAMA. 2003;289(21):2801–2809. doi: 10.1001/jama.289.21.JOC30885
- Zhou P, Yang X-L, Wang X-G, et al. A pneumonia outbreak associated with a new coronavirus of probable bat origin. Nature. 2020;579(7798):270–273. doi: 10.1038/s41586-020-2012-7
- Wang D, Hu B, Hu C, et al. Clinical characteristics of 138 hospitalized patients with 2019 novel coronavirus–infected pneumonia in Wuhan, China. JAMA. 2020;323(11):1061. doi: 10.1001/jama.2020.1585
- Huang C, Wang Y, Li X, et al. Clinical features of patients infected with 2019 novel coronavirus in Wuhan, China. Lancet. 2020;395(10223):497–506. doi: 10.1016/S0140-6736(20)30183-5
- Pan L, Mu M, Yang P, et al. Clinical characteristics of COVID-19 patients with digestive symptoms in Hubei, China: a descriptive, cross-sectional, multicenter study. Am J Gastroenterol. 2020;115(5):766–773. doi: 10.14309/ajg.0000000000000620
- Borsetto D, Hopkins C, Philips V, et al. Self-reported alteration of sense of smell or taste in patients with COVID-19: a systematic review and meta-analysis on 3563 patients. Rhinology. 2020;58:430–436. doi: 10.4193/Rhin20.185
- Davis HE, McCorkell L, Vogel JM, et al. Long COVID: major findings, mechanisms and recommendations. Nat Rev Microbiol. 2023;21(3):133–146. doi: 10.1038/s41579-022-00846-2
- Millet JK, Jaimes JA, Whittaker GR. Molecular diversity of coronavirus host cell entry receptors. FEMS Microbiol Rev. 2021;45(3):fuaa057. doi: 10.1093/femsre/fuaa057
- Li W, Moore MJ, Vasilieva N, et al. Angiotensin-converting enzyme 2 is a functional receptor for the SARS coronavirus. Nature. 2003;426(6965):450–454. doi: 10.1038/nature02145
- Hoffmann M, Kleine-Weber H, Schroeder S, et al. SARS-CoV-2 cell entry depends on ACE2 and TMPRSS2 and is blocked by a clinically proven protease inhibitor. Cell. 2020;181(2):271–280.e8. doi: 10.1016/j.cell.2020.02.052
- Acute respiratory distress syndrome: the berlin definition. JAMA. 2012;307 23. doi: 10.1001/jama.2012.5669
- Assiri A, McGeer A, Perl TM, et al. Hospital outbreak of middle east respiratory syndrome coronavirus. N Engl J Med. 2013;369(5):407–416. doi: 10.1056/NEJMoa1306742
- Clausen TM, Sandoval DR, Spliid CB, et al. SARS-CoV-2 infection depends on cellular heparan sulfate and ACE2. Cell. 2020;183(4):1043–1057.e15. doi: 10.1016/j.cell.2020.09.033
- Hofmann H, Pyrc K, van der Hoek L, et al. Human coronavirus NL63 employs the severe acute respiratory syndrome coronavirus receptor for cellular entry. Proc Natl Acad Sci U S A. 2005;102(22):7988–7993. doi: 10.1073/pnas.0409465102
- Turner AJ. ACE2 cell biology, regulation, and physiological functions. The Protective Arm Of The Renin Angiotensin System (RAS). 2015;185–189. doi: 10.1016/B978-0-12-801364-9.00025-0
- Hamming I, Timens W, Bulthuis M, et al. Tissue distribution of ACE2 protein, the functional receptor for SARS coronavirus. A first step in understanding SARS pathogenesis. J Pathol. 2004;203(2):631–637. doi: 10.1002/path.1570
- Li M-Y, Li L, Zhang Y, et al. Expression of the SARS-CoV-2 cell receptor gene ACE2 in a wide variety of human tissues. Infect Diseases Poverty. 2020;9(1):45. doi: 10.1186/s40249-020-00662-x
- Qi J, Zhou Y, Hua J, et al. The scRNA-seq expression profiling of the receptor ACE2 and the Cellular Protease TMPRSS2 Reveals human organs susceptible to SARS-CoV-2 infection. Int J Environ Res Public Health. 2021;18(1):284. doi: 10.3390/ijerph18010284
- Raj VS, Mou H, Smits SL, et al. Dipeptidyl peptidase 4 is a functional receptor for the emerging human coronavirus-EMC. Nature. 2013;495(7440):251–254. doi: 10.1038/nature12005
- Meyerholz DK, Lambertz AM, McCray PB. Dipeptidyl peptidase 4 distribution in the human respiratory tract: implications for the middle east respiratory syndrome. Am J Pathol. 2016;186(1):78–86. doi: 10.1016/j.ajpath.2015.09.014
- Lamers D, Famulla S, Wronkowitz N, et al. Dipeptidyl peptidase 4 is a novel adipokine potentially linking obesity to the metabolic syndrome. Diabetes. 2011;60(7):1917–1925. doi: 10.2337/db10-1707
- Fukasawa KM, Fukasawa K, Sahara N, et al. Immunohistochemical localization of dipeptidyl aminopeptidase IV in rat kidney, liver, and salivary glands. J Histochem Cytochem. 1981;29(3):337–343. doi: 10.1177/29.3.6787113
- Yeager CL, Ashmun RA, Williams RK, et al. Human aminopeptidase N is a receptor for human coronavirus 229E. Nature. 1992;357(6377):420–422. doi: 10.1038/357420a0
- Li BX, Ge JW, Li YJ. Porcine aminopeptidase N is a functional receptor for the PEDV coronavirus. Virology. 2007;365(1):166–172. doi: 10.1016/j.virol.2007.03.031
- Tresnan DB, Levis R, Holmes KV. Feline aminopeptidase N serves as a receptor for feline, canine, porcine, and human coronaviruses in serogroup I. J Virol. 1996;70(12):8669–8674. doi: 10.1128/jvi.70.12.8669-8674.1996
- Krempl C, Schultze B, Herrler G. Analysis of cellular receptors for human coronavirus OC43. Adv Exp Med Biol. 1995;380:371–374.
- Ahn JH, Kim J, Hong SP, et al. Nasal ciliated cells are primary targets for SARS-CoV-2 replication in the early stage of COVID-19. J Clin Invest. 2021;1485171(13):148517. doi: 10.1172/JCI148517
- Khan M, Yoo S-J, Clijsters M, et al. Visualizing in deceased COVID-19 patients how SARS-CoV-2 attacks the respiratory and olfactory mucosae but spares the olfactory bulb. Cell. 2021;184(24):5932–5949.e15. doi: 10.1016/j.cell.2021.10.027
- Hou YJ, Okuda K, Edwards CE, et al. SARS-CoV-2 reverse genetics reveals a variable infection gradient in the respiratory tract. Cell. 2020;182(2):429–446.e14. doi: 10.1016/j.cell.2020.05.042
- Wolff G, Limpens RWAL, Zevenhoven-Dobbe JC, et al. A molecular pore spans the double membrane of the coronavirus replication organelle. Science. 2020;369(6509):1395–1398. doi: 10.1126/science.abd3629
- Knoops K, Kikkert M, Worm SHEVD, et al. SARS-Coronavirus replication is supported by a reticulovesicular network of modified endoplasmic reticulum. PLoS Biol. 2008;6(9):e226. doi: 10.1371/journal.pbio.0060226
- Menachery VD, Debbink K, Baric RS. Coronavirus non-structural protein 16: evasion, attenuation, and possible treatments. Virus Res. 2014;194:191–199. doi:10.1016/j.virusres.2014.09.009
- Siu K-L, Kok K-H, Ng M-HJ, et al. Severe acute respiratory syndrome coronavirus M protein inhibits type I interferon production by impeding the formation of TRAF3·TANK·TBK1/IKKϵ complex. J Biol Chem. 2009;284(24):16202–16209. doi: 10.1074/jbc.M109.008227
- Thoms M, Buschauer R, Ameismeier M, et al. Structural basis for translational shutdown and immune evasion by the Nsp1 protein of SARS-CoV-2. Science. 2020;369(6508):1249–1255. doi: 10.1126/science.abc8665
- Banerjee AK, Blanco MR, Bruce EA, et al. SARS-CoV-2 Disrupts Splicing, Translation, and Protein Trafficking to Suppress Host Defenses. Cell. 2020;183(5):1325–1339.e21. doi: 10.1016/j.cell.2020.10.004
- Thornbrough JM, Jha BK, Yount B, et al. Middle east respiratory syndrome coronavirus NS4b protein inhibits host RNase L activation. MBio. 2016;7(2):e00258. doi: 10.1128/mBio.00258-16
- Diamond MS, Kanneganti T-D. Innate immunity: the first line of defense against SARS-CoV-2. Nat Immunol. 2022;23(2):165–176. doi: 10.1038/s41590-021-01091-0
- Sievers BL, Cheng MTK, Csiba K, et al. SARS-CoV-2 and innate immunity: the good, the bad, and the “goldilocks”. Cell Mol Immunol. 2023;21(2):171–183. doi: 10.1038/s41423-023-01104-y
- Zhang Q, Bastard P, Liu Z, et al. Inborn errors of type I IFN immunity in patients with life-threatening COVID-19. Science. 2020;370(6515):eabd4570. doi: 10.1126/science.abd4570
- Bastard P, Rosen LB, Zhang Q, et al. Autoantibodies against type I IFNs in patients with life-threatening COVID-19. Science. 2020;370(6515):eabd4585. doi: 10.1126/science.abd4585
- Blanco-Melo D, Nilsson-Payant BE, Liu W-C, et al. Imbalanced Host response to SARS-CoV-2 drives development of COVID-19. Cell. 2020;181(5):1036–1045.e9. doi: 10.1016/j.cell.2020.04.026
- Hadjadj J, Yatim N, Barnabei L, et al. Impaired type I interferon activity and inflammatory responses in severe COVID-19 patients. Science. 2020;369(6504):718–724. doi: 10.1126/science.abc6027
- Zhu L, Yang P, Zhao Y, et al. Single-cell sequencing of peripheral mononuclear cells reveals distinct immune response landscapes of COVID-19 and influenza patients. Immunity. 2020;53(3):685–696.e3. doi: 10.1016/j.immuni.2020.07.009
- Zhou Z, Ren L, Zhang L, et al. Heightened innate immune responses in the respiratory tract of COVID-19 patients. Cell Host Microbe. 2020;27(6):883–890.e2. doi: 10.1016/j.chom.2020.04.017
- Lee JS, Park S, Jeong HW, et al. Immunophenotyping of COVID-19 and influenza highlights the role of type I interferons in development of severe COVID-19. Sci Immunol. 2020;5(49):eabd1554. doi: 10.1126/sciimmunol.abd1554
- Rahmani H, Davoudi-Monfared E, Nourian A, et al. Interferon β-1b in treatment of severe COVID-19: a randomized clinical trial. Int Immunopharmacol. 2020;88:106903. doi: 10.1016/j.intimp.2020.106903
- Repurposed Antiviral Drugs for COVID-19 — Interim WHO Solidarity Trial Results. N Engl J Med NEJMoa, 2023184 (2020) doi:10.1056/NEJMoa2023184.
- Monk PD, Marsden RJ, Tear VJ, et al. Safety and efficacy of inhaled nebulised interferon beta-1a (SNG001) for treatment of SARS-CoV-2 infection: a randomised, double-blind, placebo-controlled, phase 2 trial. Lancet Respir Med. 2021;9(2):196–206. doi: 10.1016/S2213-2600(20)30511-7
- Jhuti D, Rawat A, Guo CM, et al. Interferon treatments for SARS-CoV-2: challenges and opportunities. Infect Dis Ther. 2022;11(3):953–972. doi: 10.1007/s40121-022-00633-9
- Reis G, Moreira Silva EAS, Medeiros Silva DC, et al. Early treatment with pegylated interferon lambda for covid-19. N Engl J Med. 2023;388(6):518–528. doi: 10.1056/NEJMoa2209760
- Interferons. COVID-19 Treatment Guidelines https://www.covid19treatmentguidelines.nih.gov/therapies/antivirals-including-antibody-products/interferons/.
- Bryce C, Grimes Z, Pujadas E, et al. Pathophysiology of SARS-CoV-2: the mount sinai COVID-19 autopsy experience. Mod Pathol. 2021;34(8):1456–1467. doi: 10.1038/s41379-021-00793-y
- Carsana L, Sonzogni A, Nasr A, et al. Pulmonary post-mortem findings in a series of COVID-19 cases from northern Italy: a two-centre descriptive study. Lancet Infect Dis. 2020;20(10):1135–1140. doi: 10.1016/S1473-3099(20)30434-5
- Polak SB, Van Gool IC, Cohen D, et al. A systematic review of pathological findings in COVID-19: a pathophysiological timeline and possible mechanisms of disease progression. Mod Pathol. 2020;33(11):2128–2138. doi: 10.1038/s41379-020-0603-3
- Tse GM-K, To KF, Chan PK, et al. Pulmonary pathological features in coronavirus associated severe acute respiratory syndrome (SARS). J Clin Pathol. 2004;57(3):260–265. doi: 10.1136/jcp.2003.013276
- Hwang DM, Chamberlain DW, Poutanen SM, et al. Pulmonary pathology of severe acute respiratory syndrome in Toronto. Mod Pathol. 2005;18(1):1–10. doi: 10.1038/modpathol.3800247
- Franks TJ, Chong PY, Chui P, et al. Lung pathology of severe acute respiratory syndrome (SARS): a study of 8 autopsy cases from Singapore. Hum Pathol. 2003;34(8):743–748. doi: 10.1016/S0046-8177(03)00367-8
- Gu J, Korteweg C. Pathology and pathogenesis of severe acute respiratory syndrome. Am J Pathol. 2007;170(4):1136–1147. doi: 10.2353/ajpath.2007.061088
- Ackermann M, Verleden SE, Kuehnel M, et al. Pulmonary vascular endothelialitis, thrombosis, and angiogenesis in covid-19. N Engl J Med. 2020;383(2):120–128. doi: 10.1056/NEJMoa2015432
- Miggiolaro AFRS, da Silva FPG, Wiedmer DB, et al. COVID-19 and pulmonary angiogenesis: the possible role of hypoxia and hyperinflammation in the overexpression of proteins involved in alveolar vascular dysfunction. Viruses. 2023;15(3):706. doi: 10.3390/v15030706
- Konopka KE, Nguyen T, Jentzen JM, et al. Diffuse alveolar damage (DAD) resulting from coronavirus disease 2019 infection is morphologically indistinguishable from other causes of DAD. Histopathology. 2020;77(4):570–578. doi: 10.1111/his.14180
- Matschke J, Lütgehetmann M, Hagel C, et al. Neuropathology of patients with COVID-19 in Germany: a post-mortem case series. Lancet Neurol. 2020;19(11):919–929. doi: 10.1016/S1474-4422(20)30308-2
- Xiao F, Tang M, Zheng X, et al. Evidence for gastrointestinal infection of SARS-CoV-2. Gastroenterology. 2020;158(6):1831–1833.e3. doi: 10.1053/j.gastro.2020.02.055
- Diao B, Wang C, Wang R, et al. Human kidney is a target for novel severe acute respiratory syndrome coronavirus 2 infection. Nat Commun. 2021;12(1):2506. doi: 10.1038/s41467-021-22781-1
- Kissling S, Rotman S, Gerber C, et al. Collapsing glomerulopathy in a COVID-19 patient. Kidney Int. 2020;98(1):228–231. doi: 10.1016/j.kint.2020.04.006
- Magro C, Mulvey JJ, Berlin D, et al. Complement associated microvascular injury and thrombosis in the pathogenesis of severe COVID-19 infection: a report of five cases. Transl Res. 2020;220:1–13. doi: 10.1016/j.trsl.2020.04.007
- Scobey T, Yount BL, Sims AC, et al. Reverse genetics with a full-length infectious cDNA of the Middle East respiratory syndrome coronavirus. Proceedings of the National Academy of Sciences. 2013;110:16157–16162.
- Bao L, Deng W, Huang B, et al. The pathogenicity of SARS-CoV-2 in hACE2 transgenic mice. Nature. 2020;583(7818):830–833. doi: 10.1038/s41586-020-2312-y
- Sun J, Zhuang Z, Zheng J, et al. Generation of a broadly useful model for COVID-19 pathogenesis, vaccination, and treatment. Cell. 2020;182(3):734–743.e5. doi: 10.1016/j.cell.2020.06.010
- Glass WG, Subbarao K, Murphy B, et al. Mechanisms of host defense following severe acute respiratory syndrome-coronavirus (SARS-CoV) pulmonary infection of mice. J Immunol. 2004;173(6):4030–4039. doi: 10.4049/jimmunol.173.6.4030
- McCray PB, Pewe L, Wohlford-Lenane C, et al. Lethal infection of K18-hACE2 mice infected with severe acute respiratory syndrome coronavirus. J Virol. 2007;81(2):813–821. doi: 10.1128/JVI.02012-06
- Yinda CK, Port JR, Bushmaker T, et al. K18-hACE2 mice develop respiratory disease resembling severe COVID-19. PLOS Pathog. 2021;17(1):e1009195. doi: 10.1371/journal.ppat.1009195
- Oladunni FS, Park J-G, Pino PA, et al. Lethality of SARS-CoV-2 infection in K18 human angiotensin-converting enzyme 2 transgenic mice. Nat Commun. 2020;11(1):6122. doi: 10.1038/s41467-020-19891-7
- Sun S-H, Chen Q, Gu H-J, et al. A mouse model of SARS-CoV-2 infection and pathogenesis. Cell Host Microbe. 2020;28(1):124–133.e4. doi: 10.1016/j.chom.2020.05.020
- Li K, Wohlford-Lenane C, Perlman S, et al. Middle east respiratory syndrome coronavirus causes multiple organ damage and lethal disease in mice transgenic for human dipeptidyl peptidase 4. J Infect Dis. 2016;213(5):712–722. doi: 10.1093/infdis/jiv499
- Roberts A, Deming D, Paddock CD, et al. A mouse-adapted SARS-coronavirus causes disease and mortality in BALB/c mice. PLOS Pathog. 2007;3(1):e5. doi: 10.1371/journal.ppat.0030005
- Leist SR, Dinnon KH, Schäfer A, et al. A mouse-adapted SARS-CoV-2 induces acute lung injury and mortality in standard laboratory mice. Cell. 2020;183(4):1070–1085.e12. doi: 10.1016/j.cell.2020.09.050
- Dinnon KH, Leist SR, Schäfer A, et al. A mouse-adapted model of SARS-CoV-2 to test COVID-19 countermeasures. Nature. 2020;586(7830):560–566. doi: 10.1038/s41586-020-2708-8
- Li K, Wohlford-Lenane CL, Channappanavar R, et al. Mouse-adapted MERS coronavirus causes lethal lung disease in human DPP4 knockin mice. Proceedings of the National Academy of Sciences. 2017;114:E3119–E3128.
- Israelow B, Song E, Mao T, et al. Mouse model of SARS-CoV-2 reveals inflammatory role of type I interferon signaling. J Exp Med. 2020;217(12):e20201241. doi: 10.1084/jem.20201241
- Hassan AO, Case JB, Winkler ES, et al. A SARS-CoV-2 infection model in mice demonstrates protection by neutralizing antibodies. Cell. 2020;182(3):744–753.e4. doi: 10.1016/j.cell.2020.06.011
- Carabelli AM, Peacock TP, Thorne LG, et al. SARS-CoV-2 variant biology: immune escape, transmission and fitness. Nat Rev Microbiol. 2023;21:162–177. doi: 10.1038/s41579-022-00841-7
- Pan T, Chen R, He X, et al. Infection of wild-type mice by SARS-CoV-2 B.1.351 variant indicates a possible novel cross-species transmission route. Signal Transduct Target Ther. 2021;6(1):420. doi: 10.1038/s41392-021-00848-1
- Stone S, Rothan HA, Natekar JP, et al. SARS-CoV-2 variants of concern infect the respiratory tract and induce inflammatory response in wild-type laboratory mice. Viruses. 2021;14(1):27. doi: 10.3390/v14010027
- Xu J, Zhong S, Liu J, et al. Detection of severe acute respiratory syndrome coronavirus in the brain: potential role of the chemokine mig in pathogenesis. Clin Infect Dis. 2005;41(8):1089–1096. doi: 10.1086/444461
- Hung ECW, Chim SSC, Chan PKS, et al. Detection of SARS coronavirus RNA in the cerebrospinal fluid of a patient with severe acute respiratory syndrome. Clin Chem. 2003;49(12):2108–2109. doi: 10.1373/clinchem.2003.025437
- Yang X, Deng W, Tong Z, et al. Mice transgenic for human angiotensin-converting enzyme 2 provide a model for SARS coronavirus infection. Comp Med. 2007;57(5):450–459.
- Tseng C-TK, Huang C, Newman P, et al. Severe Acute Respiratory Syndrome Coronavirus Infection of Mice Transgenic for the Human Angiotensin-Converting Enzyme 2 Virus Receptor. J Virol. 2007;81(3):1162–1173. doi: 10.1128/JVI.01702-06
- Moreau GB, Burgess SL, Sturek JM, et al. Evaluation of K18-hACE2 mice as a model of SARS-CoV-2 infection. Am J Trop Med Hyg. 2020;103(3):1215–1219. doi: 10.4269/ajtmh.20-0762
- Rathnasinghe R, Strohmeier S, Amanat F, et al. Comparison of transgenic and adenovirus hACE2 mouse models for SARS-CoV-2 infection. Emerg Microbes Infect. 2020;9(1):2433–2445. doi: 10.1080/22221751.2020.1838955
- Zheng J, Wong L-YR, Li K, et al. COVID-19 treatments and pathogenesis including anosmia in K18-hACE2 mice. Nature. 2021;589(7843):603–607. doi: 10.1038/s41586-020-2943-z
- Golden JW, Cline CR, Zeng X, et al. Human angiotensin-converting enzyme 2 transgenic mice infected with SARS-CoV-2 develop severe and fatal respiratory disease. JCI Insight. 2020;5(19):e142032. doi: 10.1172/jci.insight.142032
- Winkler ES, Bailey AL, Kafai NM, et al. SARS-CoV-2 infection of hACE2 transgenic mice causes severe lung inflammation and impaired function. Nat Immunol. 2020;21(11):1327–1335. doi: 10.1038/s41590-020-0778-2
- Solomon IH, Normandin E, Bhattacharyya S, et al. Neuropathological features of covid-19. N Engl J Med. 2020;383(10):989–992. doi: 10.1056/NEJMc2019373
- Chen M, Shen W, Rowan NR, et al. Elevated ACE-2 expression in the olfactory neuroepithelium: implications for anosmia and upper respiratory SARS-CoV-2 entry and replication. Eur Respir J. 2020;56(3):2001948. doi: 10.1183/13993003.01948-2020
- Ye Q, Zhou J, He Q, et al. SARS-CoV-2 infection in the mouse olfactory system. Cell Discov. 2021;7(1):49. doi: 10.1038/s41421-021-00290-1
- Verma AK, Zheng J, Meyerholz DK, et al. SARS-CoV-2 infection of sustentacular cells disrupts olfactory signaling pathways. JCI Insight. 2022;7(24). doi:10.1172/jci.insight.160277.
- Menachery VD,Yount Jr BL, Sims AC, et al. SARS-like WIV1-CoV poised for human emergence. Proceedings of the National Academy of Sciences. 2016;113:3048–3053.
- Jiang R-D, Liu M-Q, Chen Y, et al. Pathogenesis of SARS-CoV-2 in transgenic mice expressing human angiotensin-converting enzyme 2. Cell. 2020;182(1):50–58.e8. doi: 10.1016/j.cell.2020.05.027
- Zhang W, Golynker I, Brosh R, et al. Mouse genome rewriting and tailoring of three important disease loci. Nature. 2023;623(7986):423–431. doi: 10.1038/s41586-023-06675-4
- Rai P, Chuong C, LeRoith T, et al. Adenovirus transduction to express human ACE2 causes obesity-specific morbidity in mice, impeding studies on the effect of host nutritional status on SARS-CoV-2 pathogenesis. Virology. 2021;563:98–106. doi: 10.1016/j.virol.2021.08.014
- Korber B, Fischer WM, Gnanakaran S, et al. Tracking changes in SARS-CoV-2 spike: evidence that D614G increases infectivity of the COVID-19 virus. Cell. 2020;182(4):812–827.e19. doi: 10.1016/j.cell.2020.06.043
- Lorenzo-Redondo R, Nam HH, Roberts SC, et al. A clade of SARS-CoV-2 viruses associated with lower viral loads in patient upper airways. EBioMedicine. 2020;62:103112. doi: 10.1016/j.ebiom.2020.103112
- Hou YJ, Chiba S, Halfmann P, et al. SARS-CoV-2 D614G variant exhibits efficient replication ex vivo and transmission in vivo. Science. 2020;370(6523):1464–1468. doi: 10.1126/science.abe8499
- Zhou B, Thao TTN, Hoffmann D, et al. SARS-CoV-2 spike D614G change enhances replication and transmission. Nature. 2021;592(7852):122–127. doi: 10.1038/s41586-021-03361-1
- Rathnasinghe R, Jangra S, Ye C, et al. Characterization of SARS-CoV-2 spike mutations important for infection of mice and escape from human immune sera. Nat Commun. 2022;13(1):3921. doi: 10.1038/s41467-022-30763-0
- Gu H, Chen Q, Yang G, et al. Adaptation of SARS-CoV-2 in BALB/c mice for testing vaccine efficacy. Science. 2020;369(6511):1603–1607. doi: 10.1126/science.abc4730
- Yu W, Wang J, Yang Y, et al. SARS-CoV-2 omicron (B.1.1.529) infection in rhesus macaques, hamsters, and BALB/c mice with severe lung histopathological damage. J Med Virol. 2023;95(6):e28846. doi: 10.1002/jmv.28846
- Halfmann PJ, Iida S, Iwatsuki-Horimoto K, et al. SARS-CoV-2 omicron virus causes attenuated disease in mice and hamsters. Nature. 2022;603(7902):687–692. doi: 10.1038/s41586-022-04441-6
- Shuai H, Chan JF-W, Yuen T-T-T, et al. Emerging SARS-CoV-2 variants expand species tropism to murines. EBioMedicine. 2021;73:103643. doi: 10.1016/j.ebiom.2021.103643
- Tracking SARS-CoV-2 variants. https://www.who.int/activities/tracking-SARS-CoV-2-variants.
- Hu F-H, Jia Y-J, Zhao D-Y, et al. Clinical outcomes of the severe acute respiratory syndrome coronavirus 2 omicron and delta variant: systematic review and meta-analysis of 33 studies covering 6 037 144 coronavirus disease 2019–positive patients. Clin Microbiol Infect. 2023;29(7):835–844. doi: 10.1016/j.cmi.2023.03.017
- Rizvi ZA, Dandotiya J, Sadhu S, et al. Omicron sub-lineage BA.5 infection results in attenuated pathology in hACE2 transgenic mice. Commun Biol. 2023;6(1):935. doi: 10.1038/s42003-023-05263-6
- Shuai H, Chan JF-W, Hu B, et al. Attenuated replication and pathogenicity of SARS-CoV-2 B.1.1.529 omicron. Nature. 2022;603(7902):693–699. doi: 10.1038/s41586-022-04442-5
- Gao Q, Bao L, Mao H, et al. Development of an inactivated vaccine candidate for SARS-CoV-2. Science. 2020;369(6499):77–81. doi: 10.1126/science.abc1932
- Wang H, Zhang Y, Huang B, et al. Development of an inactivated vaccine candidate, BBIBP-CorV, with potent protection against SARS-CoV-2. Cell. 2020;182(3):713–721.e9. doi: 10.1016/j.cell.2020.06.008
- Zhang N-N, Li X-F, Deng Y-Q, et al. A thermostable mRNA vaccine against COVID-19. Cell. 2020;182(5):1271–1283.e16. doi: 10.1016/j.cell.2020.07.024
- Martinez DR, Schäfer A, Leist SR, et al. Prevention and therapy of SARS-CoV-2 and the B.1.351 variant in mice. Cell Rep. 2021;36(4):109450. doi: 10.1016/j.celrep.2021.109450
- A portfolio of COVID-19 platforms. The Jackson Laboratory https://www.jax.org/jax-mice-and-services/research-continuity/hace2.
- Martina BEE, Haagmans BL, Kuiken T, et al. SARS virus infection of cats and ferrets. Nature. 2003;425(6961):915–915. doi: 10.1038/425915a
- Chu Y-K, Ali GD, Jia F, et al. The SARS-CoV ferret model in an infection–challenge study. Virology. 2008;374(1):151–163. doi: 10.1016/j.virol.2007.12.032
- Kim Y-I, Kim S-G, Kim S-M, et al. Infection and rapid transmission of SARS-CoV-2 in ferrets. Cell Host Microbe. 2020;27(5):704–709.e2. doi: 10.1016/j.chom.2020.03.023
- Wan Y, Shang J, Graham R, et al. Receptor recognition by the novel coronavirus from Wuhan: an analysis based on decade-long structural studies of SARS coronavirus. J Virol. 2020;94(7):e00127–20. doi: 10.1128/JVI.00127-20
- Raj VS, Smits SL, Provacia LB, et al. Adenosine deaminase acts as a natural antagonist for dipeptidyl peptidase 4-mediated entry of the middle east respiratory syndrome coronavirus. J Virol. 2014;88(3):1834–1838. doi: 10.1128/JVI.02935-13
- Pulit-Penaloza JA, Belser JA, Sun X, et al. Comparative assessment of severe acute respiratory syndrome coronavirus 2 variants in the ferret model. MBio. 2022;13(5):e0242122. doi: 10.1128/mbio.02421-22
- Lieber CM, Cox RM, Sourimant J, et al. SARS-CoV-2 VOC type and biological sex affect molnupiravir efficacy in severe COVID-19 dwarf hamster model. Nat Commun. 2022;13(1):4416. doi: 10.1038/s41467-022-32045-1
- van de Ven K, van Dijken H, Wijsman L, et al. Pathology and immunity after SARS-CoV-2 infection in male ferrets is affected by age and inoculation route. Front Immunol. 2021;12. doi: 10.3389/fimmu.2021.750229
- Ryan KA, Bewley KR, Fotheringham SA, et al. Dose-dependent response to infection with SARS-CoV-2 in the ferret model and evidence of protective immunity. Nat Commun. 2021;12(1):81. doi: 10.1038/s41467-020-20439-y
- Shi J, Wen Z, Zhong G, et al. Susceptibility of ferrets, cats, dogs, and other domesticated animals to SARS–coronavirus 2. Science. 2020;368(6494):1016–1020. doi: 10.1126/science.abb7015
- Richard M, Kok A, de Meulder D, et al. SARS-CoV-2 is transmitted via contact and via the air between ferrets. Nat Commun. 2020;11(1):3496. doi: 10.1038/s41467-020-17367-2
- An D, Li K, Rowe DK, et al. Protection of K18-hACE2 mice and ferrets against SARS-CoV-2 challenge by a single-dose mucosal immunization with a parainfluenza virus 5–based COVID-19 vaccine. Sci Adv. 2021;7(27):eabi5246. doi: 10.1126/sciadv.abi5246
- Boley PA, Lee CM, Schrock J, et al. Enhanced mucosal immune responses and reduced viral load in the respiratory tract of ferrets to intranasal lipid nanoparticle-based SARS-CoV-2 proteins and mRNA vaccines. J Nanobiotechnology. 2023;21(1):60. doi: 10.1186/s12951-023-01816-3
- Cox RM, Wolf JD, Plemper RK. Therapeutically administered ribonucleoside analogue MK-4482/EIDD-2801 blocks SARS-CoV-2 transmission in ferrets. Nat Microbiol. 2021;6(1):11–18. doi: 10.1038/s41564-020-00835-2
- Park S-J, Yu K-M, Kim Y-I, et al. Antiviral efficacies of FDA-Approved drugs against SARS-CoV-2 infection in ferrets. MBio. 2020;11(3). doi: 10.1128/mbio.01114-20
- Roberts A, Vogel L, Guarner J, et al. Severe acute respiratory syndrome coronavirus infection of golden Syrian hamsters. J Virol. 2005;79(1):503–511. doi: 10.1128/JVI.79.1.503-511.2005
- Chan JF-W, Zhang AJ, Yuan S, et al. Simulation of the clinical and pathological manifestations of coronavirus disease 2019 (COVID-19) in a Golden Syrian hamster Model: implications for disease pathogenesis and transmissibility. Clinl Infect Dis. 2020;325. doi: 10.1093/cid/ciaa325
- Imai M, Iwatsuki-Horimoto K, Hatta M, et al. Syrian hamsters as a small animal model for SARS-CoV-2 infection and countermeasure development. Proc Natl Acad Sci U S A. 2020;117(28):16587–16595. doi: 10.1073/pnas.2009799117
- Rosenke K, Meade-White K, Letko M, et al. Defining the Syrian hamster as a highly susceptible preclinical model for SARS-CoV-2 infection. Emerg Microbes Infect. 2020;9(1):2673–2684. doi: 10.1080/22221751.2020.1858177
- Trimpert J, Vladimirova D, Dietert K, et al. The roborovski dwarf hamster is a highly susceptible model for a rapid and fatal course of SARS-CoV-2 infection. Cell Rep. 2020;33(10):108488. doi: 10.1016/j.celrep.2020.108488
- Zhai C, Wang M, Chung H-J, et al. Roborovski hamster (Phodopus roborovskii) strain SH101 as a systemic infection model of SARS-CoV-2. Virulence. 2021;12(1):2430–2442. doi: 10.1080/21505594.2021.1972201
- Bertzbach LD, Vladimirova D, Dietert K, et al. SARS-CoV-2 infection of Chinese hamsters (cricetulus griseus) reproduces COVID-19 pneumonia in a well-established small animal model. Transbound Emerg Dis. 2021;68(3):1075–1079. doi: 10.1111/tbed.13837
- Gruber AD, Firsching TC, Trimpert J, et al. Hamster models of COVID-19 pneumonia reviewed: how human can they be? Vet Pathol. 2022;59(4):528–545. doi: 10.1177/03009858211057197
- de Melo GD, Lazarini F, Levallois S, et al. COVID-19–related anosmia is associated with viral persistence and inflammation in human olfactory epithelium and brain infection in hamsters. Sci, Trans Med. 2021;13(596):eabf8396. doi: 10.1126/scitranslmed.abf8396
- Sia SF, Yan L-M, Chin AWH, et al. Pathogenesis and transmission of SARS-CoV-2 in golden hamsters. Nature. 2020;583(7818):834–838. doi: 10.1038/s41586-020-2342-5
- Song Z, Bao L, Yu P, et al. SARS-CoV-2 causes a systemically multiple organs damages and dissemination in hamsters. Front Microbiol. 2021;11. doi: 10.3389/fmicb.2020.618891
- Osterrieder N, Bertzbach LD, Dietert K, et al. Age-dependent progression of SARS-CoV-2 infection in Syrian hamsters. Viruses. 2020;12(7):779. doi: 10.3390/v12070779
- Varga Z, Flammer AJ, Steiger P, et al. Endothelial cell infection and endotheliitis in COVID-19. Lancet. 2020;395(10234):1417–1418. doi: 10.1016/S0140-6736(20)30937-5
- Dhakal S, Ruiz-Bedoya CA, Zhou R, et al. Sex differences in lung imaging and SARS-CoV-2 antibody responses in a COVID-19 golden syrian hamster model. MBio. 2021;12(4):e0097421. doi: 10.1128/mBio.00974-21
- Oishi K, Horiuchi S, Frere J, et al. A diminished immune response underlies age-related SARS-CoV-2 pathologies. Cell Rep. 2022;39(13):111002. doi: 10.1016/j.celrep.2022.111002
- Mohandas S, Shete A, Kumar A, et al. Comparative pathogenicity of BA.2.12, BA.5.2 and XBB.1 with the delta variant in Syrian hamsters. Front Microbiol. 2023;14:1183763. doi: 10.3389/fmicb.2023.1183763
- Yuan S, Ye Z-W, Liang R, et al. Pathogenicity, transmissibility, and fitness of SARS-CoV-2 omicron in Syrian hamsters. Science. 2022;377(6604):428–433. doi: 10.1126/science.abn8939
- Uraki R, Halfmann PJ, Iida S, et al. Characterization of SARS-CoV-2 omicron BA.4 and BA.5 isolates in rodents. Nature. 2022;612(7940):540–545. doi: 10.1038/s41586-022-05482-7
- Sharma S, Vercruysse T, Sanchez-Felipe L, et al. Updated vaccine protects against SARS-CoV-2 variants including omicron (B.1.1.529) and prevents transmission in hamsters. Nat Commun. 2022;13(1):6644. doi: 10.1038/s41467-022-34439-7
- Abdelnabi R, Foo CS, Zhang X, et al. The omicron (B.1.1.529) SARS-CoV-2 variant of concern does not readily infect Syrian hamsters. Antiviral Res. 2022;198:105253. doi: 10.1016/j.antiviral.2022.105253
- Suzuki R, Yamasoba D, Kimura I, et al. Attenuated fusogenicity and pathogenicity of SARS-CoV-2 omicron variant. Nature. 2022;603(7902):700–705. doi: 10.1038/s41586-022-04462-1
- Li J, Yu X, Hu S, et al. COVID-19 targets the right lung. Crit Care. 2020;24(1):339. doi: 10.1186/s13054-020-03033-y
- Andreotti S, Altmüller J, Quedenau C, et al. De novo-whole genome assembly of the roborovski dwarf hamster (phodopus roborovskii) genome: an animal model for severe/critical COVID-19. Genome Biol Evol. 2022;14(7):evac100. doi: 10.1093/gbe/evac100
- Fouchier RAM, Kuiken, T., Schutten, M., et al. Koch’s postulates fulfilled for SARS virus. Nature. 2003;423:240–240. doi: 10.1038/423240a 6937
- McAuliffe J, Vogel L, Roberts A, et al. Replication of SARS coronavirus administered into the respiratory tract of African green, rhesus and cynomolgus monkeys. Virology. 2004;330(1):8–15. doi: 10.1016/j.virol.2004.09.030
- Haagmans BL, Kuiken T, Martina BE, et al. Pegylated interferon-α protects type 1 pneumocytes against SARS coronavirus infection in macaques. Nat Med. 2004;10(3):290–293. doi: 10.1038/nm1001
- Rowe T, Gao G, Hogan RJ, et al. Macaque Model for Severe Acute Respiratory Syndrome. J Virol. 2004;78(20):11401–11404. doi: 10.1128/JVI.78.20.11401-11404.2004
- Kuiken T, Fouchier RA, Schutten M, et al. Newly discovered coronavirus as the primary cause of severe acute respiratory syndrome. Lancet. 2003;362(9380):263–270. doi: 10.1016/S0140-6736(03)13967-0
- de Wit E, Rasmussen, AL, and Falzarano D,et al. Middle east respiratory syndrome coronavirus (MERS-CoV) causes transient lower respiratory tract infection in rhesus macaques. Proceedings of the National Academy of Sciences. 2013;110:16598–16603.
- Rockx B, Kuiken T, Herfst S, et al. Comparative pathogenesis of COVID-19, MERS, and SARS in a nonhuman primate model. Science. 2020;368(6494):1012–1015. doi: 10.1126/science.abb7314
- Lu S, Zhao Y, Yu W, et al. Comparison of nonhuman primates identified the suitable model for COVID-19. Sig Transduct Target Ther. 2020;5(1):1–9. doi: 10.1038/s41392-020-00269-6
- Shan C, Yao Y-F, Yang X-L, et al. Infection with novel coronavirus (SARS-CoV-2) causes pneumonia in rhesus macaques. Cell Res. 2020;30(8):670–677. doi: 10.1038/s41422-020-0364-z
- Munster VJ, Feldmann F, Williamson BN, et al. Respiratory disease in rhesus macaques inoculated with SARS-CoV-2. Nature. 2020;585(7824):268–272. doi: 10.1038/s41586-020-2324-7
- Singh DK, Singh B, Ganatra SR, et al. Responses to acute infection with SARS-CoV-2 in the lungs of rhesus macaques, baboons and marmosets. Nat Microbiol. 2021;6(1):73–86. doi: 10.1038/s41564-020-00841-4
- Yu P, Qi F, Xu Y, et al. Age-related rhesus macaque models of COVID-19. AMEM. 2020;3(1):93–97. doi: 10.1002/ame2.12108
- Johnston SC, Ricks KM, Jay A, et al. Development of a coronavirus disease 2019 nonhuman primate model using airborne exposure. PloS One. 2021;16(2):e0246366. doi: 10.1371/journal.pone.0246366
- Chandrashekar A, Liu J, Martinot AJ, et al. SARS-CoV-2 infection protects against rechallenge in rhesus macaques. Science. 2020;369(6505):812–817. doi: 10.1126/science.abc4776
- Blair RV, Vaccari M, Doyle-Meyers LA, et al. Acute respiratory distress in aged, SARS-CoV-2–infected African green monkeys but not rhesus macaques. Am J Pathol. 2021;191(2):274–282. doi: 10.1016/j.ajpath.2020.10.016
- Woolsey C, Borisevich V, Prasad AN, et al. Establishment of an African green monkey model for COVID-19 and protection against re-infection. Nat Immunol. 2021;22(1):86–98. doi: 10.1038/s41590-020-00835-8
- Hartman AL, Nambulli S, McMillen CM, et al. SARS-CoV-2 infection of African green monkeys results in mild respiratory disease discernible by PET/CT imaging and shedding of infectious virus from both respiratory and gastrointestinal tracts. PLOS Pathogens. 2020;16(9):e1008903. doi: 10.1371/journal.ppat.1008903
- Lankau EW, Turner PV, Mullan RJ, et al. Use of nonhuman primates in research in North America. J Am Assoc Lab Anim Sci. 2014;53(3):278–282.
- Carlsson H-E, Schapiro SJ, Farah I, et al. Use of primates in research: a global overview. Am J Primatol. 2004;63(4):225–237. doi: 10.1002/ajp.20054
- Pedersen RM, Tornby DS, Bang LL, et al. Rectally shed SARS-CoV-2 in COVID-19 inpatients is consistently lower than respiratory shedding and lacks infectivity. Clin Microbiol Infect. 2022;28(2):304.e1–304.e3. doi: 10.1016/j.cmi.2021.10.023
- Oh T, Kim G, Baek SH, et al. Comparative spatial transcriptomic profiling of severe acute respiratory syndrome coronavirus 2 delta and omicron variants infections in the lungs of cynomolgus macaques. J Med Virol. 2023;95(6):e28847. doi: 10.1002/jmv.28847
- van Doremalen N, Singh M, Saturday TA, et al. SARS-CoV-2 omicron BA.1 and BA.2 are attenuated in rhesus macaques as compared to delta. Sci Adv. 2022;8(46):eade1860. doi: 10.1126/sciadv.ade1860
- Munster VJ, Flagg M, Singh M, et al. Subtle differences in the pathogenicity of SARS-CoV-2 variants of concern B.1.1.7 and B.1.351 in rhesus macaques. Sci Adv. 2021;7(43):eabj3627. doi: 10.1126/sciadv.abj3627
- Gagne M, Moliva JI, Foulds KE, et al. mRNA-1273 or mRNA-omicron boost in vaccinated macaques elicits similar B cell expansion, neutralizing responses, and protection from omicron. Cell. 2022;185(9):1556–1571.e18. doi: 10.1016/j.cell.2022.03.038
- Chandrashekar A, Yu J, McMahan K, et al. Vaccine protection against the SARS-CoV-2 Omicron variant in macaques. Cell. 2022;185(9):1549–1555.e11. doi: 10.1016/j.cell.2022.03.024
- Solforosi L, Costes LMM, Tolboom JTBM, et al. Booster with Ad26.COV2.S or omicron-adapted vaccine enhanced immunity and efficacy against sars-cov-2 omicron in macaques. Nat Commun. 2023;14(1):1944. doi: 10.1038/s41467-023-37715-2
- Chang MC, Hild S, Grieder F. Nonhuman primate models for SARS-CoV-2 research: consider alternatives to macaques. Lab Anim. 2021;50(5):113–114. doi: 10.1038/s41684-021-00755-6
- Williamson BN, Feldmann F, Schwarz B, et al. Clinical benefit of remdesivir in rhesus macaques infected with SARS-CoV-2. Nature. 2020;585(7824):273–276. doi: 10.1038/s41586-020-2423-5
- Hoang TN, Pino M, Boddapati AK, et al. Baricitinib treatment resolves lower-airway macrophage inflammation and neutrophil recruitment in SARS-CoV-2-infected rhesus macaques. Cell. 2021;184(2):460–475.e21. doi: 10.1016/j.cell.2020.11.007
- Cáceres CJ, Cardenas-Garcia S, Carnaccini S, et al. Efficacy of GC-376 against SARS-CoV-2 virus infection in the K18 hACE2 transgenic mouse model. Sci Rep. 2021;11(1):9609. doi: 10.1038/s41598-021-89013-w
- Villadiego J, García-Arriaza J, Ramírez-Lorca R, et al. Full protection from SARS-CoV-2 brain infection and damage in susceptible transgenic mice conferred by MVA-CoV2-S vaccine candidate. Nat Neurosci. 2023;26(2):226–238. doi: 10.1038/s41593-022-01242-y
- Prompetchara E, Ketloy C, Alameh M-G, et al. Immunogenicity and protective efficacy of SARS-CoV-2 mRNA vaccine encoding secreted non-stabilized spike in female mice. Nat Commun. 2023;14(1):2309. doi: 10.1038/s41467-023-37795-0
- Tarrés-Freixas F, Trinité B, Pons-Grífols A, et al. Heterogeneous infectivity and pathogenesis of SARS-CoV-2 variants beta, delta and omicron in transgenic K18-hACE2 and wildtype mice. Front Microbiol. 2022;13:840757. doi: 10.3389/fmicb.2022.840757
- Aiolfi R, Ahmed J, de la Torre JC, et al. Sars-cov-2 infection promotes endothelial dysfunction and thrombosis in a mouse model of COVID-19. Blood. 2021;138(Supplement 1):999. doi: 10.1182/blood-2021-148713
- Chen Y, Li C, Liu F, et al. Age-associated SARS-CoV-2 breakthrough infection and changes in immune response in a mouse model. Emerg Microbes Infect. 2022;11(1):368–383. doi: 10.1080/22221751.2022.2026741
- Sriramula S, Theobald D, Parekh RU, et al. Emerging role of kinin B1 receptor in persistent neuroinflammation and neuropsychiatric symptoms in mice following recovery from SARS-CoV-2 infection. Cells. 2023;12(16):2107. doi: 10.3390/cells12162107
- Sadhu S, Dalal R, Dandotiya J, et al. IL-9 aggravates SARS-CoV-2 infection and exacerbates associated airway inflammation. Nat Commun. 2023;14(1):4060. doi: 10.1038/s41467-023-39815-5
- Geng J, Chen L, Yuan Y, et al. CD147 antibody specifically and effectively inhibits infection and cytokine storm of SARS-CoV-2 and its variants delta, alpha, beta, and gamma. Signal Transduct Target Ther. 2021;6(1):347. doi: 10.1038/s41392-021-00760-8
- Wu J, Chen L, Qin C, et al. CD147 contributes to SARS-CoV-2-induced pulmonary fibrosis. Signal Transduct Target Ther. 2022;7(1):382. doi: 10.1038/s41392-022-01230-5
- Zost SJ, Gilchuk P, Case JB, et al. Potently neutralizing and protective human antibodies against SARS-CoV-2. Nature. 2020;584(7821):443–449. doi: 10.1038/s41586-020-2548-6
- Alsoussi WB, Turner JS, Case JB, et al. A potently neutralizing antibody protects mice against SARS-CoV-2 infection. J Immunol. 2020;205(4):915–922. doi: 10.4049/jimmunol.2000583
- Tian J-H, Patel N, Haupt R, et al. SARS-CoV-2 spike glycoprotein vaccine candidate NVX-CoV2373 immunogenicity in baboons and protection in mice. Nat Commun. 2021;12(1):372. doi: 10.1038/s41467-020-20653-8
- Yong KSM, Anderson DE, Zheng AKE, et al. Comparison of infection and human immune responses of two SARS-CoV-2 strains in a humanized hACE2 NIKO mouse model. Sci Rep. 2023;13(1):12484. doi: 10.1038/s41598-023-39628-y
- Corbett KS, Edwards DK, Leist SR, et al. SARS-CoV-2 mRNA vaccine design enabled by prototype pathogen preparedness. Nature. 2020;586(7830):567–571. doi: 10.1038/s41586-020-2622-0
- Wu S, Zhong G, Zhang J, et al. A single dose of an adenovirus-vectored vaccine provides protection against SARS-CoV-2 challenge. Nat Commun. 2020;11(1):4081. doi: 10.1038/s41467-020-17972-1
- Rogers TF, Zhao F, Huang D, et al. Isolation of potent SARS-CoV-2 neutralizing antibodies and protection from disease in a small animal model. Science. 2020;369(6506):956–963. doi: 10.1126/science.abc7520
- Hermet P, Delache B, Herate C, et al. Broadly neutralizing humanized SARS-CoV-2 antibody binds to a conserved epitope on spike and provides antiviral protection through inhalation-based delivery in non-human primates. PLOS Pathog. 2023;19(8):e1011532. doi: 10.1371/journal.ppat.1011532
- Neary M, Sharp J, Gallardo-Toledo E, et al. Evaluation of Nafamostat as chemoprophylaxis for SARS-CoV-2 infection in hamsters. Viruses. 2023;15(8):1744. doi: 10.3390/v15081744
- de Moor WRJ, Williamson A-L, Schäfer G, et al. LSDV-Vectored SARS-CoV-2 S and N vaccine protects against severe clinical disease in hamsters. Viruses. 2023;15(7):1409. doi: 10.3390/v15071409
- Rosenke K, Okumura A, Lewis MC, et al. Molnupiravir inhibits SARS-CoV-2 variants including omicron in the hamster model. JCI Insight. 2022;7(13):e160108. doi: 10.1172/jci.insight.160108
- Wyler E, Adler JM, Eschke K, et al. Key benefits of dexamethasone and antibody treatment in COVID-19 hamster models revealed by single-cell transcriptomics. Mol Ther. 2022;30(5):1952–1965. doi: 10.1016/j.ymthe.2022.03.014
- de Melo GD, Perraud V, Alvarez F, et al. Neuroinvasion and anosmia are independent phenomena upon infection with SARS-CoV-2 and its variants. Nat Commun. 2023;14(1):4485. doi: 10.1038/s41467-023-40228-7
- Yang Z, Johnson BA, Meliopoulos VA, et al. Interaction between host G3BP and viral nucleocapsid protein regulates SARS-CoV-2 replication. bioRxiv. 2023;2023(6.29.546885). doi: 10.1101/2023.06.29.546885
- Abdelaziz MO, Raftery MJ, Weihs J, et al. Early protective effect of a (“pan”) coronavirus vaccine (PanCovac) in Roborovski dwarf hamsters after single-low dose intranasal administration. Front Immunol. 2023;14:1166765. doi: 10.3389/fimmu.2023.1166765
- McMahan K, Yu J, Mercado NB, et al. Correlates of protection against SARS-CoV-2 in rhesus macaques. Nature. 2021;590(7847):630–634. doi: 10.1038/s41586-020-03041-6
- Willcox AC, Sung K, Garrett ME, et al. Detailed analysis of antibody responses to SARS-CoV-2 vaccination and infection in macaques. PLOS Pathog. 2022;18(4):e1010155. doi: 10.1371/journal.ppat.1010155
- Everett HE, Lean FZX, Byrne AMP, et al. Intranasal infection of ferrets with SARS-CoV-2 as a model for asymptomatic human infection. Viruses. 2021;13(1):113. doi: 10.3390/v13010113
- Sefik E, Israelow B, Mirza H, et al. A humanized mouse model of chronic COVID-19. Nat Biotechnol. 2022;40(6):906–920. doi: 10.1038/s41587-021-01155-4