ABSTRACT
The Picornaviridae are a large group of positive-sense, single-stranded RNA viruses, and most research has focused on the Enterovirus genus, given they present a severe health risk to humans. Other picornaviruses, such as foot-and-mouth disease virus (FMDV) and senecavirus A (SVA), affect agricultural production with high animal mortality to cause huge economic losses. The 3Dpol protein of picornaviruses is widely known to be used for genome replication; however, a growing number of studies have demonstrated its non-polymerase roles, including modulation of host cell biological processes, viral replication complex assembly and localization, autophagy, and innate immune responses. Currently, there is no effective vaccine to control picornavirus diseases widely, and clinical therapeutic strategies have limited efficiency in combating infections. Many efforts have been made to develop different types of drugs to prohibit virus survival; the most important target for drug development is the virus polymerase, a necessary element for virus replication. For picornaviruses, there are also active efforts in targeted 3Dpol drug development. This paper reviews the interaction of 3Dpol proteins with the host and the progress of drug development targeting 3Dpol.
Introduction
The Picornaviridae family comprises 158 species organized into 68 genera (https://www.picornaviridae.com). Human enteroviruses have been classified into four categories: A, B, C, and D, based on their molecular and biological properties. Nearly 100 subtypes have been found such as enterovirus A71 (EV-A71), enterovirus D68 (EV-D68), coxsackievirus A6 (CVA6), coxsackievirus B3 (CVB3), echovirus, poliovirus (PV), etc [Citation1,Citation2]. Although poliovirus has been successfully eradicated in most countries because of effective vaccination programs, other members of the Picornaviridae family still pose a severe threat to human health, especially EV-A71, coxsackievirus, and hepatitis A virus (HAV) [Citation3–7]. Due to the large number of enterovirus serotypes, the cross-protective efficacy of current vaccines appear to be insufficient in the face of simultaneous transmission of multiple serotypes [Citation8]. In addition, several picornaviruses are significant threats to the farming industry. Foot-and-mouth disease virus (FMDV) infection causes vesicular disease in cloven-hoofed animals, with high mortality rates in young animals [Citation9]. Senecavirus A (SVA), another agent of vesicular disease in swine, has indistinguishable clinical symptoms with illness caused by FMDV [Citation10]. Both viruses generate substantial economic losses and remind us of the emerging risks that must be taken seriously in farmed livestock.
The genomes of the Picornaviridae family have similar backbones, with a positive-stranded RNA genome between 6.7 kb and 10.1 kb in size and a single open reading frame (ORF) flanked by untranslated regions (UTRs) [Citation11,Citation12]. As shown in , the 5” end of the genome is linked to VPg, which can be uridylated by the 3Dpol protein to act as a primer for transcription [Citation1,Citation13]. The 5”-UTR contains the internal ribosome entry site (IRES), which directs the cap-independent initiation of protein synthesis [Citation14]. The 3’-UTR has a stem-loop structure and polyA tail, which is essential for picornaviruses [Citation15]. All Picornaviridae members have a single ORF, which translates a polyprotein that is cleaved by the viral proteases 2Apro, 3Cpro, and 3 CDpro into the precursor proteins P1, P2, and P3 [Citation14,Citation16]. The P1 precursor protein is then cleaved into four structural proteins, which we have designated VP1, VP2, VP3, and VP4; the P2 precursor protein is processed into nonstructural proteins 2Apro, 2B, and 2C; and the P3 precursor protein undergoes proteolytic cleavage into nonstructural proteins 3A, 3B, 3Cpro, and 3Dpol [Citation12]. Some picornaviruses, such as aphthoviruses, cardioviruses, and kobuviruses, additionally express a leader protein (Lpro) at the N-terminal end of the polyprotein, after which the polyprotein is cleaved to form the Lpro, P1, P2, and P3 precursor proteins [Citation17]. In addition, the coding region of the picornavirus genome has an essential element, the internal cis-acting replication element (cre), which serves as a template for VPg uridylation [Citation18,Citation19].
Figure 1. A schematic representation of the EV-A71 genome and current understanding of the picornaviruses 3Dpro protein. The enterovirus 5” end is linked to a VPg followed by an IRES. After translation of the single OFR, the polyprotein is cascade cleaved to form VP1, VP2, VP3, VP4, 2Apro, 2B, 2C, 3A, 3B, 3Cpro, and 3Dpol. at the 3” end of the EV-A71 genome is a polyA sequence. 3Dpol proteins catalyse viral RNA synthesis, catalyse VPg uridylation, interact with host proteins, and act as drug targets.
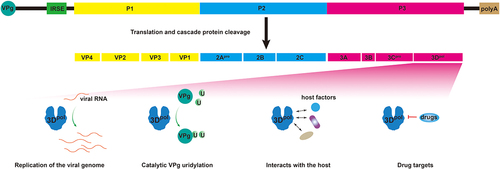
Over the past few years, we have gained a deeper understanding of members of the Picornaviridae family. The picornavirus 3Dpro has been recognized as a critical component for viral genome replication, but other functions are progressively being identified. Here, we review the recent advances in understanding the biological functions of picornavirus 3Dpro during virus infection and briefly summarize the current strategies and progress of drug design and development targeting picornavirus 3Dpro.
Characterization of the picornavirus 3Dpol
The picornavirus RNA-dependent RNA polymerase (RdRp), also known as 3Dpol, is located at the C-terminus of the viral polyprotein. Only following a series of cleavages from the precursor protein does 3Dpol exhibit a polymerase activity [Citation20]. Several picornavirus 3Dpol structures have been solved, which have shown highly conserved structural and functional elements [Citation20–23](). RdRp is the critical protein responsible for viral replication; the overall structure of all RdRps resembles a cupped right hand with palm, finger, and thumb domains. The finger and thumb contact each other to form the NTP entry channel, and becomes catalytic by a subtle conformational change in the palm domain, which cooperates to perform highly specialized functions including nucleotide recognition, catalysis of nucleotide binding, and initiation of RNA replication [Citation24,Citation25]. RdRp also has VPg uridylation activity; following nucleotidylation by RdRp, uridylated VPg can act as a primer for virus genome replication [Citation26–28]().
Figure 2. Structures of the RdRps of picornaviruses. a: EV-A71 3Dpol protein (PDB: 3N6L); b: EV-D68 3Dpol protein (PDB: 6L4R); c: CVA16 3Dpol protein (PDB: 5Y6Z); d: CVB3 3Dpol protein (PDB: 3DDK); e: PV 3Dpol protein (PDB: 4K4S); and f: FMDV 3Dpol protein (PDB: 6S2L). The palm, thumb and finger subdomains are shown in blue, cyan and magenta, respectively.
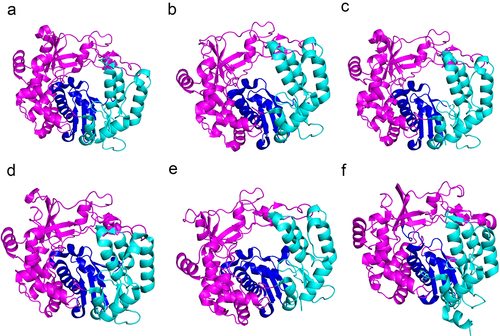
Advances in picornavirus 3Dpol-host interactions
As the structures of 3Dpol and RNA complexes have been solved, the mechanism of picornavirus RNA synthesis, in which 3Dpol functions as a polymerase, has been well explored. Given that 3Dpol is critical for virus replication, it has been suggested that 3Dpol-host interactions affect the formation of the 3Dpol-RNA replication complex. On the other hand, 3Dpol-host interactions enrich our knowledge of 3Dpol functions beyond its polymerase role and provide insights about antiviral strategies targeting protein-protein interaction sites. Here, we summarize the advances in 3Dpol-host interactions in enterovirus, FMDV, and SVA ().
Figure 3. Diverse functions of the picornavirus 3Dpol protein and interactions with multiple host factors.
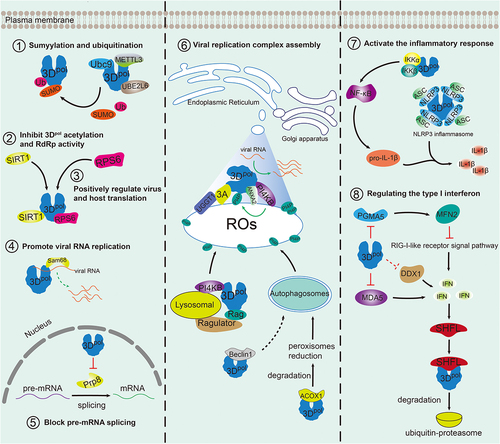
Enterovirus
Enteroviruses are a group of RNA viruses belonging to the family Picornaviridae. Their 3Dpol proteins perform diverse and complex functions in their interactions with host proteins.
Ubiquitination and SUMOylation are widely studied post-translational modifications (PTMs) that are crucial in various biological processes [Citation29–31]. The protein Ubc9 can transfer SUMO to target proteins [Citation32]. EV-A71 encoded 3Dpol can be SUMOylated and K63-linked ubiquitinated in a SUMO-dependent manner through interacting with Ubc9, which contributes to 3Dpol intracellular stability and enhances viral replication [Citation33]. The host factor methyltransferase METTL3 interacts with the 3Dpol of EV-A71 and regulates SUMOylation and ubiquitination of 3Dpol [Citation34]. The nuclear NAD-dependent deacetylase Sirtuin 1 (SIRT1) protein deacetylates many substrates, such as p53, and Notch1 (NICD) [Citation35–37]. EV-A71 infection induces upregulation of SIRT1 expression and translocation from the nucleus to the cytoplasm, where SIRT1 interacts with the EV-A71 3Dpol pinky finger domain to reverse 3Dpol acetylation and inhibit RdRp activity [Citation38]. In addition to this, EV-A71 3Dpol interacts with several cellular proteins to regulate cellular transcription-translation processes during infection. Picornavirus genome synthesis occurs in the cytoplasm, whereas 3Dpol has a nuclear localization signal that enters the nucleus and targets the pre-mRNA processing factor 8 (Prp8) to block host pre-mRNA splicing and mRNA synthesis [Citation39]. EV-A71 3Dpol can interact with the ribosomal protein S6 (RPS6) to promote IRES-dependent and cap-dependent translation, positively regulating viral and host translation [Citation40].
Picornaviruses relocate cellular regulators associated with membrane metabolism and modulate their activity to rearrange intracellular membranes to form a genome replication scaffold. These are also called replication organelles (ROs) [Citation41,Citation42]. Enteroviruses 3A and 3Dpol recruit phosphatidylinositol 4-kinase III (PI4KB) to secretory organelle membranes to promote RO formation [Citation43–45]. In addition, PI4KB catalyzes the production of phosphatidylinositol-4-phosphate (PI4P), and PI4P binding with 3Dpol promotes 3Dpol binding to RO membranes and regulates RdRp activity [Citation45]. Annexin A2 (ANXA2), a member of the annexin family, positively regulates EV-A71 replication. ANXA2 promotes PI4KB and 3Dpol interactions and forms an ANXA2-PI4KB-3Dpol complex localized to ROs to promote the formation of the EV-A71 RNA replication complex [Citation46]. Recent studies found that EV-A71 likely utilizes the Ragulator-Rag to regulate cell death in order to facilitate its replication. Ragulator is a heteropentameric protein complex composed of LAMTOR1, LAMTOR2, LAMTOR3, LAMTOR4, and LAMTOR5, with LAMTOR1 anchoring the Ragulator to the lysosomal membrane [Citation47]. Rag GTPase comprises of heterodimers RagA/B and RagC/D interacting with the Ragulator [Citation48]. The mechanism of the regulation of enterovirus replication by Ragulator-Rag is complex. Knockout or knockdown of Ragulator components inhibits EV-A71 infection-induced apoptosis and pyroptosis. Interestingly, EV-A71 replication requires the Ragulator-Rag complex, as genomic replication and viral titres are significantly reduced in LAMTOR1-KO, LAMTOR3-KO, and RagB-KO cells [Citation49]. For 3Dpol functions in ROs, previous studies showed the regulation of the PI4KB-3Dpol complex in ROs [Citation46], while further studies from another group indicated that 3Dpol directly interacts with RagB, facilitating PI4KB and 3Dpol localization at the lysosome [Citation49]. The complexes on the lysosomal membrane formed by Ragulator, Rag, PI4KB, and 3Dpol through protein-protein interactions help virus replication, as breaking the localization of the complexes in the lysosome reduces EV-A71 production [Citation49]. Based on the Ragulator-Rag-3Dpol axis, the inhibitor ZHSI-1 was screened to target 3Dpol and significantly inhibit the localization of 3Dpol and PI4KB on the lysosomal surface to limit enterovirus replication in vitro and in vivo [Citation49]. However, it is uncertain whether the Ragulator, Rag, PI4KB, and 3Dpol complexes are translocated into ROs upon EV-A71 infection-induced rearrangement of intracellular membranes. Ragulator, Rag, PI4KB, and 3Dpol complexes in lysosomal aggregates may act as viral replication sites or promote the formation of ROs, which requires further study. Additionally, the endoplasmic reticulum (ER) protein UDP-glucose glycoprotein glucosyltransferase 1 (UGGT1) is a crucial regulator of the unfolded protein response and has been shown to promote the formation of EV-A71 replication complexes. Immunoprecipitation analysis revealed that enterovirus nonstructural protein 3A acts as a bridge that allows UGGT1 and 3Dpol to establish indirect interactions to form replication complexes on RO membranes. Although UGGT1 does not interact directly with 3Dpol, its facilitation of 3A-3Dpol interactions enhances viral RNA synthesis and positively regulates the replication of EV-A71 and EV-D68 [Citation50]. It is clear that 3Dpol localization of ROs is essential for RNA virus replication and that picornaviruses achieve this by hijacking host proteins or utilizing self-encoded proteins through a complex protein-protein interaction process. Blocking the interplay between 3Dpol and host factors also provides new ideas for drug development.
Positive-sense RNA viruses can utilize the membrane structure of autophagosomes to promote viral replication [Citation42]. Recent studies have identified a strong interaction between EV-A71 3Dpol and autophagy. Beclin1 is an autophagy protein that regulates innate immune pathways [Citation51,Citation52]. RNA interference and overexpression experiments showed that the expression level of Beclin1 and EV-A71 replication were positively correlated [Citation53], suggesting that EV-A71 infection can induce autophagy to enhance viral replication [Citation54,Citation55]. Previous studies showed that Beclin1 promotes the formation of double membrane structures [Citation56,Citation57], while the mechanisms of 3Dpol-Beclin1 interactions that promote EV-A71 replication need to be better understood. Further studies revealed that two structural domains of Beclin 1, the coiled-coiled domain (CCD) and evolutionarily conserved domain (ECD), are responsible for interacting with 3Dpol and are essential for facilitating EV-A71 replication [Citation53]. This interaction may contribute to the formation of ROs and enable 3Dpol localization to the membrane and/or participate in regulating innate immunity to promote viral replication. Additionally, peroxisomes are dynamic organelles that play an essential role in multiple metabolic pathways and redox homoeostasis [Citation58,Citation59]. EV-A71 3Dpol interacts with peroxisomal protein acyl-CoA oxidase 1 (ACOX1) and degrades ACOX1 via the proteasome pathway. Moreover, infection with EV-A71 or knockdown of ACOX1 reduced the number of peroxisomes, ultimately inducing apoptosis and autophagy [Citation60].
Inflammatory cytokines and interferons are essential for fighting microbial infections [Citation61–63]. EV-A71 activates interleukin-1beta (IL-1β) by regulating the NLR family PYRIN domain containing-3 (NLRP3) inflammasome, and the RdRp activity of EV-A71 3Dpol is critical for EV-A71-induced NLRP3 inflammasome activation. Further studies revealed that 3Dpol interacts with NLRP3 to form a 3Dpol-NLRP3-ASC ring-like structure that promotes the assembly of the inflammasome complex [Citation64]. In addition, 3Dpol is involved in the regulation of type I interferon responses. Melanoma differentiation-associated protein 5 (MDA5) is a crucial pathogen recognition receptor that initiates type I interferon transcription [Citation65,Citation66]. 3Dpol of EV-A71 interacts with the caspase activation and recruitment domains (CARDs) of MDA5 to inhibit MDA5-mediated type I interferon activation signalling. Similarly, the coxsackievirus B3 3Dpol interacts with MDA5 to inhibit type I interferon activation [Citation67]. The 3Dpol of EV-D68 interacts with PGAM family member 5 (PGMA5), resulting in the downregulation of PGMA5 and upregulation of mitofusin 2 (MFN2) expression. Furthermore, MFN2 inhibits the activation of the RIG-I-like receptor signal pathway to suppress interferon beta (IFN-β) production, thereby promoting viral replication [Citation68]. EV-A71 infection induces upregulation of the expression of the interferon-stimulating factor shiftless antiviral inhibitor of ribosomal frameshifting gene (SHFL), and EV-A71 3Dpol interacts with SHFL after 3Dpol is degraded via the ubiquitin-proteasome pathway [Citation69]. It is apparent that enterovirus 3Dpol inhibits the type I interferon antiviral response by different mechanisms.
FMDV and SVA
FMDV and SVA are severe threats to agricultural production. Both viruses belong to the family Picornaviridae and are similar in many respects, including viral invasion and replication. Therefore, studies on their interactions with the host are of interest to sustainable agriculture and human health. Currently, several studies are dedicated to revealing the interactions of FMDV and SVA 3Dpol with the host.
As described above, EV-A71 3Dpol SUMOylation and ubiquitination modifications improve 3Dpol stability in cells [Citation33,Citation34]. A recent study found that overexpression of ubiquitin-conjugating enzyme E2 L6 (UBE2L6) promotes SVA replication, and conversely, knockout of UBE2L6 inhibits SVA replication; further studies showed that UBE2L6 interacts with 3Dpol to mediate 3Dpol ubiquitination and inhibit 3Dpol degradation [Citation70]. The host nuclear protein 68 kDa Src-associated substrate during mitosis (Sam68) is an RNA-binding protein involved in cellular processes within viral infections [Citation71,Citation72]. A previous study found that Sam68 interacts with 3Dpol and then relocates from the nucleus to the cytoplasm to regulate viral replication [Citation73]. Redistribution of Sam68 was similarly observed upon FMDV or bovine enterovirus 1 (BEV-1) infection, and Sam68 directly interacts with FMDV IRES. Knockdown of Sam68 using RNAi significantly inhibited FMDV replication and IRES activity [Citation71]. Further research suggests that Sam68 May be involved in FMDV RNA synthesis. Experimental studies coupled with computational predictions have shown direct binding between Sam68 and FMDV-encoded 3Dpol [Citation74]. Although the significance of the Sam68-3Dpol interaction on viral infection has yet to be determined and needs to be further explored, the above findings suggest Sam68 could act as a mediator to make FMDV RNA and 3Dpol spatially closer, thus promoting daughter RNA synthesis.
We previously described that EV-A71 3Dpol binding to NLRP3 promotes inflammasome complex assembly; SVA 3Dpol interacts with NLRP3 to activate the inflammasome assembly. In addition, SVA 3Dpol interacts with IKKα and IKKβ to activate NF-κB, thereby promoting pro-IL-1β transcription [Citation75]. DEAD-box RNA helicase 1 (DDX1) regulates RNA metabolism and can control viral replication [Citation76,Citation77]. A recent study identified DDX1 interacting with 3Dpol of FMDV by two-hybrid experiments. DDX1 inhibits the replication of FMDV, which is partly related to the activity of the DDX1 ATPase/helicase. In addition, DDX1 stimulates the activation of the IFN-β pathway in FMDV-infected cells, and experiments showed that FMDV infection upregulated DDX1 mRNA expression. Yet, the protein expression level of DDX1 was reduced [Citation78]. However, the functional details of DDX1-3Dpol interaction are unclear. Beyond indirect IFN-β effects operated by DDX1 under FMDV infection, whether 3Dpol interaction with DDX1 leads to DDX1 degradation is an exciting line of research. The IFN pathway is one of the primary mechanisms for host cells to fight pathogens [Citation62]. For 3Dpol regulation of the IFN pathway, a recent study showed that FMDV-encoded 3Dpol inhibits activation of the IFN pathway by interacting with multiple elements involved in the IFN pathway, including IKKα, IKKε, IRF3, IRF7, NEMO, MDA5, and MAVS. Interestingly, species differences exist in these protein-protein interactions, such as 3Dpol only interacting with porcine-derived MAVS [Citation79]. Obviously, the 3Dpol protein of FMDV and SVA plays a non-negligible role in viral RNA replication and is involved in diverse host cell biological processes by interacting with host factors to impose effects on virus pathogenicity.
Drugs acting on 3Dpol
Picornavirus genome replication is dependent on 3Dpol. Considering the critical role of 3Dpol proteins in the viral life cycle, 3Dpol is an attractive target for the design of antiviral strategies [Citation16,Citation80–82]. Indeed, viral RdRp has been one of the targets for developing antiviral drugs. For example, FDA-approved Remdesivir is used for COVID-19 and Ebola virus infection [Citation83], and Ribavirin is used to combat hepatitis C virus (HCV) infection [Citation84]. Polymerase inhibitors can be divided into two categories. The first category belongs to nucleoside and nucleotide substrate analog inhibitors, which target the active site of the viral polymerase. The second category is allosteric inhibitors, which inhibit the polymerase by binding to allosteric sites. Here, we summarize the drugs targeting picornaviruses 3Dpol.
Nucleoside/Nucleotide analogs
Nucleoside analog inhibitors are usually present in vivo as prodrugs that undergo metabolic activation to nucleotide forms. Nucleoside analogs mimic the entry of natural nucleotides into RdRp and inhibit RdRp by causing mutagenic effects and chain extension termination. The excessive rate of genetic mutation leads to genetic information loss, which is dangerous for organisms [Citation85]. Currently, multiple nucleoside analogs are under development for use against picornaviruses () ().
Table 1. RNA-dependent RNA polymerase nucleoside/nucleotide analog inhibitors.
Pyrimidine ribonucleoside triphosphate analog (rPTP) is a potent mutagen for the PV genome, with in vitro incorporation properties superior to those of ribavirin triphosphate [Citation87]. The N-6-modified purine analog as a mutagen significantly inhibited poliovirus (PV) and coxsackievirus replication in vitro. At the same time, a high-fidelity PV mutant (G64S) exhibited resistance to this analog, which suggests the mutagenic effect of this analog plays an antiviral role [Citation88].
In addition to the mutagenic impacts on the viral genome, inhibition of viral RNA synthesis is an essential antiviral strategy. 5-nitrocytidine triphosphate has a high affinity for PV 3Dpol and probably inhibits PV and CVB3 replication by competitively inhibiting RdRp activity [Citation89].
The adenosine analog NITD008 was previously demonstrated to block flavivirus RNA synthesis. However, NITD008 is toxic to rats [Citation90]. NITD008 also showed inhibitory effects against EV-A71, and in vivo experiments demonstrated that it protected mice from clinical signs or death [Citation91]. The NITD008 triphosphate acts as a chain terminator to inhibit EV-A71 RdRp activity directly [Citation92]. Mutations conferring NITD008 resistance were localized to the viral 3A and 3Dpol regions. Reverse genetics confirmed that mutations in 3A and 3Dpol resulted in resistance to NITD008, and that a combination of mutations in 3A and 3Dpol resulted in stronger resistance [Citation91]. Considering the toxicity of NITD008 in vivo, a series of novel NITD008 derivatives have also been synthesized. The half maximal inhibitory concentration (IC50) of phosphoamidate (15f) containing a hexyl ester of l-alanine against EV-A71 was 0.13 ± 0.08 μM. The derivative containing a cyclohexyl ester of l-alanine (15 l) showed a high selectivity index (SI) against EV-A71 (IC50 = 0.19 ± 0.27 μM, SI = 117.00) and EV-D68 (IC50 = 0.17 ± 0.16 μM, SI = 130.76). The toxicity of these NTD008 derivatives in vivo are yet to be determined [Citation93].
2’,2’-difluoro-2’-deoxycytidine, also known as gemcitabine, had anti-poliovirus potential by high-throughput screening and inhibition of PV replication in HeLa cells at an IC50 of 0.3 μM. Sensitization to gemcitabine varied between cell lines, and although 25 μM gemcitabine had no detectable cytotoxicity in HeLa cells, 0.1 μM gemcitabine was sufficient to kill Vero cells [Citation94]. Further evaluation of the in vivo toxicity of these NITD008 derivatives and gemcitabine is important to determine their potential as anti-viral drugs.
2’-Deoxy-2’-β-fluoro-4’-azidocytidine, also known as azvudine or FNC, a cytidine analog, has been previously shown to have potent antiviral activity against HCV, human immunodeficiency virus (HIV), and human and duck hepatitis B virus (HBV) [Citation95,Citation96]. In 2021, this drug received marketing approval in China for the treatment of HIV infection (https://www.nmpa.gov.cn/yaowen/ypjgyw/ypyw/20210721142223181.html). Recently, FNC was found to effectively inhibit viral replication of EV-A71, EV-D68, CVA6, CVA16, and CVB3 by targeting 3Dpol, with 50% effective concentrations (EC50) ranging from 1.548 nM to 52.12 nM. Animal experiments show FNC (1 mg/kg) protecting mice from lethal EV-A71 or CVA16 challenges [Citation97]. In light of these studies, FNC is a promising drug for treating enterovirus infections.
Remdesivir (GS-5734) is a monophosphate prodrug of an adenosine nucleoside analog that is reported to have broad antiviral activity by targeting viral RdRp [Citation98,Citation99]. Recent studies have identified that GS-5734 inhibits RNA synthesis of EV-A71, hindering enterovirus replication, and shows broad-spectrum inhibition of a wide range of enteroviruses [Citation100]. Similarly, current clinical data showed that vaccine-derived poliovirus infection in an immunocompromised patient was cured by GS-5734 treatment after multiple failed attempts to treat with human milk, ribavirin, and oral human immunoglobulin [Citation101].
Some drugs have multiple mechanisms of mutagenic effect and inhibit chain elongation. The pyrazinecarboxamide derivative, T-1105 (3-hydroxy-2-pyrazinecarboxamide), provides strong in vitro antiviral activity against a wide range of FMDV serotypes and completely protects pigs (orally administered 200 mg/kg) against FMDV infection [Citation102]. T-1105 fluorinated derivative favipiravir (6-fluoro-3-hydroxy-2-pyrazinecarboxamide), called T-705, selectively inhibits RdRp of influenza and many other RNA viruses [Citation103]. In 2020, a clinical case was reported using favipiravir and zanamivir to clear influenza B infection in an immunocompromised child [Citation104]. 100 μM favipiravir treatment significantly reduced HAV RNA levels by 80% in cell cultures. The present results suggest that the mutagenic effect of favipiravir in the HAV genome plays a crucial role in the antiviral process [Citation105]. However, recombination of picornavirus genes rescues viruses from error catastrophe [Citation106].
Treatment with the base analog 5-fluorouracil (FU) resulted in an increased frequency of mutations in FMDV, and replication inhibition was observed [Citation107,Citation108]. The FMDV MARLS mutant, which has high fitness in BHK-21 cells, showed greater resistance to FU [Citation107]. FU is converted intracellularly to FUTP, which competitively inhibits VPg uridylation, and FUMP is inserted into the UMP or CMP site of the template to induce mutagenesis [Citation109]. This suggests a dual action of FU-disruption of VPg uridylation and mutagenic inhibition of FMDV. However, the fact that FU is not easily absorbed by cells has hampered its application, so modifications to FU are being made [Citation110].
Ribavirin is a guanosine nucleoside analog that has been widely used in clinical practice for decades and exhibits a broad spectrum of antiviral activity through five mechanisms, including inosine monophosphate dehydrogenase inhibition, immunomodulatory effects, interference with RNA capping, polymerase inhibition, and lethal mutagenesis [Citation111]. Ribavirin eliminates FMDV from persistently infected cell cultures [Citation112,Citation113]. Treatment with ribavirin in an adult mouse model (50 mg/kg/day, intraperitoneal injection, twice daily for 3 days) had an 86.67% protective efficiency before FMDV challenge and 66.67% protective efficiency after FMDV challenge. Both pre and post-treatment with ribavirin protected suckling mice (50 mg/kg, intraperitoneal injection) from lethal challenge [Citation86]. Vaccines take time to produce protection against viruses, and the co-administration of ribavirin (1800 mg/pig) with the FMDV vaccine enhanced early antiviral resistance [Citation113]. Mutagenesis remains the primary mechanism of ribavirin antiviral activity. Ribavirin induces over-mutation in the poliovirus genome, forcing an “error catastrophe” that inhibits viral replication [Citation85,Citation114]. The mutagenic ribavirin adulterates the viral genome, leading to lethal mutagenesis, but ribavirin treatment may also lead to the emergence of resistant viral populations in the presence of low to moderate concentrations of the drug [Citation115].
In addition to the nucleoside analog inhibitors mentioned above, other analogs have equally good antiviral activity. 7-deaza-6-methyl-9-β-d-ribofuranosylpurine effectively inhibits poliovirus, but it does not act as a mutagen or strand terminator for 3Dpol, instead potentially exerting its antiviral effects through destabilization of the PV RdRp-RNA primer/template complex or by interfering with the host state [Citation116]. 2’-modified nucleoside analogs can compete with the substrate nucleoside triphosphate to inhibit HCV RNA synthesis [Citation117]. Ribonucleoside analog 2’-C-methylcytidine (2’-C-MetCyt) exhibits antiviral activity against FMDV and SVDV [Citation118]. Similarly, 2’-C-MetCyt inhibited human parechovirus A1 (HPeV1) and parechovirus A3 (HPeV3) replication in vitro [Citation119]. The anti-EV-A71 activity of 2’-C-MetCyt was also confirmed in human small intestinal organoids (HIOs) and RD cells [Citation120].
Non-nucleoside inhibitors
The inhibitory mechanism of non-nucleoside analog inhibitors is ambiguous, usually binding to the RdRp variable site to inhibit the enzyme conformational change or binding to the active site to impede substrate entry. Several non-nucleoside analog inhibitors have been shown to inhibit picornaviruses replication () ().
Table 2. RNA-dependent RNA polymerase non-nucleoside inhibitors.
Amiloride and its derivatives inhibit CVB3 replication, and mutational analyses showed that either a serine-to-threonine (S299T) substitution at amino acid 299 or an alanine-to-valine (A372V) substitution at amino acid 372 of the CVB3 polymerase conferred CVB3 resistance to amiloride and its derivatives. Amino acids at positions 299 and 372 of 3Dpol are in the structural modality of catalytic activity, suggesting that amiloride and its derivatives act as CVB3 inhibitors of 3Dpol [Citation121]. Further study showed that amiloride and its derivatives competitively inhibit the entry of nucleoside triphosphate and Mg2+ into CVB3 3Dpol, inhibiting VPg uridylation and chain elongation [Citation122]. Ogram et al. also found that amiloride inhibits CVB3 and PV1 RNA replication, and computer models found that amiloride docks into the VPg binding site on 3Dpol to inhibit VPg uridylation [Citation123]. The inhibitory activity of various amiloride derivatives on CVB3 3Dpol has been further investigated. Modification of the 5-amino group of amiloride increased the inhibitory activity, whereas 5-guanidino group substitution decreased the inhibitory activity. 3,5-diaminopyrazinyl substitution led to complete loss of the inhibitory activity, and the combination of 5-amino and guanidino group substitutions was more effective than both single substitutions [Citation124]. Amiloride compounds alter intracellular ion concentrations, affecting polymerase fidelity and leading to indirect mutagenic activity [Citation125]. Recent studies have shown that amiloride can also resist FMDV infection [Citation126]. In conclusion, amiloride has a broad-spectrum resistance to picornaviruses through multiple mechanisms.
Previous studies have found that piperazine-containing pyrazolo[3,4-d]pyrimidine derivatives have antiviral activity against EV-A71 [Citation127]. The further discoveries of piperazine-containing pyrazolo[3,4-d] pyrimidine derivatives, DTriP-22, showed broad antiviral activity against picornaviruses. DTriP-22 was found to act as an inhibitor of EV-A71 RNA polymerase and inhibit its elongation activity. Reverse genetics demonstrated that an arginine-to-lysine amino acid substitution at residue 163 (R163K) within the 3D polymerase conferred resistance to DTriP-22 [Citation128].
Aurintricarboxylic acid (ATA) inhibits the elongation activity of viral 3Dpol to inhibit EV-A71 viral replication [Citation129]. In addition, the study found that ATA inhibits the inflammatory response induced by EV-A71 3Dpol [Citation64]. Further evaluation of the in vivo antiviral capacity of ATA is warranted.
Compound 5D9 is a non-competitive inhibitor of FMDV polymerase for NTP and nucleic acid substrates. Notably, 5D9 inhibited FMDV replication in a dose-dependent manner and no resistant mutants were observed to arise after consecutive passages [Citation130,Citation131]. De Palma et al. presented the first non-nucleoside inhibitors targeting the RNA template channel of the picornaviruses polymerase, GPC-N114, with broad-spectrum resistance to enteroviruses and cardioviruses. Mechanistically GPC-N114 targets the polymerase template receptor nucleotide site. Notably, no resistance was obtained under GPC-N114 pressure in CVB3 or poliovirus. In contrast, 3D polymerase amino acid substitutions of both M300V and I304V allowed encephalomyocarditis virus to acquire resistance. The CVB3 3Dpol with GPC-N114 X-ray crystal structure (PDB: 4Y2A) indicates that GPC-N114 is located at the bottom of the RNA-binding channel [Citation132](). However, currently GPC-N114 cannot be formulated for animal administration and further optimization of its structure is necessary.
Figure 6. X-ray crystal structure of 3Dpol and viral polymerase inhibitors. a: CVB3 3Dpol in complex with GPC-N114 (PDB: 4Y2A); b: EV-D68 3Dpol in complex with NADPH (PDB: 5ZIT). The palm, thumb and finger subdomains are shown in blue, cyan, and magenta cartoons, respectively. The inhibitors are shown in red sticks.
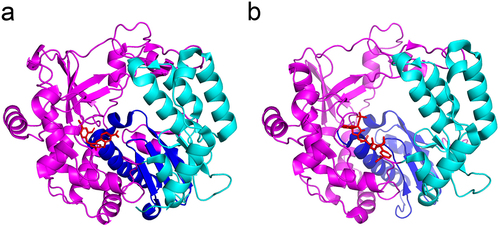
The inhibitor NADPH occupies the RNA template-binding channel of the EV-D68 RdRp and NADPH inhibits EV-D68, poliovirus, and EV-A71 RdRp activity in vitro. Furthermore, the X-ray crystal structure of the 3Dpol-NADPH complex shows the residues involved in the NADPH-binding pocket of the EV-D68 3Dpol are highly conserved in enterovirus 3Dpol (PDB: 5ZIT), suggesting that NADPH holds promise as an inhibitor of enterovirus () [Citation133]. NADPH showed inhibition of RdRp in vitro, but related cellular and animal experiments need to be performed. Seven potential poliovirus RdRp inhibitors were identified through high-throughput screening, and five were found to bind the enzyme active sites of the polymerase extension complex through X-ray crystallography. These screened inhibitors occupy the NTP binding site and interact with template RNA, mainly blocking polymerase initiation. These compounds were ineffective in stopping the elongation process of the enzyme, probably due to the lack of the triphosphate moiety [Citation134]. However, the in vivo inhibition of viral replication is unclear.
BPR-3P0128 inhibits the replication of various RNA viruses, including influenza, human rhinovirus, coxsackievirus, and enterovirus [Citation135]. Velu et al. showed that BPR-3P0128 inhibited EV-A71 replication in a dose-dependent manner; mechanistically, BPR-3P0128 inhibited EV-A71 RdRp activity and VPg uridylation. Moreover, no resistant strains were observed after 11 serial passages [Citation136]. Sorafenib, a c-RAF and multi-kinase inhibitor, is approved for treating liver cancer [Citation137]. Drug repurposing of sorafenib has been shown to inhibit viral replication in a wide range of viruses, and recent studies have found that sorafenib targets the FMDV 3Dpol active site, inhibiting polymerase activity and modulating the c-RAF and AKT/PI3K pathways to inhibit FMDV replication [Citation138].
Tenovin-1 derivative AcTU showed broad-spectrum resistance against enteroviruses such as EV-A71, EV-D68, CVA16, CVA21, and CVB1. Mechanistically, AcTU inhibited viral replication by targeting 3Dpol, and excitingly, in vivo experiments demonstrated that oral administration of AcTU at 0.6 mg/kg/d to mice was highly protective against lethal EV-A71 attack [Citation139]. Jeong et al. found 2-amino-4-arylthiazole targets FMDV 3Dpol by high throughput screening (HTS) adapting fluorescence polarization (FP), and in vitro evaluations of its derivatives 20i and 24a showed more effective inhibition of FMDV [Citation140]. Theerawatanasirikul et al. screened compounds and found NSC217697 (quinoline), NSC670283 (spiro compound), NSC292567 (nigericin), and NSC65850 target 3Dpol to inhibit FMDV replication in vitro [Citation141].
Based on 3Dpol and host protein interactions, a recent screen identified a novel inhibitor, ZHSI-1, that suppresses the replication of EV-A71 and CAV by inhibiting 3Dpol localization on the lysosomal membrane, providing adequate protection against EV-A71 infection in mice [Citation49]. These compounds provide a basis for the design of more efficient antiviral drugs.
Conclusion
The Picornaviridae family of viruses are severe threats to human and animal health. With recent advances, the picornavirus genome and protein functions are becoming more apparent. The research on EV-A71 3Dpol has been relatively in-depth compared to several other viruses of the same family. Current knowledge suggests the mechanisms of modification and regulation of host signalling pathways by 3Dpol are similar in picornaviruses, providing more information for the study of picornaviruses. The PTM of 3Dpol is critical for its stability and activity, and 3Dpol activates inflammatory responses. This offers new insights for the development of drugs that have antiviral activity or treat virus-induced inflammatory reactions. Interestingly, 3Dpol carries a nuclear localization signal that regulates host RNA splicing [Citation39]. An exciting direction to investigate would be additional roles for 3Dpol within the nucleus. In addition, further studies of 3Dpol localization at intracellular membranes are essential to understanding picornavirus replication. Finally, an increasing amount of evidence on the interplay between 3Dpol and type I interferon responses suggests a role for 3Dpol in modulating the host immune response. In summary, the 3Dpol protein of picornaviruses plays a vital role in viral replication and cell biological processes involved in the viral lifecycle, such as autophagy, inflammatory response formation, and type I interferon response caused by 3Dpol-host interactions.
The 3Dpol protein plays an indispensable role in the replication of picornaviruses and is highly conserved in structure and core functional elements, therefore it is a promising drug target. We have summarized the current discovery of drugs that most effectively target 3Dpol and inhibit of picornavirus replication. The two main categories of drugs are nucleotide analog inhibitors and non-nucleotide analog inhibitors. These inhibitors have exhibited good antiviral activity in vitro.
However, several issues need to be considered during the development of 3Dpol-targeting drugs. It is worth noting that different cells have different sensitivities to the drugs. Therefore, more attention needs to be paid to the issue of drug cytotoxicity [Citation94]. Several drugs, such as Ribavirin, NITD008, FNC, T-1105, GS-5734, and AcTU, showed antiviral activity in vivo, and their broad spectrum protection against picornaviruses deserves to be further investigated. Additionally, several drugs have already been approved for treating other diseases, so repurposing of these drugs will significantly shorten the drug development cycle. The picornavirus polymerase has low fidelity, and a single drug tends to induce the production of drug-resistant mutants. Cocktail chemotherapy has been widely used in cancer therapy and similar attempts in the treatment of EV-A71 have been shown to delay the development of drug-resistant mutants [Citation142,Citation143]. Viruses resist drugs by rapidly mutating and as more and more targeted drugs are developed, drug resistance needs to be addressed. Notably, in vitro passages with two non-nucleoside analog drugs, GPC-N114 and BPR-3P0128, did not produce resistant mutants. Remarkably, the interactions between picornavirus 3Dpol and the host facilitate viral replication. Studies have identified a novel inhibitor that blocks 3Dpol-host interactions, thereby inhibiting viral replication [Citation49]. These interactions provide a new direction for targeted 3Dpol drug screening.
In conclusion, there are still many undiscovered areas in the interactions between 3Dpol proteins and host organisms. Further studies of 3Dpol-host interactions are necessary, particularly on replication complex assembly and antagonism of innate immunity, which will be critical for understanding picornavirus replication and pathogenicity. Importantly, targeting 3Dpol-host interactions is also an exciting direction for new drug development.
Author contributions
J.P. and H.Z. wrote the initial draft with input from Z.N., F.Z., and H.Z., Z.N., F.Z., H.Z., H.Z., and J.P. edited the final draft.
Availability of data
Data sharing is not applicable to this article as no new data were created or analysed in this study.
Disclosure statement
No potential conflict of interest was reported by the author(s).
Additional information
Funding
References
- Solomon T, Lewthwaite P, Perera D, et al. Virology, epidemiology, pathogenesis, and control of enterovirus 71. Lancet Infect Dis. 2010;10(11):778–18. doi: 10.1016/S1473-3099(10)70194-8
- Zell R, Delwart E, Gorbalenya AE, et al. ICTV virus taxonomy profile: Picornaviridae. J Gen Virol. 2017;98(10):2421–2422. doi: 10.1099/jgv.0.000911
- Zhu P, Ji W, Li D, et al. Current status of hand-foot-and-mouth disease. J Biomed Sci. 2023;30(1):15. doi: 10.1186/s12929-023-00908-4
- Zhou J, Li Y, Yin Q, et al. Coxsackievirus A6 pneumonia in a child. Lancet Infect Dis. 2023;23(12):e567. doi: 10.1016/S1473-3099(23)00576-5
- Dai J, Xu D, Yang C, et al. Severe pneumonia and pathogenic damage in human airway epithelium caused by coxsackievirus B4. Emerg Microbes Infect. 2023;12(2):2261560. doi: 10.1080/22221751.2023.2261560
- Zhang X-S, Ong JJ, Macgregor L, et al. Transmission dynamics of the 2016-18 outbreak of hepatitis a among men who have sex with men in England and cost-effectiveness analysis of vaccination strategies to prevent future outbreaks. Lancet Reg Health Eur. 2022;19:100426. doi: 10.1016/j.lanepe.2022.100426
- Carrillo-Santisteve P, Tavoschi L, Severi E, et al. Seroprevalence and susceptibility to hepatitis a in the European Union and European Economic Area: a systematic review. Lancet Infect Dis. 2017;17(10):e306–e319. doi: 10.1016/S1473-3099(17)30392-4
- Tambyah PA, Oon J, Asli R, et al. An inactivated enterovirus 71 vaccine is safe and immunogenic in healthy adults: a phase I, double blind, randomized, placebo-controlled, study of two dosages. Vaccine. 2019;37(31):4344–4353. doi: 10.1016/j.vaccine.2019.06.023
- di Nardo A, Ferretti L, Wadsworth J, et al. Evolutionary and ecological drivers shape the emergence and extinction of foot-and-mouth disease virus lineages. Mol Biol Evol. 2021;38(10):4346–4361. doi: 10.1093/molbev/msab172
- Maggioli Mayara F, Lawson S, de Lima M, et al. Adaptive immune responses following Senecavirus a infection in pigs. J Virol. 2018;92(3):e01717. doi: 10.1128/JVI.01717-17
- Chen J, Chu Z, Zhang M, et al. Molecular characterization of a novel clade echovirus 3 isolated from patients with hand-foot-and-mouth disease in southwest China. J Med Virol. 2023;95(11):e29202. doi: 10.1002/jmv.29202
- Peng T, Yang F, Yang F, et al. Structural diversity and biological role of the 5’ untranslated regions of picornavirus. RNA Biol. 2023;20(1):548–562. doi: 10.1080/15476286.2023.2240992
- Ferrer-Orta C, Ferrero DS, Verdaguer N, et al. Dual role of the foot-and-mouth disease virus 3B1 protein in the replication complex: as protein primer and as an essential component to recruit 3Dpol to membranes. PLoS Path. 2023;19(5):e1011373. doi: 10.1371/journal.ppat.1011373
- Abdullah Sahibzada W, Je W, Wang X, et al. Advances and breakthroughs in IRES-directed translation and replication of picornaviruses. MBio. 2023;14(2):e00358–23. doi: 10.1128/mbio.00358-23
- Zhou L, Lu X, Zhao C, et al. Characterization of a novel picornavirus prevalent in experimental rabbits (Oryctolagus cuniculus). Heliyon. 2023;9(5):e15702. doi: 10.1016/j.heliyon.2023.e15702
- Linden LV, Wolthers KC, van Kuppeveld FJM. Replication and inhibitors of enteroviruses and parechoviruses. Viruses. 2015;7(8):4529–4562. doi: 10.3390/v7082832
- Jackson T, Belsham GJ. Picornaviruses: a view from 3A. Viruses. 2021;13(3):456. doi: 10.3390/v13030456
- Paul Aniko V, Rieder E, Kim Dong W, et al. Identification of an RNA hairpin in poliovirus RNA that serves as the primary template in the in vitro uridylylation of VPg. J Virol. 2000;74(22):10359–10370. doi: 10.1128/JVI.74.22.10359-10370.2000
- Yang Y, Rijnbrand R, McKnight Kevin L, et al. Sequence requirements for viral RNA replication and VPg uridylylation directed by the internal cis-acting replication element (cre) of human rhinovirus type 14. J Virol. 2002;76(15):7485–7494. doi: 10.1128/JVI.76.15.7485-7494.2002
- Campagnola G, Weygandt M, Scoggin K, et al. Crystal structure of coxsackievirus B3 3Dpol highlights the functional importance of residue 5 in picornavirus polymerases. J Virol. 2008;82(19):9458–9464. doi: 10.1128/JVI.00647-08
- Love RA, Maegley KA, Yu X, et al. The crystal structure of the RNA-dependent RNA polymerase from human rhinovirus: A dual function target for common cold antiviral therapy. Structure. 2004;12(8):1533–1544. doi: 10.1016/j.str.2004.05.024
- Thompson AA, Peersen OB. Structural basis for proteolysis-dependent activation of the poliovirus RNA-dependent RNA polymerase. EMBO J. 2004;23(17):3462–3471. doi: 10.1038/sj.emboj.7600357
- Ferrer-Orta C, Arias A, Perez-Luque R, et al. Structure of foot-and-mouth disease virus RNA-dependent RNA polymerase and its complex with a template-primer RNA. J Biol Chem. 2004;279(45):47212–47221. doi: 10.1074/jbc.M405465200
- Gong P, Peersen OB. Structural basis for active site closure by the poliovirus RNA-dependent RNA polymerase. Proc Natl Acad Sci USA. 2010;107(52):22505–22510. doi: 10.1073/pnas.1007626107
- Watkins CL, Kempf BJ, Beaucourt S, et al. Picornaviral polymerase domain exchanges reveal a modular basis for distinct biochemical activities of viral RNA-dependent RNA polymerases. J Biol Chem. 2020;295(31):10624–10637. doi: 10.1074/jbc.RA120.013906
- Paul AV, Yin J, Mugavero J, et al. A “slide-back” mechanism for the initiation of protein-primed RNA synthesis by the RNA polymerase of poliovirus. J Biol Chem. 2003;278(45):43951–43960. doi: 10.1074/jbc.M307441200
- Paul AV, van Boom JH, Filippov D, et al. Protein-primed RNA synthesis by purified poliovirus RNA polymerase. Nature. 1998;393(6682):280–284. doi: 10.1038/30529
- Paul Aniko V, Peters J, Mugavero J, et al. Biochemical and genetic studies of the VPg uridylylation reaction catalyzed by the RNA polymerase of poliovirus. J Virol. 2003;77(2):891–904. doi: 10.1128/JVI.77.2.891-904.2003
- Sheng Z, Zhu J, Deng Y-N, et al. Sumoylation modification-mediated cell death. Open Biol. 2021;11(7):210050. doi: 10.1098/rsob.210050
- Zhu X, Qiu C, Wang Y, et al. FGFR1 SUMOylation coordinates endothelial angiogenic signaling in angiogenesis. Proc Natl Acad Sci U S A. 2022;119(26):e2202631119. doi: 10.1073/pnas.2202631119
- Buschmann T, Fuchs SY, Lee C-G, et al. Sumo-1 modification of mdm2 prevents its self-ubiquitination and increases mdm2 ability to ubiquitinate p53. Cell. 2000;101(7):753–762. doi: 10.1016/S0092-8674(00)80887-9
- Bernier-Villamor V, Sampson DA, Matunis MJ, et al. Structural basis for E2-mediated SUMO conjugation revealed by a complex between ubiquitin-conjugating enzyme Ubc9 and RanGAP1. Cell. 2002;108(3):345–356. doi: 10.1016/S0092-8674(02)00630-X
- Liu Y, Zheng Z, Shu B, et al. SUMO modification stabilizes enterovirus 71 polymerase 3D to facilitate viral replication. J Virol. 2016;90(23):10472–10485. doi: 10.1128/JVI.01756-16
- Hao H, Hao S, Chen H, et al. N6-methyladenosine modification and METTL3 modulate enterovirus 71 replication. Nucleic Acids Res. 2019;47(1):362–374. doi: 10.1093/nar/gky1007
- Imai S-I, Armstrong CM, Kaeberlein M, et al. Transcriptional silencing and longevity protein Sir2 is an NAD-dependent histone deacetylase. Nature. 2000;403(6771):795–800. doi: 10.1038/35001622
- Jia X, Liu H, Ren X, et al. Nucleolar protein NOC4L inhibits tumorigenesis and progression by attenuating SIRT1-mediated p53 deacetylation. Oncogene. 2022;41(39):4474–4484. doi: 10.1038/s41388-022-02447-y
- Wang W, Li Y, Zhang Y, et al. SIRT1 mediates the inhibitory effect of Dapagliflozin on EndMT by inhibiting the acetylation of endothelium Notch1. Cardiovasc Diabetol. 2023;22(1):331. doi: 10.1186/s12933-023-02040-x
- Han Y, Wang L, Cui J, et al. SIRT1 inhibits EV71 genome replication and RNA translation by interfering with the viral polymerase and 5‘UTR RNA. J Cell Sci. 2016;129(24):4534–4547. doi: 10.1242/jcs.193698
- Liu Y-C, Kuo R-L, Lin J-Y, et al. Cytoplasmic viral RNA-dependent RNA polymerase disrupts the intracellular splicing machinery by entering the nucleus and interfering with Prp8. PLoS Path. 2014;10(6):e1004199. doi: 10.1371/journal.ppat.1004199
- Lee K-M, Wu C-C, Wu S-E, et al. The RNA-dependent RNA polymerase of enterovirus A71 associates with ribosomal proteins and positively regulates protein translation. RNA Biol. 2020;17(4):608–622. doi: 10.1080/15476286.2020.1722448
- van der Schaar HM, Dorobantu CM, Albulescu L, et al. Fat(al) attraction: picornaviruses usurp lipid transfer at membrane contact sites to create replication organelles. Trends Microbiol. 2016;24(7):535–546. doi: 10.1016/j.tim.2016.02.017
- Belov GA, van Kuppeveld FJM. (+)RNA viruses rewire cellular pathways to build replication organelles. Curr Opin Virol. 2012;2(6):740–747. doi: 10.1016/j.coviro.2012.09.006
- Xiao X, Lei X, Zhang Z, et al. Enterovirus 3A facilitates viral replication by promoting phosphatidylinositol 4-kinase IIIβ-ACBD3 interaction. J Virol. 2017;91(19):e00791–17. doi: 10.1128/JVI.00791-17
- Greninger Alexander L, Knudsen Giselle M, Betegon M, et al. The 3A protein from multiple picornaviruses utilizes the golgi adaptor protein ACBD3 to recruit PI4KIIIβ. J Virol. 2012;86(7):3605–3616. doi: 10.1128/JVI.06778-11
- Hsu N-Y, Ilnytska O, Belov G, et al. Viral reorganization of the secretory pathway generates distinct organelles for RNA replication. Cell. 2010;141(5):799–811. doi: 10.1016/j.cell.2010.03.050
- Zhang Q, Li S, Lei P, et al. ANXA2 facilitates enterovirus 71 infection by interacting with 3D polymerase and PI4KB to assist the assembly of replication organelles. Virol Sin. 2021;36(6):1387–1399. doi: 10.1007/s12250-021-00417-4
- Mu Z, Wang L, Deng W, et al. Structural insight into the Ragulator complex which anchors mTORC1 to the lysosomal membrane. Cell Discov. 2017;3(1):17049. doi: 10.1038/celldisc.2017.49
- Su M-Y, Morris KL, Kim DJ, et al. Hybrid structure of the RagA/C-Ragulator mTORC1 activation complex. Mol Cell. 2017;68(5):835–846.e3. doi: 10.1016/j.molcel.2017.10.016
- Wang X, Hu Z, Zhang W, et al. Inhibition of lysosome-tethered ragulator-rag-3D complex restricts the replication of enterovirus 71 and coxsackie A16. J Cell Bio. 2023;222(12):e202303108. doi: 10.1083/jcb.202303108
- Huang P-N, Jheng J-R, Arnold JJ, et al. UGGT1 enhances enterovirus 71 pathogenicity by promoting viral RNA synthesis and viral replication. PLoS Path. 2017;13(5):e1006375. doi: 10.1371/journal.ppat.1006375
- Ning Z, Zhong X, Wu Y, et al. β-asarone improves cognitive impairment and alleviates autophagy in mice with vascular dementia via the cAMP/PKA/CREB pathway. Phytomedicine. 2023;123:155215. doi: 10.1016/j.phymed.2023.155215
- Xu J, Kong L, Oliver BA, et al. Constitutively active autophagy in macrophages dampens inflammation through metabolic and post-transcriptional regulation of cytokine production. Cell Rep. 2023;42(7):112708. doi: 10.1016/j.celrep.2023.112708
- Xiang Q, Wan P, Yang G, et al. Beclin1 binds to enterovirus 71 3D protein to promote the virus replication. Viruses. 2020;12(7):756. doi: 10.3390/v12070756
- Fu Y, Xu W, Chen D, et al. Enterovirus 71 induces autophagy by regulating has-miR-30a expression to promote viral replication. Antiviral Res. 2015;124:43–53. doi: 10.1016/j.antiviral.2015.09.016
- Abernathy E, Mateo R, Majzoub K, et al. Differential and convergent utilization of autophagy components by positive-strand RNA viruses. PLoS Biol. 2019;17(1):e2006926. doi: 10.1371/journal.pbio.2006926
- Zhong Y, Wang QJ, Li X, et al. Distinct regulation of autophagic activity by Atg14L and Rubicon associated with beclin 1-phosphatidylinositol-3-kinase complex. Nat Cell Biol. 2009;11(4):468–476. doi: 10.1038/ncb1854
- Chen X, Wang K, Xing Y, et al. Coronavirus membrane-associated papain-like proteases induce autophagy through interacting with Beclin1 to negatively regulate antiviral innate immunity. Protein Cell. 2014;5(12):912–927. doi: 10.1007/s13238-014-0104-6
- Jo DS, Park NY, Cho D-H. Peroxisome quality control and dysregulated lipid metabolism in neurodegenerative diseases. Exp Mol Med. 2020;52(9):1486–1495. doi: 10.1038/s12276-020-00503-9
- Liu J, Lu W, Shi B, et al. Peroxisomal regulation of redox homeostasis and adipocyte metabolism. Redox Biol. 2019;24:101167. doi: 10.1016/j.redox.2019.101167
- You L, Chen J, Liu W, et al. Enterovirus 71 induces neural cell apoptosis and autophagy through promoting ACOX1 downregulation and ROS generation. Virulence. 2020;11(1):537–553. doi: 10.1080/21505594.2020.1766790
- Martinon F, Mayor A, Tschopp J. The inflammasomes: guardians of the body. Annu Rev Immunol. 2009;27(1):229–265. doi: 10.1146/annurev.immunol.021908.132715
- Akira S, Uematsu S, Takeuchi O. Pathogen recognition and innate immunity. Cell. 2006;124(4):783–801. doi: 10.1016/j.cell.2006.02.015
- García-Sastre A. Ten Strategies of interferon evasion by viruses. Cell Host Microbe. 2017;22(2):176–184. doi: 10.1016/j.chom.2017.07.012
- Wang W, Xiao F, Wan P, et al. EV71 3D protein binds with NLRP3 and enhances the assembly of inflammasome complex. PLoS Path. 2017;13(1):e1006123. doi: 10.1371/journal.ppat.1006123
- Liu G, Lee J-H, Parker ZM, et al. ISG15-dependent activation of the sensor MDA5 is antagonized by the SARS-CoV-2 papain-like protease to evade host innate immunity. Nat Microbiol. 2021;6(4):467–478. doi: 10.1038/s41564-021-00884-1
- Kato H, Takeuchi O, Sato S, et al. Differential roles of MDA5 and RIG-I helicases in the recognition of RNA viruses. Nature. 2006;441(7089):101–105. doi: 10.1038/nature04734
- Kuo R-L, Chen C-J, Wang Robert YL, et al. Role of enteroviral RNA-dependent RNA polymerase in regulation of MDA5-mediated beta interferon activation. J Virol. 2019;93(10):e00132–19. doi: 10.1128/JVI.00132-19
- Yang Z, Zheng H, Li H, et al. The expression of IFN-β is suppressed by the viral 3D polymerase via its impact on PGAM5 expression during enterovirus D68 infection. Virus Res. 2021;304:198549. doi: 10.1016/j.virusres.2021.198549
- Tan C, Qin X, Tan Y, et al. SHFL inhibits enterovirus A71 infection by triggering degradation of viral 3Dpol protein via the ubiquitin–proteasome pathway. J Med Virol. 2023;95(8):e29030. doi: 10.1002/jmv.29030
- Li L, Bai J, Fan H, et al. E2 ubiquitin-conjugating enzyme UBE2L6 promotes senecavirus a proliferation by stabilizing the viral RNA polymerase. PLoS Path. 2020;16(10):e1008970. doi: 10.1371/journal.ppat.1008970
- Lawrence P, Schafer EA, Rieder E. The nuclear protein Sam68 is cleaved by the FMDV 3C protease redistributing Sam68 to the cytoplasm during FMDV infection of host cells. Virology. 2012;425(1):40–52. doi: 10.1016/j.virol.2011.12.019
- Marsh K, Soros V, Cochrane A. Selective translational repression of HIV-1 RNA by Sam68DeltaC occurs by altering PABP1 binding to unspliced viral RNA. Retrovirology. 2008;5(1):97. doi: 10.1186/1742-4690-5-97
- McBride AE, Schlegel A, Kirkegaard K. Human protein Sam68 relocalization and interaction with poliovirus RNA polymerase in infected cells. Proc Natl Acad Sci U S A. 1996;93(6):2296–2301. doi: 10.1073/pnas.93.6.2296
- Rai DK, Lawrence P, Kloc A, et al. Analysis of the interaction between host factor Sam68 and viral elements during foot-and-mouth disease virus infections. Virol J. 2015;12(1):224. doi: 10.1186/s12985-015-0452-8
- Choudhury SM, Ma X, Zeng Z, et al. Senecavirus a 3D interacts with NLRP3 to induce IL-1β production by activating NF-κB and ion channel signals. Microbiol Spectr. 2022;10(2):e02097–21. doi: 10.1128/spectrum.02097-21
- Zhou Y, Wu W, Xie L, et al. Cellular RNA helicase DDX1 is involved in transmissible gastroenteritis virus nsp14-induced interferon-beta production. Front Immunol. 2017;8. doi: 10.3389/fimmu.2017.00940
- Edgcomb SP, Carmel AB, Naji S, et al. DDX1 is an RNA-dependent ATPase involved in HIV-1 rev function and virus replication. J Mol Biol. 2012;415(1):61–74. doi: 10.1016/j.jmb.2011.10.032
- Xue Q, Liu H, Zeng Q, et al. The DEAD-Box RNA helicase DDX1 interacts with the viral protein 3D and inhibits foot-and-mouth disease virus replication. Virol Sin. 2019;34(6):610–617. doi: 10.1007/s12250-019-00148-7
- Sarry M, Caignard G, Dupré J, et al. Host-specific interplay between foot-and-mouth disease virus 3D polymerase and the type-I interferon pathway. Viruses. 2023;15(3):666. doi: 10.3390/v15030666
- Wang J, Hu Y, Zheng M. Enterovirus A71 antivirals: past, present, and future. Acta Pharm Sin B. 2022;12(4):1542–1566. doi: 10.1016/j.apsb.2021.08.017
- Hu Y, Musharrafieh R, Zheng M, et al. Enterovirus D68 antivirals: past, present, and future. ACS Infect Dis. 2020;6(7):1572–1586. doi: 10.1021/acsinfecdis.0c00120
- Laajala M, Reshamwala D, Marjomäki V. Therapeutic targets for enterovirus infections. Expert Opin Ther Targets. 2020;24(8):745–757. doi: 10.1080/14728222.2020.1784141
- Liang C, Tian L, Liu Y, et al. A promising antiviral candidate drug for the COVID-19 pandemic: a mini-review of remdesivir. Eur J Med Chem. 2020;201:112527. doi: 10.1016/j.ejmech.2020.112527
- González-Grande R, Jiménez-Pérez M, González Arjona C, et al. New approaches in the treatment of hepatitis C. World J Gastroenterol. 2016;22(4):1421–1432. doi: 10.3748/wjg.v22.i4.1421
- Crotty S, Maag D, Arnold JJ, et al. The broad-spectrum antiviral ribonucleoside ribavirin is an RNA virus mutagen. Nat Med. 2000;6(12):1375–1379. doi: 10.1038/82191
- Nikunjkumar P, Tamil Selvan RP, Bhanuprakash V. Ribavirin as a curative and prophylactic agent against foot and mouth disease virus infection in C57BL/6 suckling and adult mice model. Virusdisease. 2021;32(4):737–747. doi: 10.1007/s13337-021-00746-8
- Graci Jason D, Harki Daniel A, Korneeva Victoria S, et al. Lethal mutagenesis of poliovirus mediated by a mutagenic pyrimidine analogue. J Virol. 2007;81(20):11256–11266. doi: 10.1128/JVI.01028-07
- Graci JD, Too K, Smidansky ED, et al. Lethal mutagenesis of picornaviruses with N-6-modified purine nucleoside analogues. Antimicrob Agents Chemother. 2008;52(3):971–979. doi: 10.1128/AAC.01056-07
- Harki DA, Graci JD, Galarraga JE, et al. Synthesis and antiviral activity of 5-substituted cytidine analogues: identification of a potent inhibitor of viral RNA-dependent RNA polymerases. J Med Chem. 2006;49(21):6166–6169. doi: 10.1021/jm060872x
- Yin Z, Chen Y-L, Schul W, et al. An adenosine nucleoside inhibitor of dengue virus. Proc Natl Acad Sci U S A. 2009;106(48):20435–20439. doi: 10.1073/pnas.0907010106
- Deng C-L, Yeo H, Ye H-Q, et al. Inhibition of enterovirus 71 by adenosine analog NITD008. J Virol. 2014;88(20):11915–11923. doi: 10.1128/JVI.01207-14
- Shang L, Wang Y, Qing J, et al. An adenosine nucleoside analogue NITD008 inhibits EV71 proliferation. Antiviral Res. 2014;112:47–58. doi: 10.1016/j.antiviral.2014.10.009
- Yan L, Cao R, Zhang H, et al. Design, synthesis and evaluation of 2’-acetylene-7-deaza-adenosine phosphoamidate derivatives as anti-EV71 and anti-EV-D68 agents. Eur J Med Chem. 2021;226:113852. doi: 10.1016/j.ejmech.2021.113852
- Zhang Z, Yang E, Hu C, et al. Cell-based high-throughput screening assay identifies 2‘,2’-difluoro-2’-deoxycytidine gemcitabine as a potential antipoliovirus agent. ACS Infect Dis. 2017;3(1):45–53. doi: 10.1021/acsinfecdis.6b00116
- Zheng L, Wang Q, Yang X, et al. Antiviral activity of Fnc, 2’-deoxy-2’-β-fluoro-4’-azidocytidine, against human and duck HBV replication. Antivir Ther. 2012;17(4):679–687. doi: 10.3851/IMP2094
- Wang R-R, Yang Q-H, Luo R-H, et al. Azvudine, a novel nucleoside reverse transcriptase inhibitor showed good drug combination features and better inhibition on drug-resistant strains than lamivudine in vitro. PLoS One. 2014;9(8):e105617. doi: 10.1371/journal.pone.0105617
- Xu N, Yang J, Zheng B, et al. The pyrimidine analog FNC potently inhibits the replication of multiple enteroviruses. J Virol. 2020;94(9):e00204–20. doi: 10.1128/JVI.00204-20
- Brown AJ, Won JJ, Graham RL, et al. Broad spectrum antiviral remdesivir inhibits human endemic and zoonotic deltacoronaviruses with a highly divergent RNA dependent RNA polymerase. Antiviral Res. 2019;169:104541. doi: 10.1016/j.antiviral.2019.104541
- Warren TK, Jordan R, Lo MK, et al. Therapeutic efficacy of the small molecule GS-5734 against Ebola virus in rhesus monkeys. Nature. 2016;531(7594):381–385. doi: 10.1038/nature17180
- Ye W, Yao M, Dong Y, et al. Remdesivir (GS-5734) impedes enterovirus replication through viral RNA synthesis inhibition. Front Microbiol. 2020;11:1105. doi: 10.3389/fmicb.2020.01105
- Bermingham WH, Canning B, Wilton T, et al. Case report: clearance of longstanding, immune-deficiency-associated, vaccine-derived polio virus infection following remdesivir therapy for chronic SARS-CoV-2 infection. Front Immunol. 2023;14:1135834. doi: 10.3389/fimmu.2023.1135834
- Nishi T, Fukai K, Masujin K, et al. Administration of the antiviral agent T-1105 fully protects pigs from foot-and-mouth disease infection. Antiviral Res. 2022;208:105425. doi: 10.1016/j.antiviral.2022.105425
- Furuta Y, Gowen BB, Takahashi K, et al. Favipiravir (T-705), a novel viral RNA polymerase inhibitor. Antiviral Res. 2013;100(2):446–454. doi: 10.1016/j.antiviral.2013.09.015
- Lumby CK, Zhao L, Oporto M, et al. Favipiravir and zanamivir cleared infection with influenza B in a severely immunocompromised child. Clin Infect Dis. 2020;71(7):e191–e194. doi: 10.1093/cid/ciaa023
- Sasaki-Tanaka R, Shibata T, Okamoto H, et al. Favipiravir inhibits hepatitis a virus infection in human hepatocytes. Int J Mol Sci. 2022;23(5):2631. doi: 10.3390/ijms23052631
- Kempf Brian J, Watkins Colleen L, Peersen Olve B, et al. Picornavirus RNA recombination counteracts error catastrophe. J Virol. 2019;93(14):e00652–19. doi: 10.1128/JVI.00652-19
- Sierra S, Dávila M, Lowenstein PR, et al. Response of foot-and-mouth disease virus to increased mutagenesis: influence of viral load and fitness in loss of infectivity. J Virol. 2000;74(18):8316–8323. doi: 10.1128/JVI.74.18.8316-8323.2000
- Pariente N, Airaksinen A, Domingo E. Mutagenesis versus inhibition in the efficiency of extinction of foot-and-mouth disease virus. J Virol. 2003;77(12):7131–7138. doi: 10.1128/JVI.77.12.7131-7138.2003
- Agudo R, Arias A, Pariente N, et al. Molecular characterization of a dual inhibitory and mutagenic activity of 5-fluorouridine triphosphate on viral RNA synthesis. Implications for lethal mutagenesis. J Mol Biol. 2008;382(3):652–666. doi: 10.1016/j.jmb.2008.07.033
- Farhat A, Malecki E, Bonaterra GA, et al. Cytostatic/cytotoxic effects of 5-fluorouridine nucleolipids on colon, hepatocellular, and renal carcinoma cells: In vitro identification of a potential cytotoxic multi-anticancer drug. Chem Biodivers. 2014;11(3):469–482. doi: 10.1002/cbdv.201300347
- Graci JD, Cameron CE. Mechanisms of action of ribavirin against distinct viruses. Rev Med Virol. 2006;16(1):37–48. doi: 10.1002/rmv.483
- De la Torre JC, Alarcón B, Martínez-Salas E, et al. Ribavirin cures cells of a persistent infection with foot-and-mouth disease virus in vitro. J Virol. 1987;61(1):233–235. doi: 10.1128/jvi.61.1.233-235.1987
- Choi JH, Jeong K, Kim SM, et al. Synergistic effect of ribavirin and vaccine for protection during early infection stage of foot-and-mouth disease. J Vet Sci. 2018;19(6):788–797. doi: 10.4142/jvs.2018.19.6.788
- Crotty S, Cameron CE, Andino R. RNA virus error catastrophe: direct molecular test by using ribavirin. Proc Natl Acad Sci U S A. 2001;98(12):6895–6900. doi: 10.1073/pnas.111085598
- Vignuzzi M, Stone JK, Andino R. Ribavirin and lethal mutagenesis of poliovirus: molecular mechanisms, resistance and biological implications. Virus Res. 2005;107(2):173–181. doi: 10.1016/j.virusres.2004.11.007
- Wu R, Smidansky ED, Oh HS, et al. Synthesis of a 6-methyl-7-deaza analogue of adenosine that potently inhibits replication of polio and dengue viruses. J Med Chem. 2010;53(22):7958–7966. doi: 10.1021/jm100593s
- Carroll SS, Tomassini JE, Bosserman M, et al. Inhibition of hepatitis C virus RNA replication by 2’-modified nucleoside analogs. J Biol Chem. 2003;278(14):11979–11984. doi: 10.1074/jbc.M210914200
- Goris N, De Palma A, Toussaint J-F, et al. 2’-C-methylcytidine as a potent and selective inhibitor of the replication of foot-and-mouth disease virus. Antiviral Res. 2007;73(3):161–168. doi: 10.1016/j.antiviral.2006.09.007
- Lanko K, Ma Y, Delang L, et al. Antiviral effects of selected nucleoside analogues against human parechoviruses A1 and A3. Antiviral Res. 2019;162:51–53. doi: 10.1016/j.antiviral.2018.12.009
- Masmoudi F, Santos-Ferreira N, Pajkrt D, et al. Evaluation of 3D human intestinal organoids as a platform for EV-A71 antiviral drug discovery. Cells. 2023;12(8):1138. doi: 10.3390/cells12081138
- Harrison DN, Gazina EV, Purcell DF, et al. Amiloride derivatives inhibit coxsackievirus B3 RNA replication. J Virol. 2008;82(3):1465–1473. doi: 10.1128/JVI.01374-07
- Gazina EV, Smidansky ED, Holien JK, et al. Amiloride is a competitive inhibitor of coxsackievirus B3 RNA polymerase. J Virol. 2011;85(19):10364–10374. doi: 10.1128/JVI.05022-11
- Ogram SA, Boone CD, McKenna R, et al. Amiloride inhibits the initiation of coxsackievirus and poliovirus RNA replication by inhibiting VPg uridylylation. Virology. 2014;464-465:87–97. doi: 10.1016/j.virol.2014.06.025
- Holien JK, Gazina EV, Elliott RW, et al. Computational analysis of amiloride analogue inhibitors of coxsackievirus B3 RNA polymerase. J Proteomics Bioinform. 2014;s9(Suppl 9):004. doi: 10.4172/jpb.S9-004
- Levi LI, Gnädig NF, Beaucourt S, et al. Fidelity variants of RNA dependent RNA polymerases uncover an indirect, mutagenic activity of amiloride compounds. PLoS Path. 2010;6(10):e1001163. doi: 10.1371/journal.ppat.1001163
- Gong M-J, Chang Y-Y, Shao J, et al. Antiviral effect of amiloride on replication of foot and mouth disease virus in cell culture. Microbial Pathogenesis. 2019;135:103638. doi: 10.1016/j.micpath.2019.103638
- Chern J-H, Shia K-S, Hsu T-A, et al. Design, synthesis, and structure-activity relationships of pyrazolo[3,4-d]pyrimidines: a novel class of potent enterovirus inhibitors. Bioorg Med Chem Lett. 2004;14(10):2519–2525. doi: 10.1016/j.bmcl.2004.02.092
- Chen T-C, Chang H-Y, Lin P-F, et al. Novel antiviral agent DTriP-22 targets RNA-dependent RNA polymerase of enterovirus 71. Antimicrob Agents Chemother. 2009;53(7):2740–2747. doi: 10.1128/AAC.00101-09
- Hung H-C, Chen T-C, Fang M-Y, et al. Inhibition of enterovirus 71 replication and the viral 3D polymerase by aurintricarboxylic acid. J Antimicrob Chemother. 2010;65(4):676–683. doi: 10.1093/jac/dkp502
- Durk RC, Singh K, Cornelison CA, et al. Inhibitors of foot and mouth disease virus targeting a novel pocket of the RNA-dependent RNA polymerase. PloS One. 2010;5(12):e15049. doi: 10.1371/journal.pone.0015049
- Rai DK, Schafer EA, Singh K, et al. Repeated exposure to 5D9, an inhibitor of 3D polymerase, effectively limits the replication of foot-and-mouth disease virus in host cells. Antiviral Res. 2013;98(3):380–385. doi: 10.1016/j.antiviral.2013.03.022
- van der Linden L, Vives-Adrián L, Selisko B, et al. The RNA template channel of the RNA-dependent RNA polymerase as a target for development of antiviral therapy of multiple genera within a virus family. PloS Path. 2015;11(3):e1004733. doi: 10.1371/journal.ppat.1004733
- Li L, Wang M, Chen Y, et al. Structure of the enterovirus D68 RNA-dependent RNA polymerase in complex with NADPH implicates an inhibitor binding site in the RNA template tunnel. J Struct Biol. 2020;211(1):107510. doi: 10.1016/j.jsb.2020.107510
- Campagnola G, Gong P, Peersen OB. High-throughput screening identification of poliovirus RNA-dependent RNA polymerase inhibitors. Antiviral Res. 2011;91(3):241–251. doi: 10.1016/j.antiviral.2011.06.006
- Hsu J-A, Yeh J-Y, Lin T-J, et al. Identification of BPR3P0128 as an inhibitor of cap-snatching activities of influenza virus. Antimicrob Agents Chemother. 2012;56(2):647–657. doi: 10.1128/AAC.00125-11
- Velu AB, Chen G-W, Hsieh P-T, et al. BPR-3P0128 inhibits RNA-dependent RNA polymerase elongation and VPg uridylylation activities of enterovirus 71. Antiviral Res. 2014;112:18–25. doi: 10.1016/j.antiviral.2014.10.003
- Wishart DS, Feunang YD, Guo AC, et al. DrugBank 5.0: a major update to the DrugBank database for 2018. Nucleic Acids Res. 2018;46(D1):D1074–D1082. doi: 10.1093/nar/gkx1037
- Theerawatanasirikul S, Lueangaramkul V, Semkum P, et al. Antiviral mechanisms of sorafenib against foot-and-mouth disease virus via c-RAF and AKT/PI3K pathways. Vet Res Commun. 2023;48(1):329–343. doi: 10.1007/s11259-023-10211-0
- Liu X, Xu Z, Liang J, et al. Identification of a novel acylthiourea-based potent broad-spectrum inhibitor for enterovirus 3D polymerase in vitro and in vivo. Antiviral Res. 2023;213:105583. doi: 10.1016/j.antiviral.2023.105583
- Jeong K-W, Lee J-H, Park S-M, et al. Synthesis and in-vitro evaluation of 2-amino-4-arylthiazole as inhibitor of 3D polymerase against foot-and-mouth disease (FMD). European Journal Of Medicinal Chemistry. 2015;102:387–397. doi: 10.1016/j.ejmech.2015.08.020
- Theerawatanasirikul S, Semkum P, Lueangaramkul V, et al. Non-nucleoside inhibitors decrease foot-and-mouth disease virus replication by blocking the viral 3Dpol. Viruses. 2023;15(1):124. doi: 10.3390/v15010124
- Lanko K, Shi C, Patil S, et al. Assessing in vitro resistance development in enterovirus A71 in the context of combination antiviral treatment. ACS Infect Dis. 2021;7(10):2801–2806. doi: 10.1021/acsinfecdis.0c00872
- Hu Q, Sun W, Wang C, et al. Recent advances of cocktail chemotherapy by combination drug delivery systems. Adv Drug Deliv Rev. 2016;98:19–34. doi: 10.1016/j.addr.2015.10.022