ABSTRACT
Staphylococcus aureus (S. aureus) is well known for its biofilm formation ability and is responsible for serious, chronic refractory infections worldwide. We previously demonstrated that advanced glycation end products (AGEs), a hallmark of chronic hyperglycaemia in diabetic tissues, enhanced biofilm formation by promoting eDNA release via sigB upregulation in S. aureus, contributing to the high morbidity and mortality of patients presenting a diabetic foot ulcer infection. However, the exact regulatory network has not been completely described. Here, we used pull-down assay and LC-MS/MS to identify the GlmS as a candidate regulator of sigB in S. aureus stimulated by AGEs. Dual-luciferase assays and electrophoretic mobility shift assays (EMSAs) revealed that GlmS directly upregulated the transcriptional activity of sigB. We constructed NCTC 8325 ∆glmS for further validation. qRT-PCR analysis revealed that AGEs promoted both glmS and sigB expression in the NCTC 8325 strain but had no effect on NCTC 8325 ∆glmS. NCTC 8325 ∆glmS showed a significant attenuation in biofilm formation and virulence factor expression, accompanied by a decrease in sigB expression, even under AGE stimulation. All of the changes, including pigment deficiency, decreased haemolysis ability, downregulation of hla and hld expression, and less and sparser biofilms, indicated that sigB and biofilm formation ability no longer responded to AGEs in NCTC 8325 ∆glmS. Our data extend the understanding of GlmS in the global regulatory network of S. aureus and demonstrate a new mechanism by which AGEs can upregulate GlmS, which directly regulates sigB and plays a significant role in mediating biofilm formation and virulence factor expression.
Introduction
Staphylococcus aureus (S. aureus) is a commensal gram-positive pathogen that causes a range of infections in humans, from skin infections to life-threatening illnesses such as pneumonia, osteomyelitis, and sepsis [Citation1]. S. aureus infections are more challenging to treat than non-biofilm-forming bacteria because of biofilm formation [Citation2].
Diabetic foot ulcer infection (DFI) is a notorious consequence of S. aureus infection and biofilm formation [Citation3]. As the predominant pathogen involved in the pathogenesis of DFI, S. aureus produces multiple virulence factors and forms biofilms, making the infection difficult to cure and leading to the high morbidity and mortality of patients presenting a diabetic foot ulcer infection [Citation4]. The specific host factors of diabetic patients act as environmental stimuli, affecting S. aureus sessile biofilm growth. Advanced glycation end products (AGEs), a hallmark of chronic hyperglycaemia in diabetic tissues, have received increasing attention in recent years for their damaging role in diabetic pathogenesis [Citation5–7]. AGEs are formed by the Maillard reaction, which occurs in an irreversible manner between amine group compounds (proteins, lipids, and nucleic acids) and carboxides (reducing sugar groups). Once formed, AGEs can continuously accumulate in biological tissues and produce incessant effects on the cells and microorganisms in the wound, even if blood sugar levels are well controlled. We previously demonstrated that AGEs significantly enhanced the biofilm formation of S. aureus by increasing extracellular DNA release. Global regulator sigma factor σB (SigB) plays an important role in the promotion of biofilm formation by AGEs through the downstream factor lrg operons [Citation8]. Nevertheless, the regulatory network underlying biofilm formation by S. aureus is extremely complicated and poorly understood. Despite the unambiguous role of sigB activation by AGEs in biofilm formation, how AGEs act on sigB is not known.
In this work, we show that AGEs do not directly regulate sigB but instead activate the expression of glmS. To identify candidate regulators of sigB under AGE stimulation, we used pull-down assays and LC-MS/MS to show that the most significantly different protein pulled down by the SigB promoter probe was the glucosamine-6-phosphate synthetase (GlmS) protein. The dual-luciferase assay and electrophoretic mobility shift assay (EMSA) analysis confirmed that GlmS directly upregulates the transcriptional activity of sigB. We used quantitative RT-PCR (qRT-PCR) to show that AGEs promoted the expression of glmS, and the strains lacking glmS failed to highly express sigB or form biofilms in the presence of AGEs, indicating that GlmS and sigB constitute a regulatory pathway.
GlmS, discovered in 1991 by Baev et al. [Citation9], is generally known as a ribozyme that can catalyse the conversion of fructose-6-phosphate and glutamine into glutamate and glucosamine-6-phosphate (GlcN6P), the starting point for bacterial cell wall synthesis. Aggregated GlcN6P can bind to the glmS ribozyme domain, activate latent self-cleavage activity, and degrade glmS mRNA, which is the foundation of glmS riboswitches [Citation10]. This negative-feedback loop has become a new biomolecular target for the development of antibiotics and chemical/biological tools [Citation11]. Previous studies regarding glmS of S. aureus have therefore focused on cell wall synthesis and drug resistance, along with the discovery of new riboswitch activators [Citation12,Citation13]. This study revealed another important role of GlmS involving global regulation through sigB, including biofilm formation and virulence factors. This is the first study to identify the crucial role of GlmS in the virulence factor expression of S. aureus and a new potential activator, AGEs. Consequently, another new regulatory pathway involving sigB activity has been found, which has improved the understanding of the global regulatory network of S. aureus.
Materials and methods
Bacterial strains, strain culture, and plasmid construction
S. aureus NCTC 8325, a type strain of S. aureus, was obtained from the China Medical Culture Collection Center (CMCC) and stored at −80°C. Escherichia coli DH5α was purchased from Solarbio, China (C1100) and used for plasmid construction.
NCTC 8325 colony was incubated overnight at 37°C with TSB broth in a shaker rotational speed of 200 r/min. All experiments were set up with experimental groups and blank groups. The experimental groups were stimulated with 50 µg/mL AGEs in TSB broth and cultured for 24 h. AGEs were purchased from Abcam, USA (ab51995). The blank groups were stimulated with the same concentration of bovine serum albumin (BSA) (EZ7890B203, BioFroxx, Germany).
The SigB promoter sequence was transferred into pGL4.10 vector (YouBio, China, VT1558) by restriction endonuclease sites XhoI and HindIII to express SigB promoter. The glmS gene CDS region was transferred into pcDNA3.1 and pET-28a vectors (YouBio, China, VT1001, VT1207) by restriction endonuclease sites XhoI and BamH I to express GlmS protein. The recombination products were transformed into competent E. coli DH5α cells and BL21(DE3) cells. Recombinant positive plasmids of pGL4.10 and pcDNA3.1 vectors were screened through ampicillin containing medium, while recombinant positive plasmids of pET-28a vector were screened through kanamycin containing medium.
Pull-down and LC-MS/MS analysis
The pull-down assay utilized the natural affinity of streptomycin and biotin and was performed to analyse the interaction between DNA and proteins as described previously [Citation14,Citation15]. The SigB promoter was amplified by PCR using primers F (5’-CAGCTATGACCATGATTACGAATTCTTGCAAACGACAAAATTGATAAGTGCAATTAAATAAATGTTAGTAAG-3’) and R (5’-GTAAAACGACGGCCAGTGCCAAGCTTTATTTCGCACCTGCTCTTTTTTTATATACTTAGTCATACTGAT-3’). About 800 μl of nucleoprotein extracted from NCTC 8325 was premixed with 4 μg of biotin-labelled SigB promoter probe (Figure S2 and S3) and then 40 μl of streptavidin-agarose beads (Sigma, USA) were added and rotated at 4°C for 2 h. The beads were washed four times to collect pulled-down proteins and then boiled at 100°C for 10 min for further SDS-PAGE analysis. The protein bands in SDS-PAGE gel were visualized by silver staining. Shotgun proteomics, one of LC-MS/MS analysis (Thermo ScientificTM Q ExactiveTM, USA) was chosen to identify two groups of pulled-down proteins, and the protein peak maps were compared in the UniProt database (https://www.uniprot.org/taxonomy/1280). The mass spectrometry proteomics data have been deposited to the ProteomeXchange Consortium via the PRIDE [Citation16] partner repository with the dataset identifier PXD046076. Gene Ontology (GO) functional analysis and Kyoto Encyclopedia of Genes and Genomes (KEGG) pathway analysis were performed on the differential proteins to help select candidate protein.
Transfection and dual-luciferase reporter assay
The plasmid expressing the SigB promoter (Figure S4) was constructed using pGL4.10 vector, which can also express luciferase. The plasmid expressing the GlmS protein (Figure S5) was constructed using pcDNA3.1 vector. HEK-293 T cells (ATCC®CRL-1573TM) were prepared with trypsin in 24-well plates before transfection [Citation17]. After 24 h of incubation at 37°C, the cell confluency rate was estimated to be approximately 70–80%. The SigB promoter plasmid, GlmS plasmid, and Hieff Trans™ liposomal transfection reagent (40802ES03, Yeasen Biotech, China) were mixed in serum-free DMEM (PM150210, Procell, China) according to the transfection reagent manufacturer’s protocol. After thorough mixing, the mixture was incubated for 10–25 min at room temperature to form the DNA-Hieff TransTM complex. Then, the DNA-Hieff TransTM complex was transfected into prepared HEK 293T cells after another 48 h of incubation [Citation17]. Putative binding function and the regulation effect of GlmS protein were determined using the Dual-Glo® Luciferase Assay system (Promega, USA) according to the manufacturer’s protocol in 96-well plates. GloMax® (Promega, USA) was used to measure the activity of firefly luciferase and Renilla luciferase. The ratio of luminescence from firefly luciferase to Renilla luciferase was calculated [Citation18,Citation19].
Electrophoretic mobility shift assay (EMSA)
EMSA was performed according to previous studies with some modifications [Citation20]. BL21(DE3) cells were transfected with the GlmS plasmid. The expressed proteins were extracted and purified using a Ni-NTA 6FF SefinoseTM Resin Kit (C600332, Sangon Biotech, China). The purified GlmS protein concentration was 0.75 mg/ml for subsequent experiments. The 400 bp positive SigB promoter fragment in the dual-luciferase assay was truncated into four segments of 100 bp. Each 100 bp fragment was biotinylated as an EMSA probe. EMSA was performed using a chemiluminescent EMSA kit (Axl-EMSA-100, Axl-Bio, China) according to the manufacturer’s instructions. A total of 0.1 pmol of biotinylated probe was added to each reaction. Additionally, 3.75 μg of purified protein was used per reaction when needed. The complexes obtained were separated by electrophoresis on a 5% polyacrylamide gel and then electrophoretically transferred onto nylon membranes. A 254 nm UV wavelength of 120 mJ/cm [Citation2] was selected, and crosslinking was performed for 60 s using a UV-light crosslinker (SCIENTZ 03-II, SCIENTZ, China). The bands were visualized using chemiluminescence detection.
Mutant construction
S. aureus NCTC 8325 is an ideal S. aureus strain for genetic manipulation [Citation21]. To further illustrate the role of GlmS in biofilm formation, a glmS-knockout mutant (NCTC 8325 ΔglmS) was constructed with pKOR1 plasmid through homologous recombination using seamless cloning [Citation22]. Two pairs of primers (glmS-pKOR1-F/glmS-pKOR1-R, pKOR1-glmS-F/pKOR1-glmS-R) were designed for PCR amplification. Then, glmS- pKOR1 products and pKOR1-glmS products were seamlessly connected and transformed into E. coli DH5α cells. Knockout plasmid was transferred to RN4220 using 2.5kV electricity. RN4220glmS-pKOR1 was transferred to NCTC 8325 through phage transduction method. Finally, the loss of glmS gene was confirmed by PCR using primers glmS-JD-F/glmS-JD-R and glmS-ter-F/glmS-ter-R. The PCR product electropherograms are shown in Figure S6. All the sequences of primers were listed in Table S7. During the culture process, 50 mM of N-acetyl-D-aminoglucose (GlcNAc) was added to the culture medium [Citation23].
To comprehensively illustrate the role of GlmS in biofilm formation, a glmS overexpressing strain was constructed with pCM plasmid (BIO SCI, China). Primers F (5’-TTAGGAGGATGATTATTAATGTGTGGAATTGTTGGTTATA-3’) and R (5’-GTGGTGGTGGTGGTGGTGTTCCACAGTAACTGATTTAGC-3’) were designed to amplify target gene glmS. Then, amplify overexpression vector framework using primers F (5’-CACCACCACCACCACCACTGAATTCGTAATCATGTCATAG-3’) and R (5’-ACCAACAATTCCACACATTAATAATCATCCTCCTAAGGTA-3’). Two PCR products are seamlessly cloned and then transferred into E. coli dc10b competent cells. The recombinant positive plasmids were screened through chloramphenicol containing medium. Finally, the overexpressing plasmid is transferred back to S. aureus NCTC 8325 using 2.3kV electricity. SDS-PAGE was used to confirm the overexpression of the GlmS protein (Figure S7).
RNA extraction and qRT-PCR
Total RNA was extracted from S. aureus under different culture conditions using TRIzol reagent (Invitrogen, USA) and ultrasonic waves. The quality and quantity of RNA were detected by a Nanodrop 2000 spectrophotometer (Thermo Fisher Scientific, USA) [Citation24]. cDNA was first synthesized using a HyperScriptTM First-Strand cDNA Synthesis Kit (APExBIO, USA) and detected with HotStartTM 2×SYBR Green qPCR Master Mix (APExBIO, USA). Each step referred to the kit manufacturer’s protocol. qRT-PCR was performed to detect the expression of global regulators of biofilm formation (sigB) and the expression of haemolysin genes (hla, hlb, hld, hlg) in S. aureus strains. The primers are listed in Supplementary Table S6. The relative expression levels of the tested genes were normalized to that of 16S rRNA [Citation20].
Hemolysis assay
The haemolytic ability assessment was based on the description of Jiang et al. [Citation25] with minor modifications. In brief, NCTC 8325 and NCTC 8325 ∆glmS strains were incubated at 37°C for 24 h at a rotation speed of 200 rpm overnight. After centrifugation at 10,000 rpm for 8 min, the supernatant was collected. A 6% human erythrocyte solution was used to evaluate the haemolysis ability of the strains. Then, 100 μl of supernatant was added to 900 μl of erythrocyte solution, incubated at 37°C for 30 min, and centrifuged at 2500 rpm for 10 min. TSB was used as a negative control, and 0.1% Triton-X 100 was used as a positive control. The absorbance of the supernatant (OD405) was measured using a MultiskanTM FC Microplate Photometer (Thermo Fisher Scientific, USA).
Growth curves
The bacterial growth curve was determined as previously described, with minor modifications [Citation26,Citation27]. Briefly, NCTC 8325 and NCTC 8325 ∆glmS were inoculated into TSB broth. A blank group and an experimental group were set up for each strain as described before. Then, the culture medium was incubated at 37°C and 200 rpm. The growth curves were constructed based on the OD620 at 0, 2, 4, 6, 8, 10, 12, 14, and 24 h.
Quantification of biofilms
Biofilm formation was performed according to previous studies [Citation28,Citation29]. Briefly, the overnight bacterial culture was prepared to a turbidity of 0.5 McFarland standards. A 20 µl bacterial suspension and 180 µl TSB medium were added to a 96-well flat-bottomed microtiter plate with lids in triplicate. After 24 h of incubation at 37°C, the wells were washed with PBS three times, stained with 0.5% (w/v) crystal violet (CV) for 10 min, and washed with water again. Then, the biofilm-associated CV dye was solubilized with 30% glacial acetic acid for 15 min at room temperature. The OD595 of each well was measured.
Confocal laser scanning microscopy (CLSM)
For CLSM analysis, S. aureus strains were cultured in confocal dishes according to previous studies [Citation28,Citation30]. After 24 h of incubation at 37°C, the nonadherent culture of each well was washed with PBS and stained with AO and EB solution (R20292, Yuanye, China) in a dark location for 15 min at room temperature. The stained biofilms were imaged via confocal microscopy (Olympus FV3000, Japan). The living bacteria were stained green, and the dead bacteria were stained orange.
Scanning electron microscopy (SEM) analysis
For SEM analysis, the S. aureus strain of interest was cultured in 6-well cell culture according to previous studies [Citation30,Citation31]. After 24 h of incubation, the biofilms were washed twice with PBS to remove nonadherent bacteria and fixed with 2.5% glutaraldehyde at 4°C for 4 h. Then, the biofilm samples were dehydrated and dried in a critical point dryer. After coating with gold powder, the biofilms were observed by scanning electron microscopy (Hitachi, Japan).
Statistical analysis
All data are representative of three independent experiments and expressed as the mean ± standard deviation (SD). Statistical significance was assessed using unpaired and paired two-tailed Student’s t test with GraphPad Prism 9.0 (USA). p < 0.05 was considered statistically significant. * p < 0.05, ** p < 0.01, *** p < 0.001, **** p < 0.0001, and ns represents no significance. Graphs were drawn with GraphPad Prism 9.0 and Adobe Illustrator (USA).
Results
GlmS is a candidate protein that can directly combined with SigB promoter stimulated by AGEs in S. aureus
The whole proteome and DNA pulled-down proteins of the NCTC 8325 strain were first separated by SDS-PAGE. The bands were visualized by silver staining to prove that we have pulled-down proteins for further identification and screening (Figure S1A). Then, shotgun proteomics LC-MS/MS analysis identified all of the pulled-down proteins, and we found 18 differential proteins (). GO functional analysis revealed that GlmS, a metabolite interconversion enzyme, has catalytic activity and participates in cellular processes and metabolic processes [Citation10,Citation32–34]. KEGG pathway analysis indicated that GlmS may be related to the N-acetylglucosamine metabolism pathway, which can affect the cell wall synthesis of S. aureus [Citation10,Citation34,Citation35]. Glms can recognize the small molecule in the environment, such as GlcN6P, and be activated to carry its physiologic functions [Citation36]. Finally, GlmS protein was selected as a candidate protein.
GlmS protein can directly bind to the SigB promoter and upregulate the transcriptional activity of sigB
The dual-luciferase assay can indirectly evaluate the transcriptional activity of the target gene by quantitating both firefly and Renilla luciferases, commonly used in research on promoter transcriptional activity regulation [Citation18,Citation20] and miRNA target gene validation [Citation37]. The result of dual-luciferase assay verifies that the GlmS protein can bind to the SigB promoter and upregulate its transcriptional activity (). To narrow down the binding sites between the two, the SigB promoter was divided evenly into five fragments. The dual-luciferase assay once again verified that GlmS protein can bind with the site 3 of SigB promoter and upregulate its transcriptional activity (). To further narrow down the binding sites between the two, site 3 was divided evenly into four fragments. GlmS protein was produced successfully and purified (), then used for EMSA. EMSA verified that probe 2 can bind to the GlmS protein (). Thus far, dual-luciferase reporter assay and EMSA determined the molecular mechanism by which GlmS protein can directly bind with the 100 bp fragment (5’-TTTTAACGGATGGTGTGACTGAAGCTAGAAATAGTGAAGGTACCTTTATAGATAAACAAAAACTTTTAGAATATATTAAAAAACATAAACATATGCACCC-3’) of SigB promoter and upregulate its transcriptional activity.
Figure 2. Verification of the binding sequence of the SigB promoter to the GlmS protein. (a) Luciferase activity of the SigB promoter in HEK-293T cells after cotransfection with GlmS-his. “vec” is short for “vector”. (b) Luciferase activity of five different fragments of the SigB promoter in HEK-293T cells after cotransfection with GlmS-his. (c) Vector map for expressing GlmS protein. (d) Left: SDS-PAGE electrophoresis of GlmS protein induced expression; right: SDS-PAGE electrophoresis of purified GlmS protein. The red arrow indicates the target protein, with a size of 72 KDa. (e) EMSA using different biotinylated probes of the SigB promoter to measure the binding ability of biotinylated probes with the GlmS protein. Lane 1, biotinylated probe 1 only; lane 2, biotinylated probe 2 only; lane 3, biotinylated probe 3 only; lane 4, biotinylated probe 4 only; lane 5, biotinylated probe 1 and GlmS protein; lane 6, biotinylated probe 2 and GlmS protein; lane 7, biotinylated probe 3 and GlmS protein; lane 8, biotinylated probe 4 and GlmS protein.
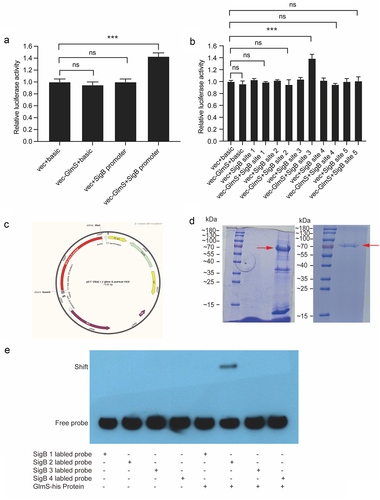
AGEs upregulate the mRNA expression of glmS
The relative quantification of mRNA expression of glmS under the promotion of BSA and AGEs was evaluated by the 2−∆∆CT method (). The average 2−∆∆CT value indicated that the mRNA expression of glmS gene increased by 3.28 times under the promotion of AGEs compared to BSA. Moreover, a statistical analysis of paired t-tests was performed on ∆CTs of the BSA and AGEs groups, and there was a significant difference between the two (p < 0.05).
Table 1. Relative quantification of the mRNA expression of glmS under the promotion of BSA and AGEs using the 2−∆∆Ct method.
*∆∆CT = (Average CT of AGEs) – (Average CT of BSA).
The glmS gene contributes to the nonpigmented phenotype, hemolytic phenotype, and cell wall synthesis of S. aureus
The NCTC 8325 strain has the typical colony morphology of S. aureus. The colony appears golden in colour, with a wide β haemolytic ring around the colony. However, NCTC 8325 ∆glmS lost its golden pigment, and the colony turned white (). Moreover, the haemolysis ability of NCTC 8325 also changed from β haemolysis to α haemolysis (), accompanied by a notable margin in haemolytic capacity (). In addition, the cell wall of NCTC 8325 ∆glmS showed a significant depression, as shown by the arrow (), which indicated once again that GlmS is the key ribozyme related to cell wall synthesis [Citation10].
Figure 3. The growth and hemolytic activity of NCTC 8325 and NCTC 8325 ∆glmS. (a) Colony morphology and hemolysis of NCTC 8325 and NCTC 8325 ∆glmS cultured on Columbia blood agar. (b) The hemolytic ability changed from β hemolysis to α hemolysis. (c) The hemolytic activity of NCTC 8325 and NCTC 8325 ∆glmS. TSB was used as the negative control. Triton-X 100 (0.1%) was used as a positive control. (d) Expression levels of hemolysin genes (hla, hlb, hld, hlg) in NCTC 8325 and NCTC 8325 ∆glmS determined by qRT-PCR. Relative gene expression was indicated by 16S rRNA gene normalization. * p < 0.05, ** p < 0.01, *** p < 0.001, and ns represents no significance. (E) The growth curve of NCTC 8325 and NCTC 8325 ∆glmS promoted by BSA and AGEs.
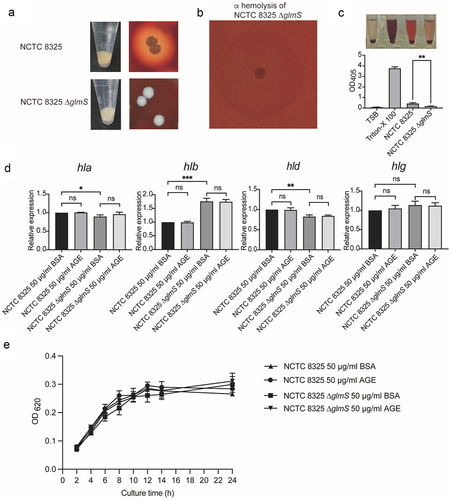
Figure 4. Biofilm formation and expression levels of sigB of NCTC 8325 and NCTC 8325 ∆glmS promoted by BSA and AGEs. (a) Crystal violet staining of the biofilms. (b) Expression levels of sigB in the NCTC 8325 and NCTC 8325 ∆glmS strains promoted by BSA and AGEs. (c) Images of biofilms obtained using CLSM and SEM. The living bacteria were stained green, and the dead bacteria were stained orange. * p < 0.05, ** p < 0.01, *** p < 0.001, **** p < 0.0001, and ns represents no significance. The white arrow indicates the cell wall depression of NCTC 8325 ∆glmS.
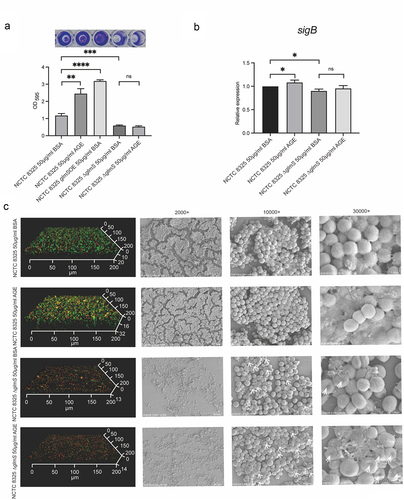
The growth curves of NCTC 8325 and NCTC 8325 ∆glmS demonstrated that BSA and AGEs do not impact their growth (), excluding the influence of growth factors on haemolysin gene expression and biofilm formation experiments.
The glmS gene contributes to changes in hemolysin gene expression in S. aureus
After observing changes in the haemolytic phenotype of the knockout strain (), we further detected the expression of haemolysin genes using qPCR. Compared with NCTC 8325, NCTC 8325 ∆glmS showed downregulation of hla and hld expression and upregulation of hlb expression. Meanwhile, AGEs had no promoting effect on the expression of haemolysin genes (). The changes in haemolysin gene expression caused decreased haemolysis ability of the NCTC 8325 strain (), which is a virulence factor of S. aureus.
AGEs cannot upregulate the expression of sigB and induce biofilm formation by NCTC 8325 ∆glmS
The biofilm mass was evaluated using crystal violet staining (). The biofilm mass of NCTC 8325 ∆glmS were significantly less than the wild strain, both in AGEs and BSA groups (p < 0.001). For NCTC 8325 strain, the biofilms formation in AGEs group was significantly higher than in the BSA group (p < 0.01). However, in NCTC 8325 ∆glmS strain, there was no significant difference between AGEs and BSA groups. The glmS overexpressing strain can promote the biofilm formation (p < 0.0001), similarly to the wild strain exposed to AGEs. Confocal laser scanning microscopy (CLSM) and scanning electron microscopy (SEM) showed the same results (). Confocal images showed that the biofilm of NCTC 8325 ∆glmS were obviously thinner and sparser than that of wild strain both in BSA (13 ± 1 µm vs. 20 ± 1 µm) and AGEs (14 ± 1 µm vs. 32 ± 1 µm) (p < 0.01) groups. The biofilm of NCTC 8325 strain promoted by AGEs (32 ± 1 µm) was significantly thicker and denser than the BSA group (20 ± 1 µm) (p < 0.05). While the biofilm thickness of NCTC 8325 ∆glmS has no significant difference between AGEs (14 ± 1 µm) and BSA (13 ± 1 µm) groups. SEM images show that the biofilm of NCTC 8325 ∆glmS was significantly reduced compared to the wild strain. AGEs can promote the biofilm formation of NCTC 8325 strain, but have no effect on NCTC 8325 ∆glmS. The expression of sigB was significantly reduced when the NCTC 8325 strain knocked out glmS gene (p < 0.05). The sigB expression of NCTC 8325 strain was upregulated in by AGEs (p < 0.05), while no change in NCTC 8325 ∆glmS strain ().
Discussion
In recent years, AGEs have attracted the attention of researchers due to increasing evidence of their involvement in many diseases, such as diabetes, cardiovascular diseases, cancers, neurodegenerative diseases, kidney diseases, liver diseases, infertility, infectious diseases, and even infection with the SARS-CoV-2 virus [Citation5–7,Citation38–40]. Our previous studies showed that the presence of AGEs, a reflection of hyperglycaemia in diabetic tissues, significantly promotes S. aureus biofilm formation via eDNA release. These effects are the consequence of upregulating sigB transcription and the subsequent downregulation of its downstream gene, lrgA [Citation8].
Alternative sigB, which primarily modulates the stress responses of several gram-positive bacteria, plays essential roles in regulating biofilm formation, virulence factor expression, and pigment synthesis in S. aureus [Citation1]. The sigB operon of S. aureus represents a global regulatory system that enables the organism to deal with environmental stresses [Citation29,Citation41]. AGEs, an important factor in the environment of diabetic foot tissue, upregulated sigB transcription, independent of the two-component systems Agr and SarA examined in our previous work. In this study, we further clarified that sigB is modulated by GlmS and enhances biofilm formation in S. aureus under AGE stimulation. To identify the regulator of sigB in the process of biofilm formation stimulated by AGEs, we used pull-down assays and LC-MS/MS to show that the most significantly different protein pulled down by the SigB promoter probe was the GlmS protein. The dual-luciferase assay and EMSA analysis confirmed that GlmS directly upregulates the transcriptional activity of sigB, and the binding site between the two was narrowed down to a 100 bp fragment (5’-TTTTAACGGATGGTGTGACTGAAGCTAGAAATAGTGAAGGTACCTTTATAGATAAACAAAAACTTTTAGAATATATTAAAAAACATAAACATATGCACCC-3’). To confirm the exact effect of biofilm formation, NCTC 8325 ∆glmS was used for biofilm formation experiments under AGE stimulation. We conducted a comprehensive and multilevel analysis on the biofilm formation of NCTC 8325 and NCTC 8325 ∆glmS under AGE stimulation, including the total amount of biofilm (), the thickness and vitality of the biofilms, and the density of the biofilms and the amount of extracellular matrix (). Compared with the wild-type strain, NCTC 8325 ∆glmS no longer responded to AGE stimulation in terms of biofilm formation. Concurrently, AGEs had no upregulation effect on sigB in NCTC 8325 ∆glmS, which indicated that GlmS plays a significant role in the S. aureus biofilm formation promoted by AGEs.
This discovery is very unexpected and interesting. To date, research on glmS has mainly focused on cell wall synthesis, drug resistance, and the development of antibiotic targets [Citation11,Citation32,Citation34], but no research has shown its roles in the global regulation and pathogenicity of S. aureus thus far. In previous studies, riboswitches have been classified as a family of 5’-untranslated mRNA regions mostly found in bacteria. The glmS riboswitch is unique among the family of riboswitches, as it is a ribozyme that undergoes self-cleavage upon binding to GlcN6P and is distributed in many important human pathogens, such as Bacillus anthracis, S. aureus, and Clostridium botulinum [Citation42]. Due to the close relationship with GlcN6P and cell wall synthesis, glmS is a very attractive target for developing broad-spectrum antibacterial compounds [Citation13,Citation34]. In our study, the growth curve of NCTC 8325 ∆glmS, cultured with 50 mM of GlcNAc remained unchanged compared to that of the wild-type strain (). However, it was also found that the cell wall of NCTC 8325 ∆glmS exhibited a depression (). The change in the cell wall is likely to cause changes in the drug sensitivity of a series of antibiotics that target the cell wall, such as penicillin, cephalosporin, vancomycin, bacitracin, and fosfomycin. In addition, we discovered the important role of GlmS in S. aureus pathogenicity. S. aureus produces many virulence factors involved in pathogenicity, which could be important in deciding infectious outcomes. As an important virulence factor, staphyloxanthin can impair neutrophil killing and protect bacteria against the host innate immune system [Citation43]. Our study found that glmS knockout resulted in decreased pigment expression in the NCTC 8325 strain. Along with the inhibition of the production of staphyloxanthin in NCTC 8325 ∆glmS, the pathogenicity of the other strains was also significantly decreased, including haemolysin phenotype changes, changes in haemolysin gene expression and reductions in biofilms. The above virulence phenotype attenuation in NCTC 8325 ∆glmS was accompanied by downregulation of the sigB gene compared with that in the wild-type strain, which indicated that GlmS participates in the global regulation of S. aureus by regulating the activities of sigB, including virulence factor expression, pigments, biofilms, etc.
GlmS responds to the intracellular concentration of a variety of metabolites and second messengers. The natural ligand of glmS is GlcN6P [Citation36], a key precursor for the synthesis of amino sugars in bacterial and eukaryotic cell walls, which participates in the glycosyl transfer reaction in the synthesis of glycoproteins by adding glycosyl to the proteins and thus forming glycoproteins. Recent research shows that carba-sugars and carba-sugar analogs can activate the glmS riboswitch [Citation13,Citation35]. AGEs refer to a group of stable terminal products produced by the free amino groups of proteins, amino acids, lipids or nucleic acids, and the aldehyde groups of reducing sugars. As the end products of GlcN6P, AGEs-upregulated glmS seems to be a new “negative feedback” regulation of ribose switching within bacterial cells, which requires further research and confirmation.
In conclusion, this study clarified the mechanism and regulatory pathway by which AGEs promote the biofilm formation of S. aureus, and elucidates a new regulatory factor of sigB and reveals the important role of the GlmS protein in the global regulatory network of S. aureus.
Author contributions
LJN and XYX were responsible for designing experiments and writing the manuscript. RS, HL, LSH, YWD, and XYL participated in analysis of experimental results and drawing tables and figures. LJN, RS, XXL, XFZ, and YLW were responsible for the experiments. ZHD participated in literature searching and manuscript modification. All authors have read and approved the final manuscript. Informed consent was obtained from all individual participants included in the study.
Supplemental Material
Download MS Word (3.1 MB)Acknowledgements
The authors would like to thank the technical staff of the Nanhai Translational Innovation Center of Precision Immunology of Sun Yat-sen Memorial for their excellent technical assistance.
Disclosure statement
No potential conflict of interest was reported by the author(s).
Data availability statement
The data generated during the study are available at the repository figshare at https://doi.org/10.6084/m9.figshare.25627158.v1. The raw data of mass spectrometry proteomics data have been deposited to the ProteomeXchange repository at http://www.ebi.ac.uk/pride, with the dataset identifier PXD046076.
Supplemental data
Supplemental data for this article can be accessed online at https://doi.org/10.1080/21505594.2024.2352476
Additional information
Funding
References
- Archer NK, Mazaitis MJ, Costerton JW, et al. Staphylococcus aureus biofilms: properties, regulation, and roles in human disease. Virulence. 2011;2(5):445–12. doi: 10.4161/viru.2.5.17724
- Arciola CR, Campoccia D, Speziale P, et al. Biofilm formation in Staphylococcus implant infections. A review of molecular mechanisms and implications for biofilm-resistant materials. Biomaterials. 2012;33(26):5967–5982. doi: 10.1016/j.biomaterials.2012.05.031
- Xie X, Bao Y, Ni L, et al. Bacterial profile and antibiotic resistance in patients with diabetic foot ulcer in Guangzhou, Southern China: focus on the differences among different Wagner’s grades, IDSA/IWGDF grades, and ulcer types. Int J Endocrinol. 2017;2017:8694903. doi: 10.1155/2017/8694903
- McCarthy H, Rudkin JK, Black NS, et al. Methicillin resistance and the biofilm phenotype in Staphylococcus aureus. Front Cell Infect Microbiol. 2015;5:1. doi: 10.3389/fcimb.2015.00001
- Vlassara H, Uribarri J. Advanced glycation end products (AGE) and diabetes: cause, effect, or both? Curr Diab Rep. 2014;14(1):453. doi: 10.1007/s11892-013-0453-1
- Stensen MH, Tanbo T, Storeng R, et al. Advanced glycation end products and their receptor contribute to ovarian ageing. Hum Reprod. 2014;29(1):125–134. doi: 10.1093/humrep/det419
- Xie X, Yang C, Duan C, et al. Advanced glycation end products reduce macrophage-mediated killing of Staphylococcus aureus by ARL8 upregulation and inhibition of autolysosome formation. Eur J Immunol. 2020;50(8):1174–1186. doi: 10.1002/eji.201948477
- Xie X, Liu X, Li Y, et al. Advanced glycation end products enhance biofilm formation by promoting extracellular DNA release through sigB upregulation in Staphylococcus aureus. Front Microbiol. 2020;11:1479. doi: 10.3389/fmicb.2020.01479
- Baev N, Endre G, Petrovics G, et al. Six nodulation genes of nod box locus 4 in rhizobium meliloti are involved in nodulation signal production: nodM codes for D-glucosamine synthetase. Mol Gen Genet. 1991;228(1–2):113–124. doi: 10.1007/BF00282455
- Ferré-D’Amaré AR. The glmS ribozyme: use of a small molecule coenzyme by a gene-regulatory RNA. Quart Rev Biophys [internet]. 2010 [cited 2022 Dec 27];43(4):423–447. Available from: https://www.cambridge.org/core/product/identifier/S0033583510000144/type/journal_article
- Deigan KE, Ferré-D’Amaré F-D. Riboswitches: discovery of drugs that target bacterial gene-regulatory RNAs. Acc Chem Res. 2011;44(12):1329–1338. doi: 10.1021/ar200039b
- Komatsuzawa H, Fujiwara T, Nishi H, et al. The gate controlling cell wall synthesis in Staphylococcus aureus. Mol Microbiol. 2004;53(4):1221–1231. doi: 10.1111/j.1365-2958.2004.04200.x
- Stängle D, Silkenath B, Gehle P, et al. Carba-sugar analogs of glucosamine-6-phosphate: new activators for the glmS riboswitch. Chemistry A European J. 2023;29(3):e202202378. doi: 10.1002/chem.202202378
- Li Z, Zhang Y, Sui S, et al. Targeting HMGB3/hTERT axis for radioresistance in cervical cancer. J Exp Clin Cancer Res [internet]. 2020 [cited 2023 Apr 21];39(1):1–17.
- Kido K, Yamanaka S, Nakano S, et al. AirID, a novel proximity biotinylation enzyme, for analysis of protein-protein interactions. Elife. 2020;9:e54983. doi: 10.7554/eLife.54983
- Perez-Riverol Y, Bai J, Bandla C, et al. The PRIDE database resources in 2022: a hub for mass spectrometry-based proteomics evidences. Nucleic Acids Res. 2022;50(D1):D543–D552. doi: 10.1093/nar/gkab1038
- Tanhaeian A, Azghandi M, Mousavi Z, et al. Expression of thanatin in HEK293 Cells and investigation of its antibacterial effects on some human pathogens. Protein Pept Lett. 2020;27(1):41–47. doi: 10.2174/0929866526666190822162140
- Zeng L, Wang Y-L, Wang F, et al. Construction of the POT1 promoter report gene vector, and the effect and underlying mechanism of the POT1 promoter in regulating telomerase and telomere length. Oncol Lett. 2017;14:7232–7240. doi: 10.3892/ol.2017.7127
- Young TL, Whisenhunt KN, LaMartina SM, et al. Sonic hedgehog intron variant associated with an unusual pediatric cortical cataract. Invest Ophthalmol Vis Sci [internet]. 2022 [cited 2023 May 11];63(6):25. Available from: https://www.ncbi.nlm.nih.gov/pmc/articles/PMC9234370/
- Zhang J, Zhao L, Li Y, et al. Circadian clock regulates granulosa cell autophagy through NR1D1-mediated inhibition of ATG5. American Journal of Physiology-Cell Physiology [internet]. 2022 [cited 2023 Jun 1];322(2):C231–C245.
- Baba T, Bae T, Schneewind O, et al. Genome sequence of Staphylococcus aureus strain Newman and comparative analysis of staphylococcal genomes: polymorphism and evolution of two major pathogenicity islands. J Bacteriol. 2008;190(1):300–310. doi: 10.1128/JB.01000-07
- Bae T, Schneewind O. Allelic replacement in Staphylococcus aureus with inducible counter-selection. Plasmid [Internet]. 2006 [cited 2023 Oct 21];55(1):58–63. doi:10.1016/j.plasmid.2005.05.005
- Zhang L, Zou W, Ni M, et al. Development and application of two inducible expression systems for Streptococcus suis. Microbiol Spectr [Internet]. 2022 [cited 2023 Sep 27];10(4):e00363–22.
- Imran M, Liu T, Wang Z, et al. Evolutionary conservation of nested MIR159 structural microRNA genes and their promoter characterization in Arabidopsis thaliana. Frontiers in plant science [Internet]. 2022 [cited 2023 May 15];13. Available from 10.3389/fpls.2022.948751
- Jiang L, Yi T, Shen Z, et al. Aloe-emodin attenuates Staphylococcus aureus pathogenicity by interfering with the oligomerization of α-toxin. Front Cell Infect Microbiol. 2019;9:157. doi: 10.3389/fcimb.2019.00157
- Liang H, He K, Li T, et al. Mechanism and antibacterial activity of vine tea extract and dihydromyricetin against Staphylococcus aureus. Sci Rep. 2020;10(1):21416. doi: 10.1038/s41598-020-78379-y
- Zheng X, Chen L, Zeng W, et al. Antibacterial and anti-biofilm efficacy of Chinese Dragon’s blood against Staphylococcus aureus isolated from infected wounds Isolated From Infected Wounds. Frontiers in Microbiology [Internet] Front Microbiol. 2021 [cited 2023 Aug 2];12.
- Grossman AB, Burgin DJ, Rice KC. Quantification of Staphylococcus aureus biofilm formation by crystal violet and confocal microscopy [Internet]. In: Rice K, editor. Staphylococcus aureus: methods and protocols. New York (NY): Springer US; 2021 [cited 2023 Aug 3]. p. 69–78.
- Liu H, Shang W, Hu Z, et al. A novel SigB(Q225P) mutation in Staphylococcus aureus retains virulence but promotes biofilm formation. Emerg Microbes Infect [Internet] 2018 [cited 2023 Jun 2];7:1–12.
- Qi M, Liu Q, Liu Y, et al. Staphylococcus aureus biofilm inhibition by high voltage prick electrostatic field (HVPEF) and the mechanism investigation. International Journal of Food Microbiology [internet]. 2022 [cited 2023 Aug 4];362:109499. Available from: https://www.sciencedirect.com/science/article/pii/S016816052100458X
- Wu S, Qin B, Deng S, et al. CodY is modulated by YycF and affects biofilm formation in Staphylococcus aureus. Front Microbiol [Internet]. 2022 [cited 2023 Jun 16];13:967567. 10.3389/fmicb.2022.967567
- Cui J, Zhang H, Mo Z, et al. Cell wall thickness and the molecular mechanism of heterogeneous vancomycin-intermediate Staphylococcus aureus. Lett Appl Microbiol. 2021;72(5):604–609. doi: 10.1111/lam.13456
- Wang Y, Lau PC. Sequence and expression of an isocitrate dehydrogenase-encoding gene from a polycyclic aromatic hydrocarbon oxidizer, sphingomonas yanoikuyae B1. Gene. 1996;168:15–21. doi: 10.1016/0378-1119(95)00732-6
- Traykovska M, Popova KB, Penchovsky R. Targeting glmS ribozyme with chimeric antisense oligonucleotides for antibacterial drug development. ACS synth biol [Internet]. 2021 [cited 2023 Feb 15];10(11):3167–3176.
- Lünse CE, Schmidt MS, Wittmann V, et al. Carba-sugars activate the glmS-riboswitch of Staphylococcus aureus. ACS Chem Biol. 2011;6(7):675–678. doi: 10.1021/cb200016d
- Klein DJ, AR F-D. Structural basis of glmS ribozyme activation by glucosamine-6-phosphate. Science. 2006;313(5794):1752–1756. doi: 10.1126/science.1129666
- Chen Q, Wang H, Li Z, et al. Circular RNA ACTN4 promotes intrahepatic cholangiocarcinoma progression by recruiting YBX1 to initiate FZD7 transcription. J Hepatol [internet]. 2022 [cited 2023 May 30];76(1):135–147. 10.1016/j.jhep.2021.08.027
- Twarda-Clapa A, Olczak A, Białkowska AM, et al. Advanced glycation end-products (AGEs): formation, chemistry, classification, receptors, and diseases related to AGEs. Cells. 2022;11(8):1312. doi: 10.3390/cells11081312
- Takeuchi M, Sakasai-Sakai A, Takata T, et al. Effects of Toxic AGEs (TAGE) on human health. Cells. 2022;11(14):2178. doi: 10.3390/cells11142178
- Sellegounder D, Zafari P, Rajabinejad M, et al. Advanced glycation end products (AGEs) and its receptor, RAGE, modulate age-dependent COVID-19 morbidity and mortality. A review and hypothesis. Int Immunopharmacol. 2021;98:107806. doi: 10.1016/j.intimp.2021.107806
- Tran HT, Bonilla CY. SigB-regulated antioxidant functions in gram‐positive bacteria. World J Microbiol Biotechnol [Internet]. 2021 [cited 2023 Aug 15];37(3):38. Available from 10.1007/s11274-021-03004-7
- McCown PJ, Roth A, Breaker RR. An expanded collection and refined consensus model of glmS ribozymes. RNA. 2011;17(4):728–736. doi: 10.1261/rna.2590811
- Liu C-I, Liu GY, Song Y, et al. A cholesterol biosynthesis inhibitor blocks Staphylococcus aureus virulence. Science. 2008;319(5868):1391–1394. doi: 10.1126/science.1153018