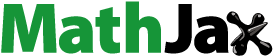
ABSTRACT
Taken separately, a single sweet sorghum stem bioconversion process for bioethanol and biomethane production only leads to a partial conversion of organic matter. The direct fermentation of crushed whole stem coupled with the methanization of the subsequent solid residues in a two-stage process was experimented to improve energy bioconversion yield, efficiency, and profitability. The raw stalk calorific value was 17,144.17 kJ/kg DM. Fermentation step performed using Saccharomyces cerevisiae resulted in a bioconversion yield of 261.18 g Eth/kg DM, i.e. an energy recovery efficiency of 6921.27 kJ/kg DM. The methanogenic potentials were 279 and 256 LCH4/kg DM, respectively, for raw stem and fermentation residues, i.e. energy yields of 10,013.31 and 9187.84 kJ/kg DM, respectively. Coupling processes have significantly increased yield and made it possible to reach 13,309.57 kJ/kg DM, i.e. 77.63% of raw stem energy recovery yield, compared to 40.37% and 58.40%, respectively, for single fermentation and methanization processes.
HIGHLIGHTS
Sweet sorghum stem is a viable feedstock source for efficient coproduction of ethanol and methane
Sorghum stems calorific value determination revealed an energy potential of 17.15 MJ/kg DM
Energy recovery by single methanization yielded 18.03% more than ethanol fermentation
Coupling processes has significantly increased energy recovery yield and profitability
GRAPHICAL ABSTRACT
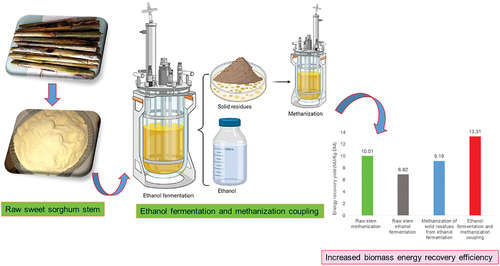
1. Introduction
World population increase and economic development lead to an exponential growth of the global energy demand. The energy sector, which is essentially based on fossil resources, is the source of about three quarters of GHG emissions and has already raised the mean global temperature by 1.1°C with a lot of negative impacts on the environment [Citation1]. While fossil resources are increasingly depleted, further accentuating concerns about energy and environmental security. Faced with this context, the international community has opted for an energy transition policy targeted to reduce GHG emissions and achieve carbon neutrality in order to cap global warming to 1.5°C by 2050 [Citation2]. The use of renewable energy sources has become an absolute and unavoidable necessity in order to meet this current challenge. Development of energy recovery efficiency from biomass is one of the most important ways of keeping our environment sustainable. Lignocellulosic biomass is one of the most available alternative sources of energy and accounts for approximately 14% of renewable energy consumption worldwide [Citation3]. In the last decade, the use of lignocellulosic biomass agricultural residues as alternative to generate various kinds of energy vector biomolecules, has gained increasing interest because of its several interesting features including diversity, sustainability, and low GHG emissions [Citation4]. Sweet sorghum is one of the most attractive sources of bioenergy because of its versatility, combining the production of starchy grains, high fermentable sugars content stalks and a high green biomass yield [Citation5]. Sorghum multifunctionality gives it the specificity of providing interesting solutions to meet energy and environmental challenges without adversely affecting food chain [Citation6]. Its green biomass productivity varies from 20 to 120 tonnes/ha, depending on the agronomic and environmental conditions as well as the variety’s botanical specificities [Citation7]. In addition, several features make sorghum one of the most interesting cereal crops, including resistance to extreme agronomic conditions, resilience, low input requirements and high production yield. Fifth most produced cereal crops worldwide, sorghum global production in 2020 was estimated at 58.70 million tonnes out of a cropping area of 40.25 million hectares [Citation8]. Sweet sorghum stalks energy recovery like any other lignocellulosic biomass, can be carried out using several techniques, in particular thermochemical conversion (direct combustion, pyrolysis, gasification, liquefaction, etc.) or biological conversion (ethanol fermentation, biomethanization, dark fermentation for hydrogen production, etc.). Thermochemical conversion has low overall energy recovery yields and emits large amounts of pollutants, and therefore many operational and environmental challenges are associated with it [Citation9]. On the other hand, bioconversion presents economic and environmental advantages compared to other technologies [Citation10]. Bioethanol and biomethane are among the most well-known energy vector biomolecules produced from the bioconversion of sweet sorghum stem [Citation11]. Currently, methanization proves to be the more efficient method of lignocellulosic biomass energy bioconversion compared to ethanol fermentation [Citation12]. However, taken separately, a single bioconversion process only leads to a partial conversion of organic matter. More specifically, energy recovery yield from sweet sorghum stems by single ethanol fermentation process is between 6500 and 8900 kJ/kg DM (considering ethanol energy yield at 26,500 kJ/kg) [Citation10,Citation11], while the energy potential or calorific value of sweet sorghum stalk has been estimated at around 18,320 kJ/kg DM [Citation13,Citation14]. This low yield is mainly due to the fact that this lignocellulosic biomass is composed mostly of cellulose, hemicellulose and lignin which integrate strongly forming a complex structure, resistant and difficult to metabolize by microorganisms. A pretreatment step aimed at fractionation is often necessary in order to enhance the accessibility of microorganisms to carbohydrate polymers. Several pre-treatment techniques (mechanical, chemical, thermal, biological, or their eventual combination, etc.) are currently used and have improved performance [Citation15]. However, they have limitations such as high energy or reagent costs, potential pollutants, formation of inhibitor compounds for microbial metabolism, low efficiencies, substrate loss, etc. [Citation4]. In addition, the pretreatment of lignocellulosic biomass, in particular, the production of sugar monomers from the fibrous fraction, is a complex and the costliest part of the biomass energy conversion process [Citation16]. Moreover, the typical process of ethanol production from sweet sorghum stalks proves to be unprofitable because of the very costly juice extraction step. The liquid fraction and the solid residues are usually processed separately. This fractionation step leads to a low energy bioconversion yield, while efficient pathways of biomass energy recovery with lower energy consumption are essential for cost-effective production of bioenergy from lignocellulosic feedstocks [Citation17–19]. Development of more efficient and profitable energy bioconversion process is therefore necessary. This study experiments a combination of direct ethanol fermentation and methanization in order to enhance the overall energy recovery efficiency and profitability of this process. Direct ethanol fermentation (without juice extraction nor pre-treatment step) of crushed whole stem using Saccharomyces cerevisiae coupled with the mesophilic methanization of the subsequent solid residues in a two-stage process has been proposed in this work. The overall objective is to improve the energy bioconversion process yield and performance of sweet sorghum stems.
2. Material and methods
2.1. Sorghum stems collecting and pretreatment
The studied sweet sorghum stalks (Sorghum bicolor (L) Moench) were harvested in the locality of ’KODEK’ (10° 39′ 19”N; 14° 24’ 42”E; 880 m), department of DIAMARE, Far North region of Cameroon. Stalk samples were then forthwith stripped, cut out, and kept at −20°C. Ethanol fermentation and methanization have been studied (separately and in combination). shows the overall process diagram. The sorghum variety was first formally identified (species, cultivar, GPS coordinates, etc.), and then a sufficient quantity of stalks was harvested. The stems then underwent a physicochemical characterization. Ethanol production has been experimented on different fractions of the biomass (juice, pith, and whole stem) in order to compare the process efficiency. For this purpose, the stripped stems were cut up, dried (steaming at 60°C until obtaining constant mass) and crushed to a grain size ≤0.5 mm using a mill. A sample of stems was peeled to separate the pith from the bark, and then dried and crushed in the same way. The juice was extracted from wet pith samples by grinding and mechanical squeezing using a mill then characterized before being fermented following the description of route 1 (). According to itinerary 2, direct fermentation of crushed pith suspended in water has been experimented. During route 3, single direct ethanol fermentation and direct methanization were experimented on raw shredded whole stalk. Then, the methanization of the subsequent solid residues from ethanol fermentation was also tested in order to compare the efficiencies of the processes separately and the fermentation/methanization coupling process.
2.2. Sweet sorghum stalk physicochemical characterization
The determination of the biomass dry matter content has been carried out according to the Association of Official Analytical Chemists (AOAC) method [Citation20]. Samples were first steamed at 105°C while monitoring the decrease in mass until a stable mass was obtained. The biomass dry matter rate, expressed as a percentage of wet matter, has been estimated after cooling samples in the atmosphere of a desiccator, according to EquationEquation (1)(1)
(1) :
With, DMr: Dry matter rate (%); Mf: Final crucible mass and its residual dry contents (g); Mi: Initial crucible mass and its wet contents (g); T: Tare of empty crucible (g).
The moisture rate of the samples (Mr) has been deduced from the dry matter content according to EquationEquation (2)(2)
(2) :
The ash content determination has been carried out by incineration in an electric muffle furnace (550°C for 6 h) of the subsequent dry residues of the samples. The mineral content has been expressed as a percentage of DM and estimated after cooling in the atmosphere of a desiccator, according to EquationEquation (3)(3)
(3) :
With, MMr: Mineral matter rate (%); Mf: Final crucible mass and its residual sample contents after incineration (g); Mi: Initial crucible mass and its dry sample contents before incineration (g); T: Tare of empty crucible (g).
The organic matter content (OMC) has been deduced from the mineral matter content according to EquationEquation (4)(4)
(4) :
All experiments have been performed in triplicate, and the results are the mean values of the repetitions.
The determination of the sorghum stalk biochemical composition has been carried out according to Van Soest [Citation21].
Sorghum stalk total fiber rate, expressed as a percentage of DM, has been evaluated as described by EquationEquation (5)(5)
(5) :
With, S: Initial mass of biomass sample (g) and W1: Residual mass of insoluble in neutral detergent (g).
Hemicellulose has been evaluated from the dried residual parts of neutral detergent-insoluble fibers (W1). It was expressed as a percentage of DM and quantified as described by EquationEquation (6)(6)
(6) :
where W2 represents the residual mass of a biomass fraction insoluble in acid detergent (g).
Cellulose and lignin have been quantified after sulfuric acid (72%) digestion of residual fractions of acid-detergent-insoluble fibers (W2). They were expressed as a percentage of DM and estimated as described by EquationEquations. (7)(7)
(7) and (Equation8
(8)
(8) ), respectively.
where W3 represents the residual mass of a biomass sample after digestion with sulfuric acid (g).
The phenolic compounds content was determined by the assay method using Folin Ciocalteu’s reagent according to the protocol described by Gutfinger [Citation22] and expressed in grams of gallic acid equivalent/liter (g GAE/L).
Calorific values were determined according to the bomb calorimeter method using an isoperibol calorimeter (calorimètre PARR 6400). To this end, the shredded biomass fractions were previously compressed (3 kPa for 1 min) in the form of pellets using a mechanical press. Then, a complete combustion (in the presence of pressurized oxygen) of a pellet sample was carried out in the bomb calorimeter. The bomb was immersed in the water of the calorimeter, and the heat released by the combustion was transmitted to the water whose temperature rise was measured. The higher calorific value, calculated as gross heat of combustion, was calculated according to EquationEquation (9)(9)
(9) :
where HCV is the higher calorific value of the sample (cal/g); M represents the total water mass of the calorimeter (g); Ce is the water specific heat capacity (1 cal/g.°C); ΔT (Tmax − Tmin) is the water temperature increase (°C); e1, e2, and e3 represent, respectively, the heat released by the combustion of nitrogen, sulfur, and fuse wire (cal); m represents the mass of the biomass sample (g).
The total mass of water in the calorimeter (M) has been previously determined by the combustion of a mass (m) of benzoic acid of known higher calorific value (HCV = 6319 cal/g), and the value of M was calculated according to EquationEquation (10)(10)
(10) :
2.3. Determination of sugar content and carbohydrate profile of biomass
The contents of water-soluble sugars and non-water-soluble structural sugars were determined by the enzymatic assay method, using K-SUFRG assay kit (Megazyme, Bray Business Park Bray, Co. Wicklow A98 YV29, Ireland) according to the protocol elaborated by Outlaw and Mitchell [Citation23], Beutler [Citation24], and Kunst et al. [Citation25]. For this purpose, the carbohydrate extracts were previously filtered using Whatman N°1 filter paper and then D-glucose concentration of samples was firstly estimated before and after hydrolysis of sucrose by β-fructosidase (invertase). The samples D-fructose contents were estimated after the D-glucose assay and after isomerization by phosphoglucose isomerase (PGI). Water-soluble sugars were extracted from different shredded biomasses with hot water. To that end, 1 g of ground biomass fraction has been mixed with 50 mL of Milli-Q water in a flask and heated to 90°C for 90 min using a water bath. The solution was filtered under vacuum using a size 3 frit (40–90 μm), and the filtrate was recovered and used for the determination of total water-soluble sugars as well as for the determination of the carbohydrate profile. The subsequent fibrous fractions from the filtration were dried (60°C until mass stabilization) then hydrolyzed with sulfuric acid (0.75 M) in order to extract the structural water-insoluble sugars. To do this, 0.2 g of the dried fibrous fractions have been mixed with 5 mL of sulfuric acid solution (0.75 M) and heated (100°C for 90 min) using a water bath. The hydrolyzates were then subjected to 10 min of centrifugation at 13 000 g. The supernatants were recovered and neutralized (pH = 7.0 ± 0.1) using a 10 M NaOH solution and used for total water-insoluble sugars assay and for the determination of their monosaccharidic composition.
The monosaccharidic composition of the different extracts was investigated by the High-Performance Anion-Exchange chromatography (HPAEC-PAD) device, which is an ICS 3000 ion chromatography chain (Dionex Corporation, Sunnyvale (CA), USA). It consists of a pump module that can operate in gradient and a pulsed amperometric detector (HPAE-PAD). The stationary phase consists of polystyrene and divinylbenzene beads 10 μm in diameter on which particles functionalized by NR4+ groups are agglomerated. It reaches stable pressures of 275 bars in a pH range of 0 to 14. The samples were eluted in isocratic mode with a 16 mM decarbonated NaOH solution at 0.5 mL/min of flow rate for 20 min. After each elution, a 30 min chase with a 100 mM NaOH solution was performed to elute any contaminants still interacting with the stationary phase. Before each analysis, the column was equilibrated with the 16 mM NaOH solution for 10 min. All samples have been filtered using 0.22 µm filter before being injected. The injections are performed in triplicate, and the injection loop is 25 µL. Data acquisition and processing have been carried out using chromeleon software (version 6.8).
2.4. Ethanol production from different sorghum stalk fractions
Saccharomyces cerevisiae ATCC 7754 was used for ethanol production. The strain was taken up in sterile water and spread on YM-agar agar (yeast Medium, Difco 0712-01-8) and then incubated at 28°C for 24 h. It was then preserved at 4°C and subcultured on a petri dish for 24 h before being used as a preculture to inoculate the reactor [Citation26]. The fermentation was carried out using an aerated and stirred reactor of 6 L total volume (Biostat A, B. BRAUN, Germany), with 4 L of working volume. Culture media consisted of 10% (m/v) of equivalent biomass fraction (particle size of 0.5 mm) or juice diluted at 60 g/L of total sugars content, supplemented with additional nutrients as indicated by Kristiansen [Citation27] with the exception of glucose. A glucose control was carried out in parallel. Culture media have been firstly autoclaved (121°C for 20 min) before being inoculated with 10% (v/v) of a preculture of Saccharomyces cerevisiae in the exponential growth phase. The temperature of the culture medium was set at 30°C, with an aeration of 1.5 L/min, 500 rpm of stirring speed, and the pH was fixed at 5 by the automatic addition of a 2 M NaOH solution. Samples have been taken every 2 h (shredded stalk and marrow) and every 1 h (sorghum juice and glucose control) for fermentation kinetics monitoring (total sugars consumption, microbial growth, and the production of bioethanol). The total sugars consumption (for juice, crushed whole stem and marrow) was monitored by the assay method described by Dubois et al. [Citation28] using sulfuric acid and phenol. Biomass growth was monitored measuring A600 (Biomate 3S, UV–visible spectrophotometer, Thermo Scientific, Lyon, France). Bioethanol production and glucose consumption (for glucose control) were monitored using HPLC (1260 Infinity Quaternary LC system, Agilent Technology, Palo Alto, CA, USA) device. It was provided with two ionic exclusion columns (Rezex ROA 300 × 7.8 mm, Phenomenex, Torrance, CA, USA) interconnected in series in an oven (50°C) and coupled to a refractometer (HP 1100 series, Agilent Technologies, USA) as products detector. The mobile phase involved a solution of 2 mM sulfuric acid at 0.7 mL/min flow rate and 70 bars (7000 kPa). In order to avoid column clogging, all the samples have been firstly deproteinized before injection. To this end, 125 μL of a 0.3 M barium hydroxide solution and 125 μL of zinc sulfate solution (5% w/v) have been mixed to 1 mL of samples and subjected to 10 min of centrifugation (Thermo scientific, Lyon, France) at 10 000 g. The supernatants have been filtered using a 0.2 μm cellulose acetate filter (Chromafil, Steinheim, Germany) before being analyzed.
2.5. Determination of biochemical methane potential
The methanogenic potential was determined on the shredded raw stem without ethanol fermentation as well as on the subsequent solid residues from fermentation. Methanization was carried out in a stirred reactor of 2 L total volume with 1.4 L of working volume. The substrate was inoculated by a consortium from the digestate of the Methelec methanization unit. The tests were carried out under mesophilic conditions (37 ± 1°C), with a stirring speed of 120 rpm. The methanogenic potential and the quality of the gas (volume, biogas composition) have been followed according to the French Agency for Ecological Transition (ADEME) method [Citation29]. Data statistical analysis was performed using the Excel spreadsheet of the Microsoft office software version 2013. The experiments have been performed in three repetitions, and results were reported as an average ± standard deviation.
3. Results and Discussion
3.1. Sugar content and monosaccharidic composition of biomass
The whole sorghum stem water-soluble sugars composition was sucrose, glucose, and fructose with concentrations estimated, respectively, at 79.29, 199.22, and 196.08 g/kg DM (). The sugar concentrations of sorghum variety subject to this study are much higher than those of the varieties experimented by Crépeau et al. [Citation30] whose concentrations were 2.4–34.9, 25.2–39.1, and 20.4–32.1 g/kg DM, respectively. However, it is much closer to those of the varieties experimented by Ostovareh et al. [Citation31], with, respectively, 121, 92, and 83 g/kg DM. The same carbohydrate profile was observed in the pith and in the bark. However, the sucrose contents were much higher in the pith and in the bark compared to that of the whole stem. They were 212.83 and 82.05 g/kg DM, respectively, for marrow and bark. This study shows that, sorghum stalk water-soluble sugar content is essentially concentrated in the pith compared to the bark, where the contents were only 82.05, 52.28, and 47.52 g/kg DM, respectively, for sucrose, glucose, and fructose. These results are in accordance with the remarks made by Billa et al. [Citation32], who studied the biochemical composition of the sweet sorghum stem and indicated that the pith was twice as rich in glucose and sucrose (71% of dry weight) compared to bark (34.6%). These results show that this agricultural by-product, due to its high concentration of fermentable water-soluble sugars, constitutes an interesting source of raw material for bioethanol production. This sorghum stem’s high content of free and fermentable sugars makes it suitable for the production of several types of energy vector biomolecules. It is therefore relevant to explore and find the most efficient and profitable energy conversion pathways. Direct ethanol fermentation/methanization coupling process would optimize energy recovery while avoiding the costly steps of fractionation and pretreatment of the biomass. Ethanol fermentation produces not only ethanol but also a large quantity of microbial biomass (Saccharomyces cerevisiae) which is often very little valued. Optimization of energy bioconversion process of fermentable sugars from sorghum stalks would involve the energy recovery of solid fermentation co-products, in particular, the Saccharomyces cerevisiae biomass. The valorization of liquid secondary metabolites from ethanol fermentation, particularly short-chain carboxylic acids (succinate, acetate, propionate, lactate, etc.) is also positioned as a new innovative and promising bioconversion pathway. Medium chain carboxylic acids production from waste biomass through chain elongation reaction has currently attracted the attention of several authors and is considered as a promising way for the future compared to traditional techniques of single ethanol fermentation or methanization pathways [Citation33].
Table 1. Carbohydrate profile and sugar content of different biomass fractions.
Furthermore, analysis of the biomass fibrous fraction (bagasse) hydrolyzate reveals that the water-insoluble structural sugars were composed mainly of xylose, glucose, arabinose, and some traces of galactose. For the crushed whole stem bagasse, the sugar proportions were 53.07%, 38.55%, 6.44%, and 1.92%, respectively, for xylose, glucose, arabinose, and galactose (). The same carbohydrate profile was observed for the marrow and bark fractions, with a predominance of xylose and glucose contents, evaluated, respectively, at 25.65% and 67.88% for the marrow bagasse and 67.81% and 24.79% for bark bagasse. Sipos et al. [Citation34] also investigated the carbohydrate profile of sweet sorghum stalk bagasse. The sugar concentrations were 44.14, 17.96, and 5.53 g/L, respectively, for xylose, glucose, and arabinose, i.e. 65.26%, 26.55%, and 8.17%, respectively. These results show that sweet sorghum stalk bagasse is a source of additional sugars that could be used to optimize sorghum stem energy bioconversion through a prior saccharification process. However, lignocellulosic biomass saccharification techniques (enzymatic hydrolysis, biocatalyst in particular), although they improve the energy bioconversion yield, prove to be economically unprofitable due to the cost of reagents and operating conditions. Chemical hydrolysis, due to its toxic and corrosive nature, is not environmentally friendly and generates microbial growth inhibitor compounds. It is therefore necessary to reconsider the energy bioconversion strategies and pathways of this biomass. Moreover, due to the presence of a high proportion of xylose in bagasse hydrolyzate, its valorization into bioethanol requires the choice of microorganisms capable of metabolizing pentoses in order to make the bioconversion process more profitable.
3.2. Biochemical composition of sorghum stalk juice
The biochemical composition of the biomass is a crucial factor that determines the relevance of the choice of adequate energy recovery technique. The juice sugar content of the S. bicolor studied was 252.51 and 78.46 g/L, respectively, for total water-soluble sugars and reducing sugars. This concentration of total water-soluble sugar is much higher than that indicated by Djomdi et al. [Citation35] on the same variety (98.85 g/L). This sugar content is also higher than those of the varieties experimented by Crépeau et al. [Citation30] and Daniel et al. [Citation36], who reported sugar contents evaluated, respectively, at 60.89–111.10 g/L and 100.3 g/L. Moreover, the sorghum juice carbohydrate profile for this study was mainly composed of glucose, fructose, and sucrose. Sucrose was the dominant sugar, and the proportions were 64.4%, 21.13%, and 14.47%, respectively, for sucrose, glucose, and fructose. Similar compositions, evaluated on other sweet sorghum varieties, were previously indicated by Prasad et al. [Citation37] (60%, 33%, and 7%, respectively, in sucrose, glucose, and fructose) and Serna-Saldívar et al. [Citation38] with proportions estimated at 53–85%, 9–33%, and 6–21%, respectively. This high sucrose content is consistent with other experiments [Citation39,Citation40], and the glucose concentration is very often higher than that of fructose. This carbohydrate profile of sorghum juice is comparable to that of sugar cane juice. However, sugar cane juice has a relatively higher sucrose content (90–98%) with very low proportions of glucose (0.5–4%) and fructose (0.5–6%) [Citation38,Citation41]. The sorghum juice phenolic compounds content was evaluated at 0.32 g/L. A similar observation was indicated by Sereme et al. [Citation42] who reported contents of phenolic compounds <2% DM in the sorghum caudatum stalk. This low content of phenolic compounds in sorghum juice is a major asset for its valorization for ethanol production, since these compounds have detrimental effects on biomass growth and metabolism and can act, at high concentrations, as antimicrobials [Citation43]. Beyond this sorghum stalk juice biochemical composition (high fermentable sugars content with low concentration of phenolic compounds), very suitable for ethanol production, the extraction processes tend to restrict its profitability due to high water and energy consumption. Enzymatic treatment applied to the shredded whole stems without extraction step has been recently demonstrated by Bakari et al. [Citation44]. Admittedly, the authors have demonstrated that the extraction step could be dispensed and therefore significantly improve the process economic profitability, but the energy bioconversion yield remains to be perfected. This explains the need to experiment with the coupling of direct ethanol fermentation of shredded whole stems and the methanization of subsequent solid residues. This would make it possible to valorize both the stem fibrous fraction and the microbial biomass (Saccharomyces cerevisiae) as well as other fermentation secondary metabolites. Avoiding the extraction and hydrolysis steps would make the process more profitable.
3.3. Biochemical composition and calorific value of different biomass fractions
Analysis of the chemical composition of raw deleafed sorghum stalk revealed contents estimated at 93.5% and 87.5%, respectively, for dry matter and organic matter. These contents are comparable to those of the varieties experimented by Luna et al. [Citation45], who reported 95.3–95.9% and 94.6–95.6%, respectively, for DM and OM. The solid residues from the ethanol fermentation process have compositions similar to that of the shredded raw stalk. The contents were 96.0% and 86.5%, respectively, for DM and OM. This could be explained by the fact that these solid fermentation residues, although devoid of water-soluble sugars, also contain large quantities of microorganisms (Saccharomyces cerevisiae), and therefore additional organic matter. The hemicellulose, cellulose, and lignin contents of the raw stem were 17.39%, 22.27%, and 5.09% DM, respectively, i.e. 88.61% of these sorghum stem total fibers were made up of hemicellulose (38.85%) and cellulose (49.76%). This represents a potential source of substrates for bio-methane production, since the expensive process of fibrous fraction saccharification is not necessary for energy recovery through methanation pathway. These contents are much higher than those of the variety experimented by Billa et al. [Citation32], who reported 10.2% and 12.4% DM, respectively, for hemicellulose and cellulose. However, they are much closer to those of the varieties experimented by Khalil et al. [Citation40], (11.7–17.2% and 20.1–26.1% DM, respectively, for hemicellulose and cellulose) and those of the variety studied by Billa et al. [Citation32] (4.8% for lignin). Similar experiments performed by several authors indicate that total fibers content as well as its distribution in hemicellulose, cellulose, and lignin vary widely depending on the studied varieties of sorghum. Moreover, the total fiber contents were 81.84% DM and 44.75% DM, respectively, for ethanol fermentation solid residues and raw stems. Ethanol fermentation solid residues cellulose, hemicellulose, and lignin contents were 33.11, 44.87, 3.86% DM, respectively (), and 95.28% of total fibers consisted of hemicellulose (54.83%) and cellulose (40.45%) compared to 88.61% for raw stem fibers. This confirms that the residual stem fibrous fraction has been enriched with fibers from Saccharomyces cerevisiae growth and is therefore a potential source of additional energy that can be recovered by a subsequent methanation process. In addition to the microbial fiber supply, fermentation process also allowed partial degradation of sorghum stem fibers (). However, Saccharomyces cerevisiae not being able to degrade fibers, this degradation observed after fermentation was essentially due to the autoclaving operation. The ethanol fermentation-methanization coupling process effectively improves the energy recovery yield without costly traditional pretreatment methods. The calorific values were 17,144.17 and 18,117.23 kJ/kg DM, respectively, for raw stalk and solid residues from ethanol fermentation process. These results show that ethanol fermentation residues have a slightly higher energy potential than that of the raw biomass. This relatively high energy content of the ethanol fermentation residues could be explained by their high fiber content (81.84% DM) compared to the raw biomass (44.75% DM) (). Sweet sorghum stem biochemical composition and its calorific value were comparable to those of other lignocellulosic feedstocks such as corn stover, sugarcane, rice straw, switchgrass, etc., estimated at 16.28–18.64 MJ/kg (for calorific value), 35–53% DM (for cellulose), 15–36% DM (for hemicellulose), and 14–32% DM (for lignin) [Citation13,Citation38,Citation46].
Figure 2. Structure of raw sorghum stalk fibers before fermentation (a) and mixture of sorghum stalk fibers and microbial biomass after fermentation (b).

Table 2. Calorific value and biochemical composition of sorghum stem biomass before and after ethanol fermentation.
3.4. Ethanol production from different stalk fractions
Ethanol production was tested on three fractions of sorghum stalk biomass (shredded whole stalk and marrow with particle size ≤0.5 mm and mechanical extracted juice) in order to compare the efficiency of fermentation processes. The fermentation kinetics are presented in . The degradation rates (residual mass after fermentation) were 30.47% and 43.12%, respectively, for whole stem and pith. Ethanol fermentation efficiencies were 0.46, 0.43, and 0.44 g Eth/g consumed total sugars, respectively, for whole stem, pith, and juice (). These results show that there is no major difference between the fermentation efficiencies of the different stem fractions. The biomass fractionation, in particular, the juice extraction step, is therefore not necessary, thus offering the possibility of fermenting the whole ground stem, improving the process efficiency. In addition, whole stalk fermentation brings additional Saccharomyces cerevisiae fibers to the fibrous sorghum fraction and constitutes a major asset for subsequent methanization step of the ethanol fermentation residues. These results confirm the observations made by Bakari et al. [Citation44] who reported that the very expensive and laborious juice extraction step, consuming energy and water, could be avoided, significantly enhancing the efficiency and sorghum stem fermentation process performance. Similar ethanol production yields, 0.41–0.44 g Eth/g consumed sugar [Citation34]; 0.39–0.48 g Eth/g consumed sugar [Citation47] and 0.42–0.48 g Eth/g consumed sugar [Citation48] have been reported in different studies. The juice fermentation kinetics was similar and comparable to that of the glucose control fermentation (), which implies that the sweet sorghum juice, due to its biochemical composition and in particular its carbohydrate profile, constitutes an adequate substrate for ethanol production using Saccharomyces cerevisiae.
Figure 3. Kinetics of different biomass fractions fermentation: (a) shredded whole stalk and marrow, (b) juice and glucose control.

Furthermore, the bioconversion yields were 261.18, 280.02, and 274.85 g Eth/kg DM, respectively, for whole stalk, marrow, and juice (). These yields are much higher than those obtained by Ban et al. [Citation49], who reported 147 g/kg DM. These bioconversion yields, expressed in terms of energy recovery efficiency, were 6921.27, 7420.53, and 7283.52 kJ/kg DM, respectively (considering ethanol calorific value at 26,500 kJ/kg [Citation10]). These results are in accordance with those reported by some authors in the literature [Citation10,Citation11], who indicated that energy recovery efficiency of sorghum stalk bioconversion to ethanol was between 6500 and 8900 kJ/kg DM. These results indicate that sorghum stalk bioconversion by single ethanol fermentation made it possible to recover, respectively, 40.37%, 43.28%, and 42.48% of the raw biomass initial energy content. This justifies the need and the choice to recover the ethanol fermentation solid residues in the form of methane in order to optimize the energy conversion yield of this sorghum by-product.
3.5. Production of bio-methane from different biomass fractions
The bio-methanization was tested on the shredded raw stem before and after ethanol fermentation. The methanogenic potentials were (319 ± 11) and (296 ± 23) LCH4/kg of organic matter, respectively, for raw deleafed stalk and ethanol fermentation residues (). This result is in line with those of several other previously reported studies. Głąb et al. [Citation12] reported that the methanogenic potential of sweet sorghum stalks was 296–342 LCH4/kg total solids. It was 317 LCH4/kg volatile solids according to the study performed by Herrmann et al. [Citation50] on hybrid variety of sweet sorghum (Sorghum bicolor x sudanense). Expressed in terms of raw biomass, the potentials were (279 ± 10) and (256 ± 20) LCH4/kg of raw biomass, respectively, for raw deleafed stalk and fermentation residues. These results are comparable to those reported by Ostovareh et al. [Citation31], who obtained 278.7 LCH4/kg volatile solids after organosolv pretreatment of sweet sorghum stems. This bioconversion by anaerobic digestion process leads to the energy recovery yields of 10,013.31 and 9187.84 kJ/kg of raw biomass, respectively, for raw deleafed stalk and fermentation residues (considering the lower calorific value of methane at 35.89 MJ/m3 [Citation51]). Antonopoulou et al. [Citation10] experimented with methane production from sweet sorghum stem bagasse and reported that the methanogenic potential was 78 LCH4/kg and an energy yield of 9845 kJ/kg DM. This study shows that sorghum stalk bioconversion by anaerobic digestion process made it possible to recover, respectively, 58.40% and 53.59% of raw stalks initial energy content. These results show that the ethanol fermentation residues have similar and comparable methanogenic potential to that of the raw stem. This could be explained by the high fiber content of these residues composed of 44.87% and 33.11%, respectively, in hemicellulose and cellulose, against 17.39% and 22.27%, respectively, for the raw stem fibers (). The ethanol fermentation allowed an additional microbial fiber supply, significantly improving the methanogenic potential of the solid residues. The partial degradation of stem fibers during the fermentation step also facilitated their decomposition by methanogenic bacteria enhancing energy bioconversion efficiencies. Furthermore, bioconversion by single methanization of raw shredded stem has a better energy recovery yield (10013.31 kJ/kg) compared to single ethanol fermentation (6921.27 kJ/kg). The direct fermentation of shredded stalks coupled with methanization of the subsequent solid residues made it possible to reach 13,309.57 kJ/kg DM, with an energy recovery yield of 77.63% of raw biomass initial energy content (), i.e. respectively 37.26% and 19.23% more compared to single fermentation and single methanization (). Recovering sweet sorghum stems without going through costly extraction or hydrolysis processes significantly improves the overall energy conversion efficiency, making the process more cost-effective.
Table 3. Sorghum stalks bioconversion yield.
illustrates the cumulative production kinetics and the evolution of the methanogenic potential of the biomass as a function of the hydraulic retention time. The cumulative production of methane began from the first days of anaerobic digestion without a latency phase. It was relatively low (25%) during the first 11 days for both substrates. The exponential production phase started from the 12th day and the plateau (100%) was reached after 22 days and 25 days of methanization, respectively, for shredded raw stem and ethanol fermentation residues (). These results show that the ethanol fermentation solid residues, because of their high fiber content, constitute a substrate for methanization in the same way and comparable to the raw stem. Thus, highlighting the relevance of recovering them into methane in order to optimize the process energy efficiency. Głąb et al. [Citation12] experimented with the biomethanization of sweet sorghum stalk and noticed that the cumulative methane production increased intensively during the initial phase until the 5th day and the plateau was reached after approximately 30 methanization days. These observations are similar to those reported by Herrmann et al. [Citation50] who studied methanization on certain cereal crops (sorghum, maize, rye, triticale) and noticed a rapid production of methane during the first days of methanization without inhibition or lag phase. This rapid and intense production of methane could be explained by the fact that the organic matter contained in the substrate is easily biodegradable.
Figure 6. Kinetics of cumulative methane production (a) and evolution of biomass methanogenic potential (b). Evolution of CH4 and CO2 contents as a function of hydraulic retention time for raw stem (c) and solid ethanol fermentation residues (d).

shows the evolution of biogas composition, in particular, the evolution of the CH4 and CO2 contents according to the hydraulic retention time during the bio-digestion process. Biogas composition analysis revealed a high CO2 content at the beginning of the methanization process until the 7th day (62% CO2 and 38% CH4), then this trend was gradually reversed and reached 68% CH4 and 32% CO2 after the 22nd day of methanization of the shredded raw stem (). The same trend was observed for the bio-digestion of ethanol fermentation residues. The composition of the biogas was 53% CO2 and 47% CH4 on the 7th day and 60% CH4 and 40% CO2 after the 25th day of bio-digestion (). These results are comparable to those obtained by Antonopoulou et al. [Citation10] who experimented with the methanogenic potential of sweet sorghum stems and indicated that the methane content was 64%. Takaki et al. [Citation52] experimented with the methane production from the vinasse resulting from ethanol fermentation process from sweet sorghum stalk juice. They obtained biogas with an average methane content of 62.7%. This study shows that solid residues from sorghum stalk ethanol fermentation process constitute a raw material for the production of biogas with a high methane content and comparable to that of raw stalk.
3.6. Economic analysis
Several studies have demonstrated the cost-effectiveness of sweet sorghum fermentable sugars bioconversion into ethanol. The cost of ethanol production estimated at 615.4 $/ton (0.49 $/L) was reported by Li et al. [Citation53] using advanced solid state fermentation technology. This study indicated that feedstock cost accounts for 78% of the total cost. A similar ethanol production cost of 0.58 $/L has been reported by Regassa and Wortmann [Citation54] for Florida’s sweet sorghum stems. These cost estimates were made including sweet sorghum production and harvesting, the input for biomass logistics, etc., which were the major expenses. For example, costs were estimated at 54.4% to 57.8% for biomass logistics input factor, and biomass conversion into ethanol accounted for about 38.4% to 42.2% [Citation55]. In fact, although considering all these factors, the cost of producing bioethanol from free sugars of sweet sorghum stems was found to be the lowest and cost-effective compared to other similar lignocellulosic biomass (sugar cane, wheat, cassava, beet, etc.) [Citation53]. The present study focused on sweet sorghum stalks that were agricultural by-products. Generally, in Africa, sorghum was grown mainly for seeds for human consumption, and the stalks were left in the fields in abundance. Therefore, in the economic analysis, the production and harvesting costs have not been considered, but only the costs of transport and other input factors related to the bioconversion of biomass, are considered. Thus, the cost of biomass logistics input factor (representing 54.4% to 57.8% of the total cost), given by the previous authors, would be significantly improved, making the proposed method of the present study more profitable. Moreover, the estimated bioconversion cost made by the authors mentioned above also includes costs linked to the biomass pre-treatment operations (juice extraction, hydrolysis, etc.). Biomass pre-treatment costs (electricity, water, enzymes, reagents, etc.) significantly increase the input factor of bioconversion process. For example, enzymatic hydrolysis leads to an increase in production cost estimated at 0.03–0.2 $/L of ethanol [Citation56,Citation57]. Similarly, an increase of 1.78–1.90 $/L depending on the type of pre-treatment applied was reported by Da Silva et al. [Citation58] for different pre-treatment processes. Devi et al. [Citation59] reported that the cost was 0.57 $/L of ethanol produced from lignocellulosic biomass through enzymatic and chemical hydrolysis. They found that, the major part of the production cost was attributed to the chemical pre-treatment and saccharification of the biomass. The direct fermentation of the shredded whole stems tested in this study made it possible to recover the free sugars while avoiding the juice extraction cost, reducing therefore the total operating cost and improving the economic profitability of the bioconversion process. On the other hand, the methanation of the subsequent solid residues from the direct fermentation has eliminated the costs of biomass hydrolysis. The energy recovery efficiency and the economic profitability could be significantly improved compared to the expensive traditional method of biomass hydrolysis.
4. Conclusion
Optimization of energy recovery process from sorghum stalks has been investigated. Physicochemical characterization of raw biomass reveals a high concentration of fermentable free sugars, composed of 199.22, 196.08, and 79.29 g/kg DM, respectively, for glucose, fructose, and sucrose. Its composition (31.74% dry matter) was total fibers, hemicellulose, cellulose, and lignin at, respectively, 63.50%, 16.96%, 18.25%, and 28.60% DM. Its calorific value was 17,144.17 kJ/kg DM. Energy recovery yields by single bioconversion processes of fermentation and methanization were, respectively, 6921.27 and 10,013.31 kJ/kg DM. Coupling processes significantly enhanced yield reaching 13,309.57 kJ/kg DM, with 77.63% energy recovery, i.e. 37.26% and 19.23% more compared to single fermentation and methanation, respectively.
Credit Authors Contribution Statement
Bakari Hamadou, Djomdi Djomdi, and Ruben Zieba Falama: Conceptualization, Methodology, Investigation, Software, Data curation, Writing – Original draft preparation. Fabrice Audonnet and Pierre Fontanille: Visualization, Investigation. Cedric Delattre and Guillaume Pierre: Software, Validation. Roger Djouldé Darnan and Pascal Dubessay: Meyhodology, Writing – Reviewing and Editing. Philippe Michaud and Gwendoline Christophe: Conceptualization, Methodology, and Supervision.
Acknowledgment
The data that support the findings of this study are available from the corresponding author [G.C.] upon reasonable request.
Disclosure statement
The authors declare that they have no known competing financial interests or personal relationships that could have appeared to influence the work reported in this paper.
Additional information
Funding
References
- IEA, 2021. World Energy Outlook 2021. IEA, Paris. https://www.iea.org/reports/world-energy-outlook-2021.
- IEA, 2022. World Energy Investment 2022. IEA, Paris. https://www.iea.org/reports/world-energy-investment-2022.
- Kevser M, Tekbaş M, Doğan M, et al. Nexus among biomass energy consumption, economic growth, and financial development: Evidence from selected 15 countries. Energy Rep. 2022;8:8372–8380. doi: 10.1016/j.egyr.2022.06.033
- Gaballah ES, El-Fatah AA, Xu C, et al. Enhancement of biogas production from rape straw using different co-pretreatment techniques and anaerobic co-digestion with cattle manure. Biores Technol. 2020;309:123311. doi: 10.1016/j.biortech.2020.123311
- Zhang CW, Wen H, Zheng J, et al. A combination of evaporation and chemical preservation for long-term storage of fresh sweet sorghum juice and subsequent bioethanol production. J Food Process Pres. 2018;42:13825. doi: 10.1111/jfpp.13825
- Bakari H, Djomdi, Ruben ZF, et al. Sorghum (Sorghum bicolor L. Moench) and its main parts (by-products) as promising sustainable sources of value-added ingredients. Waste Biomass Valor. 2022; doi: 10.1007/s12649-022-01992-7
- Saballos A. Development and utilization of sorghum as a bioenergy crop. In: Vermerris W, editor. Genetic improvement of bioenergy crops. New York, NY: Springer New York; 2008. pp. 211–248. doi: 10.1007/978-0-387-70805-8_8
- FAOSTAT, 2022. Food and agriculture data. Accessed 21 December 2022. http://www.fao.org/faostat/fr/#home
- Caputo AC, Palumbo M, Pelagagge PM, et al. Economics of biomass energy utilization in combustion and gasification plants: effects of logistic variables. Biomass Bioenergy. 2005;28(1):35–51. doi: 10.1016/j.biombioe.2004.04.009
- Antonopoulou G, Gavala HN, Skiadas IV, et al. Biofuels generation from sweet sorghum: fermentative hydrogen production and anaerobic digestion of the remaining biomass. Bioresour Technol. 2008;99(1):110–119. doi: 10.1016/j.biortech.2006.11.048
- Mamma D, Christakopoulos P, Koullas D, et al. An alternative approach to the bioconversion of sweet sorghum carbohydrates to ethanol. Biomass Bioenergy. 1995;8(2):99–103. doi: 10.1016/0961-9534(95)00006-S
- Głąb L, Sowiński J, Chmielewska J, et al. Comparison of the energy efficiency of methane and ethanol production from sweet sorghum (Sorghum bicolor (L.) Moench) with a variety of feedstock management technologies. Biomass Bioenergy. 2019;129:105332. doi: 10.1016/j.biombioe.2019.105332
- Brown RC, Brown TR. 2014. Biorenewable resources: engineering new products from agriculture. 2nd ed. Lowa: John Wiley & Sons; doi: 10.1002/9781118524985
- Appiah-Nkansah NB, Li J, Rooney W, et al. A review of sweet sorghum as a viable renewable bioenergy crop and its techno-economic analysis. Renewable Energy. 2019;143:1121–1132. doi: 10.1016/j.renene.2019.05.066
- Venturin B, Frumi CA, Scapini T, et al. Effect of pretreatments on corn stalk chemical properties for biogas production purposes. Bioresour Technol. 2018;266:116–124. doi: 10.1016/j.biortech.2018.06.069
- Mamata S, Beom SK. Current developments in lignocellulosic biomass conversion into biofuels using nanobiotechnology approach. Energies. 2020;13(20):5300. doi: 10.3390/en13205300
- Manikandan S, Vickram S, Sirohi R, et al. Critical review of biochemical pathways to transformation of waste and biomass into bioenergy. Biores Technol. 2023;372:128679. doi: 10.1016/j.biortech.2023.128679
- Mamata S, Aarti RD, Beom SK. Cellulase mimicking nanomaterial-assisted cellulose hydrolysis for enhanced bioethanol fermentation: an emerging sustainable approach. Green Chem. 2021;23(14):5064–5081. doi: 10.1039/D1GC01239H
- Mamata S, Beom SK. Lignin valorization using biological approach. Biotechnol Appl Biochem. 2020;68(3):459–468. doi: 10.1002/bab.1995
- AOAC A. Official methods of analysis 16th Ed. Association of Official Analytical Chemists. Washington DC, USA: Sci. Educ; 1995.
- Van Soest PJ. The use of detergents in the analysis of fibrous feeds: II. A rapid method for the determination of fiber and lignin. Official Agri Chem. 1963;46(5):829. doi: 10.1093/jaoac/46.5.829
- Gutfinger T. Polyphenols in olive oils. J Am Oil Chem Soc. 1981;58(11):966–968. doi: 10.1007/BF02659771
- Outlaw WH, Mitchell CT. Sucrose. In: Bergmeyer HU, editor Methods of enzymatic analysis. Vol. VI. 3rd ed. Cambridge, UK: VCH Publishers (UK) Ltd; 1988. pp. 96–103.
- Beutler HO. D-Fructose. In: Bergmeyer HU, editor Methods of enzymatic analysis. Vol. VI. 3rd ed. Cambridge, UK: VCH Publishers (UK) Ltd; 1988. pp. 321–327.
- Kunst A, Draeger B, Ziegenhorn J. D-Glucose. In: Bergmeyer HU, editor Methods of enzymatic analysis. Vol. VI. 3rd ed. Cambridge, UK: VCH Publishers (UK) Ltd; 1988. pp. 163–172.
- Bahry H, Pons A, Abdallah R, et al. Valorization of carob waste: Definition of a second-generation bioethanol production process. Bioresour Technol Elsevier. 2017;235:25–34. doi: 10.1016/j.biortech.2017.03.056
- Kristiansen B. Integrated design of a fermentation plant: The production of Baker’s yeast. Weinheim, Germany; New York, NY, USA: VCH; 1994.
- Dubois M, Gilles KA, Hamilton JK, et al. Colorimetric method for determination of sugar and related substances. Anal Chem. 1956;28:350–356. doi: 10.1021/ac60111a017
- Ribeiro T, Cresson R, Pommier S, et al. Measurement of biochemical methane potential of heterogeneous solid substrates: results of a two-phase French inter-laboratory study. Water. 2020;12:2814. doi: 10.3390/w12102814
- Crépeau M, Khelifi M, Vanasse A, et al. Compressive forces and harvest time effects on sugars and juice extracted from sweet pearl millet and sweet sorghum. Trans ASABE. 2013;56(5):1665–1671. doi: 10.13031/trans.56.10271
- Ostovareh S, Karimi K, Zamani A. Efficient conversion of sweet sorghum stalks to biogas and ethanol using organosolv pretreatment. Ind Crops Prod. 2015;66:170–177. doi: 10.1016/j.indcrop.2014.12.023
- Billa E, Koullas DP, Monties B, et al. Structure and composition of sweet sorghum stalk components. Ind Crops Prod. 1997;6(3–4):297–302. doi: 10.1016/S0926-6690(97)00031-9
- Qinglian W, Xian B, Wanqian G, et al. Medium chain carboxylic acids production from waste biomass: Current advances and perspectives. Biotechnol Adv. 2019;37(5):599–615. doi: 10.1016/j.biotechadv.2019.03.003
- Sipos B, Récey J, Somorai Z, et al. Sweet sorghum as feedstock for ethanol production: Enzymatic hydrolysis of steam-pretreated bagasse. Appl Biochem Biotech. 2009;153(1–3):151–162. doi: 10.1007/s12010-008-8423-9
- Djomdi HB, Klang MJ, Djoulde DR, et al. Extraction performance of juice and bioethanol production from sweet sorghum (Sorghum bicolor (L.) Moench). Global J Eng Sci Res Manage. 2019;6(7):57–66. doi: 10.5281/zenodo.3357842
- Daniel EE, Ajit KM, Mark LJ, et al. Evaluation of three cultivars of sweet sorghum as feedstocks for ethanol production in the Southeast United States. Heliyon. 2017;3(12):e00490. doi: 10.1016/j.heliyon.2017
- Prasad S, Singh A, Jain N, et al. Ethanol production from sweet sorghum syrup for utilization as automotive fuel in India. Energy Fuels. 2007;21:2415–2420. doi: 10.1021/ef060328z
- Serna-Saldívar SO, Chuck-Hernández C, Pérez-Carrillo E, et al. Sorghum as a multifunctional crop for the production of fuel ethanol: Current status and future trends. Bioethanol, Prof. Marco Aurelio Pinheiro Lima (Ed.), ISBN: 978-953-51-0008-9. InTech. 2012;51–74. ISBN: 978-953-51-0008-9. http://www.intechopen.com/books/bioethanol/sorghum-as-a-multifunctional-crop-for-the-production-of-fuel-ethanol-current-status-and-future-tren
- Amaducci S, Monti A, Venturi G. Non-structural carbohydrates and fibre components in sweet and fibre sorghum as affected by low and normal input techniques. Ind Crops Prod. 2004;20:111–118. doi: 10.1016/j.indcrop.2003.12.016
- Khalil SRA, Abdelhafez AA, Amer EAM. Evaluation of bioethanol production from juice and bagasse of some sweet sorghum varieties. Ann Agric Sci. 2015;60:317–324. doi: 10.1016/j.aoas.2015.10.005
- Ratnavathi CV, Kalyana SC, Komala V.V, et al. Sweet sorghum as feedstock for biofuel production: A review. Sugar Tech. 2011;13(4):399–407. doi: 10.1007/s12355-011-0112-2
- Sereme A, Kuoda-Bonafos M, Nacro M. Phenolic compounds in Sorghum caudatum tissues during plant development. Biomass Bioenergy. 1993;4(1):69–71. doi: 10.1016/0961-9534(93)90028-3
- Hao C, Xuejiao T, Qunli Y, et al. Sweet sorghum stalks extract has antimicrobial activity. Ind Crops Prod. 2021;170:113746. doi: 10.1016/j.indcrop.2021.113746
- Bakari H, Djomdi, Ruben ZF, et al. Optimization of bioethanol production after enzymatic treatment of sweet sorghum stalks. Waste Biomass Valor. 2023; doi: 10.1007/s12649-022-02026-y
- Luna P, Risfaheri H, Charalampopoulos D, et al. Fractionation of carbohydrate polymers from Indonesian sorghum by-products. Food Bioprod Process. 2022;135:114–122. doi: 10.1016/j.fbp.2022.07.007
- Kim M, Day DF. Composition of sugar cane, energy cane, and sweet sorghum suitable for ethanol production at Louisiana sugar mills. J Ind Microbiol Biotechnol. 2011;38(7):803–807. doi: 10.1007/s10295-010-0812-8
- Ratnavathi CV, Suresh K, Vijay KBS, et al. Study on genotypic variation for ethanol production from sweet sorghum juice. Biomass Bioenergy. 2010;34(7):947–952. doi: 10.1016/j.biombioe.2010.02.002
- Laopaiboon L, Pornthap T, Jaisil P, et al. Ethanol production from sweet sorghum juice in batch and fed-batch fermentations by Saccharomyces cerevisiae. World J Microbiol Biotechnol. 2007;23(10):1497–1501. doi: 10.1007/s11274-007-9383-x
- Ban J, Yu J, Xu Z, et al. Ethanol production from sweet sorghum residual. Front Chem Sci Eng. 2008;2(4):452–455. doi: 10.1007/s11705-008-0072-6
- Herrmann C, Heiermann M, Idler C. Effects of ensiling, silage additives and storage period on methane formation of biogas crops. Bioresour Technol. 2011;102(8):5153–5161. doi: 10.1016/j.biortech.2011.01.012
- Theuretzbacher F, Bauer A, Lizasoain J, et al. Potential of different Sorghum bicolor (L. Moench) varieties for combined ethanol and biogas production in the Pannonian climate of Austria. Energy. 2013;55:107–113. doi: 10.1016/j.energy.2013.04.020
- Takaki M, Tan L, Murakami T, et al. Production of biofuels from sweet sorghum juice via ethanol–methane two-stage fermentation. Ind Crops Prod. 2014;63:329–336. doi: 10.1016/j.indcrop.2014.10.009
- Li S, Li G, Zhang L, et al. A demonstration study of ethanol production from sweet sorghum stems with advanced solid state fermentation technology. Appl Energy. 2013;102:260–265. doi: 10.1016/j.apenergy.2012.09.060
- Regassa TH, Wortmann CS. Sweet sorghum as a bioenergy crop: Literature review. Biomass Bioenergy. 2014;64:348–355. doi: 10.1016/j.biombioe.2014.03.052
- Yang X, Li M, Liu H, et al. Technical feasibility and comprehensive sustainability assessment of sweet sorghum for bioethanol production in China. Sustainability. 2018;10(3):731. doi: 10.3390/su10030731
- Kandasamy M, Hamawand I, Bowtell L, et al. Investigation of ethanol production potential from lignocellulosic material without enzymatic hydrolysis using the ultrasound technique. Energies. 2017;10(1):62. doi: 10.3390/en10010062
- Klein-Marcuschamer D, Oleskowicz-Popiel P, Simmons BA, et al. The challenge of enzyme cost in the production of lignocellulosic biofuels. Biotechnol Bioeng. 2012;109(4):1083–1087. doi: 10.1002/bit.24370
- Da Silva ARG, Ortega CET, Rong B-G. Techno-economic analysis of different pretreatment processes for lignocellulosic-based bioethanol production. Biores Technol. 2016;218:561–570. doi: 10.1016/j.biortech.2016.07.007
- Devi A, Niazi A, Ramteke M, et al. Techno-economic analysis of ethanol production from lignocellulosic biomass–a comparison of fermentation, thermo catalytic, and chemocatalytic technologies. Bioprocess Biosyst Eng. 2021;44:1093–1107. doi: 10.1007/s00449-020-02504-4