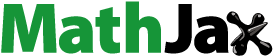
ABSTRACT
Fermentation effluents from organic wastes contain simple organic acids and ethanol, which are good electron sources for exoelectrogenic bacteria, and hence are considered a promising substrate for hydrogen production in microbial electrolysis cells (MECs). These fermentation products have different mechanisms and thermodynamics for their anaerobic oxidation, and therefore the composition of fermentation effluent significantly influences MEC performance. This study examined the microbial electrolysis of a synthetic fermentation effluent (containing acetate, propionate, butyrate, lactate, and ethanol) in two-chamber MECs fitted with either a proton exchange membrane (PEM) or an anion exchange membrane (AEM), with a focus on the utilization preference between the electron sources present in the effluent. Throughout the eight cycles of repeated batch operation with an applied voltage of 0.8 V, the AEM-MECs consistently outperformed the PEM-MECs in terms of organic removal, current generation, and hydrogen production. The highest hydrogen yield achieved for AEM-MECs was 1.26 L/g chemical oxygen demand (COD) fed (approximately 90% of the theoretical maximum), which was nearly double the yield for PEM-MECs (0.68 L/g COD fed). The superior performance of AEM-MECs was attributed to the greater pH imbalance and more acidic anodic pH in PEM-MECs (5.5–6.0), disrupting anodic respiration. Although butyrate is more thermodynamically favorable than propionate for anaerobic oxidation, butyrate was the least favored electron source, followed by propionate, in both AEM- and PEM-MECs, while ethanol and lactate were completely consumed. Further research is needed to better comprehend the preferences for different electron sources in fermentation effluents and enhance their microbial electrolysis.
1. Introduction
Microbial electrolysis cells (MECs) provide an attractive bioelectrochemical platform for converting organic matter to green hydrogen. In an MEC, organic matter is oxidized to CO2 by exoelectrogenic bacteria on the anode, and released protons and electrons to form hydrogen gas at the cathode with a small external energy input (typical applied voltage ≤1 V) [Citation1–4]. If this input energy is provided from carbon-neutral energy source such as wind, solar, and tidal powers, the MEC technology can establish a feasible hydrogen economy. The transfer of protons (or hydroxide ions) between electrodes occurs through the ion exchange membrane physically separating the anode and cathode sides in a two-chamber MEC or by simple diffusion in the electrolyte in a single-chamber MEC. The single-chamber design is attractive since it does not need expensive ion exchange membranes. However, single-chamber MECs are not suitable to achieve high hydrogen purity and productivity mainly due to the consumption of hydrogen by hydrogenotrophic methanogens. In contrast, the two-chamber design allows the production high-purity hydrogen by separating the bioanode (exoelectrogenic oxidation of organic matter) from the abiotic cathode (hydrogen evolution) [Citation5,Citation6].
An important element to ensure the practical applicability of MECs is to find low-cost sustainable substrates suitable for exoelectrogenic respiration. Waste streams rich in organic matter, such as sugar-processing wastewater, food waste fermentation effluent, landfill leachate, and distillery wastewater, are good candidates for alternative MEC substrates [Citation7–11]. However, real wastewaters generally contain relatively complex organic materials and considerable amounts of suspended particles, which causes performance and stability degradation related to membrane fouling and pH imbalance, especially in two-chamber MECs [Citation12, Citation13], and wastewater-fed MECs are still far away from practical application.
Dark fermentation of organic wastes, such as food waste, produces mainly simple organic acids and alcohols, which are readily utilized by exoelectrogenic bacteria for anodic respiration in MECs [Citation14], and therefore the fermentation effluent can be a promising substrate for MECs. Fermentation effluents typically contain acetate, propionate, butyrate, and lactate as the major organic acids along with ethanol, and their composition varies greatly depending on the feedstocks and operating conditions such as hydraulic retention time, organic loading rate, pH, and temperature [Citation11, Citation15, Citation16]. The compositional variation can affect the utilizability of fermentation effluents and hence the performance of MECs since the fermentation products are used and converted to hydrogen with different efficiencies in MECs [Citation17]. Accordingly, many previous studies have examined major organic acids, especially acetate, propionate, and butyrate, as MEC substrates and reported different results on their utilization and conversion efficiencies. For example, Escapa et al. [Citation18] and Yang et al. [Citation19] reported that butyrate is more readily consumed than propionate, while Torres et al. [Citation20] reported a higher reaction rate with propionate than with butyrate. Lactate and ethanol have been relatively less studied as substrates for hydrogen production in MECs [Citation17, Citation21], although they are also major fermentation products and often occur in high concentrations in industrial effluents, for example, from food and beverage processing [Citation22, Citation23].
Different fermentation products coexist in fermentation effluents, and thus understanding the differences in their utilization rates and hydrogen-producing efficiencies can help to improve the performance and application of fermentation effluent-fed MECs. However, not much has been reported on the utilization preference of MECs for different fermentation products in a single mixture. Rivera et al. [Citation24] examined two synthetic fermentation effluents (SFE) in two-chamber MECs; however, the effluents did not contain lactate and/or ethanol, and the focus was given to optimizing the operating conditions rather than assessing the preference for electron sources. Paz-Mireles et al. [Citation25] examined the effects of adding lactate-ethanol mixtures on the performance of two-chamber MECs fed with an acetate-propionate-butyrate mixture as the base substrate; however, they focused on the effects on the reaction rate, with little attention to the utilization preference between electron sources.
This study focuses on comparing the utilization and conversion properties of the major fermentation products in MECs fed with their mixture. For a more comprehensive comparison, two-chamber MECs equipped with two different types of ion exchange membranes, i.e. anion exchange membrane (AEM) or proton exchange membrane (PEM), were operated in parallel with an equal chemical oxygen demand (COD) concentration mixture of acetate, propionate, butyrate, lactate, and ethanol. The MECs were closely monitored during the operation for the consumption of organic acids and ethanol as well as bioelectrochemical performance.
2. Materials and methods
2.1. Microbial electrolysis cell construction
Two-chamber cube cells with an ion exchange membrane sandwiched between anode and cathode were used to construct the experimental MECs (). The anode was made up of a rectangular piece of carbon felt (50 mm length × 45 mm width × 3.18 mm thickness, Alfa Aesar, USA), which was affixed to a 304 stainless steel plate current collector using silver paste. The carbon felt was cleaned with acetone by ultrasonication for an hour before use to remove any organic impurities on the surface [Citation26, Citation27]. Subsequently, it was soaked in 10% (v/v) nitric acid for 12 h to improve hydrophilicity, rinsed with deionized water, and then dried overnight at 110°C [Citation28]. A perforated titanium plate (50 mm length × 45 mm width × 1.5 mm thickness) coated with 2 g/cm2 of platinum served as the cathode and current collector. The cathode chamber was equipped with an Ag/AgCl reference electrode (+0.199 V vs. standard hydrogen electrode, RE-1B, ALS, Japan) to measure the cathode potential every 10 min using a multimeter (DAQ 6510, Keithley, USA) [Citation29]. The two-chamber MECs were installed with either a PEM (70 mm × 70 mm, Nafion 117, Chemours, USA) and an AEM (70 mm × 70 mm, AMI-7001, Membrane International Inc., USA). The PEM was pretreated by soaking it successively in 5% H2O2, ultrapure water, 0.5 M of H2SO4, and ultrapure water at 80°C for one hour each [Citation30], while the AEM was immersed in a 5% NaCl solution for 12 h [Citation31].
2.2. Microbial electrolysis cell operation
Two sets of duplicate MECs, each consisting of two MECs with either an AEM (AEM-MECs) or a PEM (PEM-MECs), were operated for eight consecutive batch cycles at room temperature over a period of 51 d. An external voltage of 0.8 V was applied between the anode and cathode using a power supply (LW-K3010D, China) [Citation32, Citation33]. The anode chamber was inoculated at 50% (v/v) with the effluent from lab-scale upflow anaerobic sludge blanket reactors fed with expired rice wine. The inoculum had a suspended solids concentration of 185 ± 77 mg/L, with 130 ± 42 mg/L of it being volatile suspended solids (i.e. organic particles). The remaining half of the anode chamber was filled with 100 mM phosphate buffer solution (PBS, pH 7.5), which was supplemented with nutrients and minerals as described previously [Citation34]. The cathode chamber was fully filled with the same PBS without nutrients and minerals.
The high-concentration stock of SFE used as the substrate for the experimental MECs was prepared by mixing equal COD loads of acetate, propionate, butyrate, lactate, and ethanol to a total concentration of 100 g COD/L. At time 0, a 2.6-mL aliquot of the SFE stock was injected into the anode chamber to a create an initial substrate concentration of 1.5 g COD/L. To ensure successful inoculation and acclimation of exoelectrogenic bacteria, at the end of the first and second cycles, the anodic biomass was allowed to settle by gravity for approximately 10 min before replacing 50% (v/v) of the anolyte with fresh anolyte containing the substrate the next cycle of operation. From the third cycle onwards, the anolyte (including the suspended anodic biomass) was fully replaced at the end of each cycle before starting the next cycle. A batch cycle was lasted until the cumulative hydrogen production reached a plateau (approximately seven days per cycle). Before beginning each cycle, both anodic and cathodic chambers filled with electrolyte were purged with ultra-pure nitrogen gas for 5 min to ensure an anaerobic environment and remove any residual gas from the previous cycle. The MECs were operated without pH adjustments.
2.3. Analytical methods
Gas production from the cathode chamber was measured in real time using a respirometer (EET BRS-100, Eco-Environment Technology, Korea) that was connected to the headspace of the chamber. The respirometer was calibrated at least three times before each cycle to ensure accuracy and minimize measurement errors. At the end of each cycle, a 1-mL gas sample was taken from the headspace using a 1.5-mL gas-tight syringe (Hamilton Sample Lock Syringe #1002, USA) and analyzed for gas composition (H2, CH4, and CO2) using a gas chromatograph (7820A, Agilent, USA) coupled with a thermal conductivity detector and a ShinCarbon ST column (Restek, USA). Volatile fatty acids (C2–C7) and ethanol were measured using another 7820A gas chromatograph equipped with a flame ionization detector and an Innowax column (Agilent, USA). COD was colorimetrically determined using the HS-COD-MR kit (HUMAS Co., Korea). Lactate was measured using the D-/L-Lactate assay kit (Megazyme, Ireland) with a spectrophotometer (UV-1800, Shimadzu, Japan). The samples for measuring organic acids, ethanol, and soluble COD were filtered using a 0.45-μm pore size filter prior to analysis. All chemical analyses above were replicated at least twice.
A 10 Ω resistor was connected in series between the cathode and the negative lead of the power supply in the circuit of each experimental MEC, and the voltage across the resistor was monitored at 10-min intervals using a multimeter (DAQ 6510, Keithley, USA) to calculate the current using Ohm’s Law (V = IR). The current density (A/m2) was determined based on the projected surface area of the cathode (20 cm2), and the maximum current density for each cycle was obtained by averaging the 10 highest current densities recorded [Citation26].
2.4. Calculations
The Coulombic efficiency () was calculated using the following equation [Citation11]:
where is the total Coulomb production calculated by integrating the current over time,
is Faraday’s constant (96,485 C/mol e−),
is the moles of electrons produced per mole of oxygen (4 mol e−/mol O2), and
is the moles of COD removed. The cathodic hydrogen recovery (
) was calculated according to the following equation [Citation11]:
where is the moles of hydrogen produced, and
is the moles of electrons consumed per mole of hydrogen produced (2 mol e−/mol H2). The overall hydrogen recovery (
) was calculated as
.
The observed hydrogen production profiles were modeled using the following modified Gompertz equation [Citation35]:
where is the cumulative hydrogen production [Citation36] at time
(d),
is the tial (mL H2/L anode chamber),
is the lag time (d),
is the maximum hydrogen production rate (mL/L anode chamber∙d), and
is the operation time (d). The modeling was carried out using the Origin Pro 2020 software (OriginLab, USA).
3. Results and discussion
3.1. Hydrogen and current productions
This study examined the MEC performance with a synthetic fermentation effluent containing acetate, propionate, butyrate, lactate, and ethanol with a focus on the conversion preference between the organic substrates using MECs equipped with either AEM or PEM. The cumulative hydrogen production per subculture cycle of 7 days (HC) increased steadily with the number of cycles and reached a plateau of approximately 288 mL in the last two cycles (no significant difference between the seventh and eighth cycles; t-test, p > 0.05) in AEM-MECs (). Meanwhile, the HC in PEM-MECs did not increase significantly after the third cycle and rather decreased in the last two cycles, with its maximum of 122 mL being recorded in the sixth cycle. AEM-MECs showed significantly higher HC values than PEM-MECs throughout the subcultures, and accordingly the hydrogen yield and recovery also remained significantly higher in AEM-MECs (>2-fold in the last two cycles; ). In AEM-MECs, the hydrogen yield reached up to 1.26 L/g COD, which is approximately 90% of the theoretical maximum of 1.40 L/g COD [Citation37], in the last cycle. In contrast, the highest hydrogen yield in PEM-MECs was only 0.68 L/g COD, which was recorded in the sixth cycle. The maximum hydrogen production rate estimated by the Gompertz model was also much higher in AEM-MECs (>1.6-fold in the last two cycles), and correspondingly the maximum current density, which indicates the peak rate of electrochemical reaction, was significantly greater in AEM-MECs (>1.3-fold in the last two cycles). The hydrogen content in the gas recovered from the cathode remained consistently above 94% throughout the experimental cycles in both AEM- and PEM-MECs. These results clearly demonstrate that using AEM is significantly better than using PEM in two-chamber MECs for producing hydrogen from fermentation effluents, in terms of hydrogen productivity.
Figure 2. Hydrogen (a) and current (b) production profiles during repeated batch operation of experimental MECs. Asterisk denotes that the measurement of cathodic hydrogen production in AEM-MECs during the sixth cycle was compromised by mechanical issues with respirometer.

Figure 3. Performance comparison between experimental AEM- and PEM-MECs during repeated batch operation: (a) maximum hydrogen production rate, (b) maximum current density, (c) coulombic efficiency, (d) cathodic hydrogen recovery, (e) COD removal efficiency, and (F) hydrogen yield and recovery. Asterisks denote that the measurement of cathodic hydrogen production in AEM-MECs during the sixth cycle was compromised by mechanical issues with respirometer. Data from the first two cycles run for microbial inoculation and acclimation were excluded from comparison.

In both AEM- and PEM-MECs, significant current generation and hydrogen evolution were observed from the first cycle after a two-day lag time, and relatively high and stable coulombic efficiency and coulomb production were maintained after the third cycle (). In addition, the MECs produced hydrogen immediately without a lag time from the second cycle. These results indicate that the inoculation and acclimation of exoelectrogenic bacteria on the anodes (i.e. the development of bioanodes) over the first three cycles (see Subsection 2.2 for details) were successful [Citation38]. The average value of the maximum current density for the fourth to eighth cycles was approximately 1.3-fold higher in AEM-MECs (5.1 ± 0.4 A/m2) than in PEM-MECs (3.9 ± 0.3 A/m2). These values are comparable to those reported in previous studies using two-chamber MECs. Cario et al. [Citation39] reported a maximum current density of approximately 5 A/m2 in acetate-fed AEM-MECs equipped with carbon felt anodes and Pt/C-treated stainless steel mesh cathodes at an applied voltage of 0.8 V. Apostolopoulos et al. [Citation40] observed a peak current density of approximately 3.8 A/m2 in PEM-MECs installed with carbon paper anodes and Pt-coated carbon cloth cathodes, using a mixture of volatile fatty acids (C2–C6) as the substrate at an applied voltage of 0.9 V.
It is worth noting that the hydrogen production rate and yield were significantly greater in AEM-MECs than in PEM-MECs although both maintained comparably high levels of coulombic efficiency (≥96% in the last two cycles; ). The superior performance of AEM-MECs were attributed to their significantly higher COD removal efficiency (i.e. more electrons to be transferred to the anodes) and cathodic hydrogen recovery (i.e. higher proportion of cathodic electrons toward hydrogen evolution) compared to PEM-MECs. Correspondingly, AEM-MECs showed markedly higher coulomb production than PEM-MECs (>1.4-fold in the last two cycles). The differences in cathodic hydrogen recovery between AEM-MECs (approximately 85%) and PEM-MECs (approximately 51%) were particularly pronounced in the last two cycles (>1.7-fold greater in AEM-MECs). These results can be related to the pH imbalance between the anode and cathode sides, which was greater in PEM-MECs compared to AEM-MECs especially in the later cycles (). An imbalance in pH levels across the membrane is a significant factor that reduces the performance of two-chamber MECs [Citation41]. Furthermore, the more acidic anodic pH in PEM-MECs (≤6.0) likely contributed to their poorer performance compared to AEM-MECs, given that the optimal pH for most exoelectrogenic bacteria is between 6 and 7 [Citation42]. The effect of pH imbalance on the MEC performance will be discussed in detail in subsection 3.2. Another factor that could lower the cathodic hydrogen recovery is the loss of hydrogen through membrane permeation from the cathode to anode sides [Citation43].
3.2. pH imbalance
The anodic pH measured at the end of each cycle was consistently higher in AEM-MECs (6.2–6.7) compared to PEM-MECs (5.5–6.0) throughout the experiment (). The cathodic pH also remained higher in AEM-MECs (11.1–12.1) than in PEM-MECs (10.8–11.6) during the first five cycles, but it was the same (11.9–12.1) in both in the following cycles. Consequently, the pH imbalance between anolyte and catholyte across the ion exchange membrane was significantly greater in PEM-MECs (5.9–6.6) compared to AEM-MECs (5.4–5.6) during the last three cycles. A large pH difference between acidified anolyte and alkalized catholyte results in significant reductions in current generation and hydrogen production by inducing a high concentration overpotential, which increases the energy needed for hydrogen evolution [Citation44, Citation45]. Therefore, the higher pH imbalance in PEM-MECs compared to AEM-MECs appears to have contributed significantly to the poorer cathodic hydrogen recovery in the former, which was more pronounced in the last three cycles (). Although it is difficult to quantitatively determine all electron sinks other than hydrogen evolution at the cathode, pH imbalance must have had a significant impact on the flow of cathodic electrons. Another point to consider is that the anodic pH level in PEM-MECs was as low as 5.5–6.0, which is not favorable for the growth of exoelectrogenic bacteria. The acidic anodic pH likely inhibited exoelectrogenic electron transfer to the anode, thereby reducing MEC performance [Citation46].
The increase in cathodic pH and decrease in anodic pH have been extensively reported in two-chamber MECs [Citation41, Citation47–49]. In PEM-MECs, pH imbalance can occur due to slower proton transport across the membrane than the rates of proton generation (at the anode) or consumption (at the cathode), and the undesired transport of other cations commonly present in electrolytes (e.g. Na+, K+, and NH4+) exacerbates the problem by interfering with proton transport [Citation50]. Typically, the electrolyte containing 105 times higher concentration of other cations than that of protons could provoke slower proton transport rate by transferring other cations more easily instead of protons to balance the charge [Citation51]. The less acidic anodic pH in AEM-MECs compared to PEM-MECs can be attributed to the more efficient transport of hydroxide ions from the cathode to anode sides to balance electroneutrality in AEM-MECs, as compared to the transport of protons from the anode to cathode sides in PEM-MECs [Citation52]. This difference appears to have contributed to the larger pH imbalance and poorer performance of PEM-MECs compared to AEM-MECs ().
Moreover, in AEM-MECs, the transmembrane transport of protons can be facilitated with the assistance of pH buffers such as phosphate or carbonate [Citation46]. For example, in PBS systems, as used in this study, HPO42− can be converted to H2PO4− by combining with protons produced at the anode, and then transported through AEM to the cathode side. The transported H2PO4− ions can release protons at the cathode by reverting back to HPO42− ions, which can then move back to the anode side [Citation46, Citation53]. This proton shuttling across AEM also likely helped, in part, to reduce the pH imbalance and thus minimize the performance loss in AEM-MECs [Citation54]. According to a recent study on MECs, it was found that an imbalance in pH levels between the anolyte and catholyte in two-chamber MECs can be addressed by utilizing a new cathode design. This innovative design does not require a liquid catholyte and instead incorporates closely spaced electrodes and an AEM [Citation51]. The study reported achieving a record-high current density and hydrogen production rate at a cell voltage of 0.79 V, suggesting that this vapor-fed design should be tested in MECs used for treating fermentation effluent to enhance MEC efficiency.
3.3. Electron source utilization
After the first three cycles of inoculating and acclimating the anodic microbial community, the AEM-MECs maintained significantly higher COD removal (≥95%) compared to the PEM-MECs (≤68%) throughout the entire experimental cycles (). This difference corresponds to the superior performance of the AEM-MECs to the PEM-MECs discussed above. Butyrate was the organic compound that remained the most in the anode chamber after the batch reaction, followed by propionate and then acetate, throughout the experimental cycles in both AEM- and PEM-MECs (). Meanwhile, lactate and ethanol were entirely removed and not detected in any of the cycles, indicating that they could be readily utilized as electron sources in the MECs, either directly or indirectly. Acetate is commonly known to be the most rapidly consumed electron source, followed by butyrate and then propionate, in MECs [Citation20, Citation55]. The detection of a small amount of residual acetate is likely due to its production during the degradation of other electron sources in the SFE ().
Figure 5. Residual concentrations of organic electron sources in each cycle in (a) AEM-MECs and (b) PEM-MECs.

Table 1. Biochemical reactions involved in exoelectrogenic utilization of fermentation products under anaerobic conditions.
Note that the utilization of butyrate as an electron source was significantly less efficient than other sources, even propionate, although the anaerobic degradation of propionate is less thermodynamically favorable than that of butyrate. The preferred utilization of butyrate over propionate in MECs has been reported in numerous studies [Citation18,Citation19,Citation61,Citation62], but there are also many studies that report the opposite result [Citation20,Citation35,Citation55,Citation63]. These conflicting observations suggest that there are other factors beyond thermodynamics, such as energy investment [Citation35], that influence the utilization preference between different electron sources. The preferred utilization of propionate over butyrate observed in this study may also be attributed to the potential existence of specialized exoelectrogenic bacteria, such as Geobacter anodireducens, capable of directly utilizing propionate for current generation [Citation56,Citation64]. Lactate, which has a similar molecular structure to propionate, can also be directly converted to electrical current by some Geobacter species [Citation53]. Lactate can be consumed directly by exoelectrogenic bacteria (e.g. Geobacter sulfurreducens PCA) without electron shuttle through intracellular conversion of lactate to acetate and hydrogen, followed by their oxidation to electrons, and this overall oxidation reaction is known to be highly spontaneous process [Citation65]. Although the anodic microbial community was not analyzed in this study, it is possible that exoelectrogenic bacteria, which can directly utilize propionate (and lactate), were active and helped to facilitate the utilization of propionate (and lactate) in the experimental MECs. The facilitated propionate utilization would have benefited the syntrophic exoelectrogenic utilization of lactate via propionate (). These results suggest that MECs can have different preferences for various electron sources, which warrants further research on the microbial interactions and their effects on the conversion of electron sources to current and then hydrogen in MECs.
Another point to consider regarding the exoelectrogenic propionate utilization is the effect of pH. Syntrophic degradation of propionate can occur through two pathways: the acetate/H2 pathway, which is more favorable at a pH below 6.37, or the acetate/formate pathway, which is more favorable at a pH above that [Citation64]. Given that the latter pathway is more energetically favorable than the former (), the less acidic anodic pH of AEM-MECs (6.2–6.7) compared to PEM-MECs (5.5–6.0) likely contributed to the more efficient degradation of propionate in the AEM-MECs (). The difference in the utilizability of propionate, which is a relatively challenging electron source, can be linked to the performance difference in current and hydrogen production observed between the AEM- and PEM-MECs.
Unlike propionate and lactate, there is currently no evidence that exoelectrogenic bacteria can directly utilize butyrate and ethanol to generate electrical current. It is believed that these compounds can only be converted to current indirectly via acetate in MECs [Citation66, Citation67]. Since the anaerobic oxidation of butyrate or ethanol to acetate is thermodynamically unfavorable (), their exoelectrogenic utilization requires syntrophic relationship with hydrogen scavengers [Citation66]. However, the significantly lower Gibbs free energy of ethanol oxidation compared to butyrate oxidation makes ethanol a more favorable electron source than butyrate. This thermodynamic advantage of ethanol is well reflected in the absence of residual ethanol in every cycle in both AEM- and PEM-MECs ().
While this study presents intriguing findings regarding the choice of organic compounds in SFE depending on the type of membrane employed, there are a few limitations associated with the current experiments. First, a fermentation effluent which was artificially synthesized was used here. There were some previous studies which utilized the actual fermentation effluent in MECs or MFCs generated from food wastewater, landfill leachate, and corn stalk as substrate for fermentation processes [Citation11, Citation55, Citation68]. Incorporating the actual fermentation effluent in future studies would be beneficial in reducing the operating costs and improving the practicality and commercial feasibility of MECs. Additionally, it is important to note that the current experiments were conducted using small lab-scale reactors with a working volume of 200 mL. This limited scale makes it challenging to accurately predict and scale up the process to larger industrial-scale bioreactors. Once process optimization and a comprehensive understanding of the dynamics of MECs with mixed fermentation effluent have been achieved through lab-scale research, the technology can be scaled up to pilot-scale reactors for the treatment of actual fermentation effluent, as demonstrated in previous works involving other actual organic wastewater [Citation69–71]. It is worth noting that for scaling up the two-chamber MEC technology, the choice of ion exchange membrane (e.g. PEM and AEM) becomes a critical factor that influences the cost. Although the ion exchange membrane accounts for up to 40% of the overall cost of MECs, it is inevitable to use a membrane to get a high-purity hydrogen from MECs [Citation36]. The AEM (e.g. AMI-7001, ~80 USD/m2) is approximately 18-folds cheaper than PEM (e.g. Nafion 117, ~1,400 USD/m2) [Citation72]. Given that nearly double higher hydrogen yield in AEM than PEM in our study, using AEM can enhance the cost-effectivity of MECs.
4. Conclusions
Exoelectrogenic degradation of SFE and its conversion to hydrogen was significantly more efficient in AEM-MECs than PEM-MECs. This performance difference was attributed to the larger pH imbalance and more acidic anodic pH in PEM-MECs, which inhibit electron transfer and growth of exoelectrogenic bacteria. Butyrate was the least favored electron source in both MECs, followed by propionate. The preference for utilizing propionate over butyrate implies that factors beyond thermodynamics, such as energy investment and microbial community are involved in determining the utilization preference for different electron sources. Therefore, a deeper understanding of the varying preferences for different electron sources is required.
Author contributions statement
Yunjeong Choi and Danbee Kim: Formal analysis, Investigation, Data curation, Writing – Original draft, Visualization. Hyungmin Choi and Junho Cha: Investigation, Formal analysis, Writing – Original draft. Gahyun Baek: Writing – Review & editing, Supervision. Changsoo Lee: Conceptualization, Funding acquisition, Writing – Review & editing, Supervision. All authors approved the final manuscript for submission (and publication) and agreed to be accountable for all aspects of the work.
All authors meet the criteria for authorship as per the ICMJE criteria.
Disclosure statement
No potential conflict of interest was reported by the authors.
Data availability statement
The data that support the findings of this study are available from the corresponding author, GH Baek and CS Lee, upon reasonable request.
Additional information
Funding
References
- Park S-G, Rajesh PP, Sim Y-U, et al. Addressing scale-up challenges and enhancement in performance of hydrogen-producing microbial electrolysis cell through electrode modifications. Energy Rep. 2022;8:2726–446. doi: 10.1016/j.egyr.2022.01.198
- Amin MM, Arvin A, Feizi A, et al. Meta-analysis of bioenergy recovery and anaerobic digestion in integrated systems of anaerobic digestion and microbial electrolysis cell. Biochem Eng J. 2022;178:108301. doi: 10.1016/j.bej.2021.108301
- Tran TTH, Nguyen PKT. Enhanced hydrogen production from water hyacinth by a combination of ultrasonic-assisted alkaline pretreatment, dark fermentation, and microbial electrolysis cell. Biores Technol. 2022;357:127340. doi: 10.1016/j.biortech.2022.127340
- He K, Li W, Tang L, et al. Suppressing methane production to boost high-purity hydrogen production in microbial electrolysis cells. Environ Sci Technol. 2022;56(17):11931–11951. doi: 10.1021/acs.est.2c02371
- Askari A, Taherkhani M, Vahabzadeh F, et al. Bioelectrochemical treatment of olive oil mill wastewater using an optimized microbial electrolysis cell to produce hydrogen. Korean J Chem Eng. 2022;39(8):2148–2155. doi: 10.1007/s11814-022-1167-3
- Murugaiyan J, Narayanan A, Naina Mohamed S, et al. An overview of microbial electrolysis cell configuration: Challenges and prospects on biohydrogen production. Int J Energy Res. 2022;46(14):20811–20827. doi: 10.1002/er.8494
- Yang E, Omar Mohamed H, Park S-G, et al. A review on self-sustainable microbial electrolysis cells for electro-biohydrogen production via coupling with carbon-neutral renewable energy technologies. Biores Technol. 2021;320:124363. doi: 10.1016/j.biortech.2020.124363
- Jayabalan T, Matheswaran M, Naina Mohammed S, et al. Biohydrogen production from sugar industry effluents using nickel based electrode materials in microbial electrolysis cell. Int J Hydrogen Energy. 2019;44(32):17381–17388. doi: 10.1016/j.ijhydene.2018.09.219
- Qin M, Molitor H, Brazil B, et al. Recovery of nitrogen and water from landfill leachate by a microbial electrolysis cell–forward osmosis system. Biores Technol. 2016;200:485–492. doi: 10.1016/j.biortech.2015.10.066
- Samsudeen N, Spurgeon J, Matheswaran M, et al. Simultaneous biohydrogen production with distillery wastewater treatment using modified microbial electrolysis cell. Int J Hydrogen Energy. 2020;45(36):18266–18274. doi: 10.1016/j.ijhydene.2019.06.134
- Cardeña R, Moreno‐Andrade I, Buitrón G, et al. Improvement of the bioelectrochemical hydrogen production from food waste fermentation effluent using a novel start-up strategy. J Chem Technol Biot. 2018;93(3):878–886. doi: 10.1002/jctb.5443
- San-Martín MI, Sotres A, Alonso RM, et al. Assessing anodic microbial populations and membrane ageing in a pilot microbial electrolysis cell. Int J Hydrogen Energy. 2019;44(32):17304–17315. doi: 10.1016/j.ijhydene.2019.01.287
- Kim IS, Yang E, Choi M-J, et al. Bioelectrochemical production of hydrogen from organic waste. Prod Hydrogen Renewable Resour. 2015;249–281. Springer Netherlands. doi: 10.1007/978-94-017-7330-0_9
- Jia X, Li M, Wang Y, et al. Enhancement of hydrogen production and energy recovery through electro-fermentation from the dark fermentation effluent of food waste. Environ Sci Ecotechnol. 2020;1:100006. doi: 10.1016/j.ese.2019.100006
- Khongkliang P, Kongjan P, Utarapichat B, et al. Continuous hydrogen production from cassava starch processing wastewater by two-stage thermophilic dark fermentation and microbial electrolysis. Int J Hydrogen Energy. 2017;42(45):27584–27592. doi: 10.1016/j.ijhydene.2017.06.145
- Jung K-W, Kim D-H, Kim S-H, et al. Bioreactor design for continuous dark fermentative hydrogen production. Biores Technol. 2011;102(18):8612–8620. doi: 10.1016/j.biortech.2011.03.056
- Cheng S, Logan BE. Sustainable and efficient biohydrogen production via electrohydrogenesis. Proc Nat Acad Sci. 2007;104(47):18871–18873. doi: 10.1073/pnas.0706379104
- Escapa A, Lobato A, García DM, et al. Hydrogen production and COD elimination rate in a continuous microbial electrolysis cell: The influence of hydraulic retention time and applied voltage. Environ Prog Sustainable Energy. 2013;32(2):263–268. doi: 10.1002/ep.11619
- Yang N, Hafez H, Nakhla G, et al. Impact of volatile fatty acids on microbial electrolysis cell performance. Biores Technol. 2015;193:449–455. doi: 10.1016/j.biortech.2015.06.124
- Torres CI, Kato Marcus A, Rittmann BE. Kinetics of consumption of fermentation products by anode-respiring bacteria. Appl Microbiol Biotechnol. 2007;77(3):689–697. doi: 10.1007/s00253-007-1198-z
- Georg S, de Eguren Cordoba I, Sleutels T, et al. Competition of electrogens with methanogens for hydrogen in bioanodes. Water Res. 2020;170:115292. doi: 10.1016/j.watres.2019.115292
- Leicester D, Amezaga J, Heidrich E, et al. Is bioelectrochemical energy production from wastewater a reality? Identifying and standardising the progress made in scaling up microbial electrolysis cells. Renew Sust Energ Rev. 2020;133:110279. doi: 10.1016/j.rser.2020.110279
- Ziara RMM, Miller DN, Subbiah J, et al. Lactate wastewater dark fermentation: The effect of temperature and initial pH on biohydrogen production and microbial community. Int J Hydrogen Energy. 2019;44(2):661–673. doi: 10.1016/j.ijhydene.2018.11.045
- Rivera I, Buitrón G, Bakonyi P, et al. Hydrogen production in a microbial electrolysis cell fed with a dark fermentation effluent. J Appl Electrochem. 2015;45(11):1223–1229. doi: 10.1007/s10800-015-0864-6
- Paz-Mireles CL, Razo-Flores E, Trejo G, et al. Inhibitory effect of ethanol on the experimental electrical charge and hydrogen production in microbial electrolysis cells (MECs). J Electroanal Chem. 2019;835:106–113. doi: 10.1016/j.jelechem.2019.01.028
- Baek G, Shi L, Rossi R, et al. The effect of high applied voltages on bioanodes of microbial electrolysis cells in the presence of chlorides. Chem Eng J. 2021;405:126742. doi: 10.1016/j.cej.2020.126742
- Choi Y-J, Mohamed HO, Park S-G, et al. Electrophoretically fabricated nickel/nickel oxides as cost effective nanocatalysts for the oxygen reduction reaction in air-cathode microbial fuel cell. Int J Hydrogen Energy. 2020;45(10):5960–5970. doi: 10.1016/j.ijhydene.2019.05.091
- Song Y, Kim D-S, Woo J-H, et al. Effect of surface modification of anode with surfactant on the performance of microbial fuel cell. Int J Energy Res. 2015;39(6):860–868. doi: 10.1002/er.3284
- Li W, Zhu J, Lou Y, et al. MnO2/Tourmaline composites as efficient cathodic catalysts enhance bioelectroremediation of contaminated river sediment and shape biofilm microbiomes in sediment microbial fuel cells. Appl Catal B Environ. 2020;278:119331. doi: 10.1016/j.apcatb.2020.119331
- Zain S, Ching NL, Jusoh S, et al. Different types of microbial fuel cell (MFC) systems for simultaneous electricity generation and pollutant removal. J Teknologi. 2015;74(3):74(3. doi: 10.11113/jt.v74.4544
- Salman HH, Ismail ZZ. Bioelectrochemical treatment of sewage associated with desalination of wetland saline water and bioelectricity generation in stacked microbial fuel cell. 2020.
- Yuan H, Li J, Yuan C, et al. Facile synthesis of MoS2@CNT as an effective catalyst for hydrogen production in microbial electrolysis cells. ChemElectrochem. 2014;1(11):1828–1833. doi: 10.1002/celc.201402150
- Chookaew T, Prasertsan P, Ren ZJ, et al. Two-stage conversion of crude glycerol to energy using dark fermentation linked with microbial fuel cell or microbial electrolysis cell. New Biotechnology. 2014;31(2):179–184. doi: 10.1016/j.nbt.2013.12.004
- Wukasch RF. Proceedings of the 49th Industrial Waste Conference; 1994 May 9–11; Purdue University. Boca Raton (FL): Lewis Publishers; 1994.
- Dhar BR, Elbeshbishy E, Hafez H, et al. Hydrogen production from sugar beet juice using an integrated biohydrogen process of dark fermentation and microbial electrolysis cell. Biores Technol. 2015;198:223–230. doi: 10.1016/j.biortech.2015.08.048
- Ghasemi M, Wan Daud WR, Alam J, et al. Sulfonated poly ether ether ketone with different degree of sulphonation in microbial fuel cell: Application study and economical analysis. Int J Hydrogen Energy. 2016;41(8):4862–4871. doi: 10.1016/j.ijhydene.2015.10.029
- Feng Q, Song Y-C, Ahn Y, et al. Electroactive microorganisms in bulk solution contribute significantly to methane production in bioelectrochemical anaerobic reactor. Biores Technol. 2018;259:119–127. doi: 10.1016/j.biortech.2018.03.039
- Gele MY, Kachooei AS, Yaghmaei S, et al. Developing a self-powered microfluidic microbial electrolysis cell (MEC) for converting oxalate into hydrogen. J Environ Chem Eng. 2023;11(2):109373. doi: 10.1016/j.jece.2023.109373
- Cario BP, Rossi R, Kim K-Y, et al. Applying the electrode potential slope method as a tool to quantitatively evaluate the performance of individual microbial electrolysis cell components. Biores Technol. 2019;287:121418. doi: 10.1016/j.biortech.2019.121418
- Apostolopoulos I, Bampos G, Soto Beobide A, et al. The effect of anode material on the performance of a hydrogen producing microbial electrolysis cell, operating with synthetic and real wastewaters. Energies. 2021;14(24):8375. doi: 10.3390/en14248375
- Cheng KY, Kaksonen AH, Cord-Ruwisch R, et al. Ammonia recycling enables sustainable operation of bioelectrochemical systems. Biores Technol. 2013;143:25–31. doi: 10.1016/j.biortech.2013.05.108
- Guang L, Koomson DA, Jingyu H, et al. Performance of exoelectrogenic bacteria used in microbial desalination cell technology. Int J Environ Res Public Health. 2020;17(3):1121. doi: 10.3390/ijerph17031121
- Kyazze G, Popov A, Dinsdale R, et al. Influence of catholyte pH and temperature on hydrogen production from acetate using a two chamber concentric tubular microbial electrolysis cell. Int J Hydrogen Energy. 2010;35(15):7716–7722. doi: 10.1016/j.ijhydene.2010.05.036
- Ki D, Popat SC, Torres CI, et al. Reduced overpotentials in microbial electrolysis cells through improved design, operation, and electrochemical characterization. Chem Eng J. 2016;287:181–188. doi: 10.1016/j.cej.2015.11.022
- Liang D, Zhang L, He W, et al. Efficient hydrogen recovery with CoP-NF as cathode in microbial electrolysis cells. Appl Energy. 2020;264:114700. doi: 10.1016/j.apenergy.2020.114700
- Dhar BR, Lee H-S. Membranes for bioelectrochemical systems: challenges and research advances. Environ Technol. 2013;34(13–14):1751–1764. doi: 10.1080/09593330.2013.822007
- Jiang Y, Liang P, Zhang C, et al. Periodic polarity reversal for stabilizing the pH in two-chamber microbial electrolysis cells. Appl Energy. 2016;165:670–675. doi: 10.1016/j.apenergy.2016.01.001
- Zhao N, Liang D, Li X, et al. Hydrophilic porous materials provide efficient gas-liquid separation to advance hydrogen production in microbial electrolysis cells. Biores Technol. 2021;337:125352. doi: 10.1016/j.biortech.2021.125352
- Zhang Z, She L, Zhang J, et al. Electrochemical acidolysis of magnesite to induce struvite crystallization for recovering phosphorus from aqueous solution. Chemosphere. 2019;226:307–315. doi: 10.1016/j.chemosphere.2019.03.106
- Rozendal RA, Hamelers HVM, Buisman CJN. Effects of membrane cation transport on pH and microbial fuel cell performance. Environ Sci Technol. 2006;40(17):5206–5211. doi: 10.1021/es060387r
- Rossi R, Baek G, Logan BE. Vapor-fed cathode microbial electrolysis cells with closely spaced electrodes enables greatly improved performance. Environ Sci Technol. 2022;56(2):1211–1220. doi: 10.1021/acs.est.1c06769
- Yaoli Y, Logan BE. The importance of OH− transport through anion exchange membrane in microbial electrolysis cells. Int J Hydrogen Energy. 2018;43(5):2645–2653. doi: 10.1016/j.ijhydene.2017.12.074
- Zhen G, Lu X, Kumar G, et al. Microbial electrolysis cell platform for simultaneous waste biorefinery and clean electrofuels generation: Current situation, challenges and future perspectives. Prog Energ Combust Sci. 2017;63:119–145. doi: 10.1016/j.pecs.2017.07.003
- Sleutels THJA, Hamelers HVM, Rozendal RA, et al. Ion transport resistance in microbial electrolysis cells with anion and cation exchange membranes. Int J Hydrogen Energy. 2009;34(9):3612–3620. doi: 10.1016/j.ijhydene.2009.03.004
- Li X-H, Liang D-W, Bai Y-X, et al. Enhanced H2 production from corn stalk by integrating dark fermentation and single chamber microbial electrolysis cells with double anode arrangement. Int J Hydrogen Energy. 2014;39(17):8977–8982. doi: 10.1016/j.ijhydene.2014.03.065
- Hari AR, Katuri KP, Gorron E, et al. Multiple paths of electron flow to current in microbial electrolysis cells fed with low and high concentrations of propionate. Appl Microbiol Biotechnol. 2016;100(13):5999–6011. doi: 10.1007/s00253-016-7402-2
- Sun D, Call DF, Kiely PD, et al. Syntrophic interactions improve power production in formic acid fed MFCs operated with set anode potentials or fixed resistances. Biotechnol Bioeng. 2012;109(2):405–414. doi: 10.1002/bit.23348
- Hasibar B, Ergal İ, Abreu Dias S, et al. Competing acetate consumption and production inside a microbial electrolysis cell. J Environ Chem Eng. 2020;8(4):103847. doi: 10.1016/j.jece.2020.103847
- Amani T, Nosrati M, Sreekrishnan TR, et al. Anaerobic digestion from the viewpoint of microbiological, chemical, and operational aspects — a review. Environ Rev. 2010;18:255–278. doi: 10.1139/A10-011
- Robles A, Yellowman TL, Joshi S, et al. Microbial chain elongation and subsequent fermentation of elongated carboxylates as H2-producing processes for sustained reductive dechlorination of chlorinated ethenes. Environ Sci Technol. 2021;55(15):10398–10410. doi: 10.1021/acs.est.1c01319
- Khongkliang P, Jehlee A, Kongjan P, et al. High efficient biohydrogen production from palm oil mill effluent by two-stage dark fermentation and microbial electrolysis under thermophilic condition. Int J Hydrogen Energy. 2019;44(60):31841–31852. doi: 10.1016/j.ijhydene.2019.10.022
- Shao Q, Li J, Yang S, et al. Effects of different substrates on microbial electrolysis cell (MEC) anodic membrane: biodiversity and hydrogen production performance. Water Sci Technol. 2019;79(6):1123–1133. doi: 10.2166/wst.2019.107
- Zhang J, Chang H, Li X, et al. Boosting hydrogen production from fermentation effluent of biomass wastes in cylindrical single-chamber microbial electrolysis cell. Environ Sci Pollut Res. 2022;29(59):89727–89737. doi: 10.1007/s11356-022-22095-9
- Flayac J-C, Trably E, Bernet N, et al. Microbial ecology of anodic biofilms: from species selection to microbial interactions. Microbial Fuel Cell. 2018;63–85. Springer International Publishing. doi: 10.1007/978-3-319-66793-5_4
- Flayac J-C, Trably E, Bernet N. Microbial ecology of anodic biofilms: from species selection to microbial interactions. In: Das D, editor. Microbial Fuel Cell: A Bioelectrochem Syst Converts Waste to Watts. Springer, Cham; 2018. pp. 63–85. doi: 10.1007/978-3-319-66793-5_4
- Parameswaran P, Torres CI, Lee H-S, et al. Syntrophic interactions among anode respiring bacteria (ARB) and Non‐ARB in a biofilm anode: electron balances. Biotechnol Bioeng. 2009;103(3):513–523. doi: 10.1002/bit.22267
- Miceli JF, Garcia-Peña I, Parameswaran P, et al. Combining microbial cultures for efficient production of electricity from butyrate in a microbial electrochemical cell. Biores Technol. 2014;169:169–174. doi: 10.1016/j.biortech.2014.06.090
- Mahmoud M, Parameswaran P, Torres CI, et al. Fermentation pre-treatment of landfill leachate for enhanced electron recovery in a microbial electrolysis cell. Biores Technol. 2014;151:151–158. doi: 10.1016/j.biortech.2013.10.053
- Heidrich ES, Dolfing J, Scott K, et al. Production of hydrogen from domestic wastewater in a pilot-scale microbial electrolysis cell. Appl Microbiol Biotechnol. 2013;97(15):6979–6989. doi: 10.1007/s00253-012-4456-7
- Heidrich ES, Edwards SR, Dolfing J, et al. Performance of a pilot scale microbial electrolysis cell fed on domestic wastewater at ambient temperatures for a 12 month period. Biores Technol. 2014;173:87–95. doi: 10.1016/j.biortech.2014.09.083
- Cotterill SE, Dolfing J, Curtis TP, et al. Community assembly in wastewater-fed pilot-scale microbial electrolysis cells. Front Energy Res. 2018;6:98. doi: 10.3389/fenrg.2018.00098
- Li W-W, Sheng GP, Liu XW, et al. Recent advances in the separators for microbial fuel cells. Bioresource Technology query. 2011;102(1):244–252. doi: 10.1016/j.biortech.2010.03.090