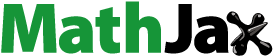
ABSTRACT
Bioethanol is a renewable fuel widely used in road transportation and is generally regarded as a clean energy source. Although fermentation is one of the major processes in bioethanol production, studies on improving its efficiency through operational design are limited, especially compared to other steps (pretreatment and hydrolysis/saccharification). In this study, two adapted feeding strategies, in which feed medium addition (sugar delivery) was adjusted to increase the supply of fermentable sugar, were developed to improve ethanol productivity in 5-L fed-batch fermentation by Saccharomyces cerevisiae. Specifically, a linear adapted feeding strategy was established based on changes in cell biomass, and an exponential adapted feeding strategy was developed based on cell biomass accumulation. By implementing these two feeding strategies, the overall ethanol productivity reached 0.880.04 and 0.87
0.06 g/L/h, respectively. This corresponded to ~20% increases in ethanol productivity compared to fixed pulsed feeding operations. Additionally, there was no residual glucose at the end of fermentation, and final ethanol content reached 95
3 g/L under the linear adapted operation and 104
3 g/L under the exponential adapted feeding strategy. No statistical difference was observed in the overall ethanol yield (ethanol-to-sugar ratio) between fixed and adapted feeding strategies (~91%). These results demonstrate that sugar delivery controlled by adapted feeding strategies was more efficient than fixed feeding operations, leading to higher ethanol productivity. Overall, this study provides novel adapted feeding strategies to improve sugar delivery and ethanol productivity. Integration into the current practices of the ethanol industry could improve productivity and reduce production costs of fermentation processes.
1. Introduction
According to the International Energy Agency (IEA), global energy demand is expected to increase by 9% between 2019 and 2030. This and net-zero emissions targets for 2050 have led many countries to aim for bioenergy to become a primary energy source, increasing its demand by 60% [Citation1]. Bioethanol, a renewable liquid biofuel, has become one of the main renewable long-term energy sources. It can be blended with gasoline for road transportation, leading to increases in octane rating compared to pure gasoline [Citation2] and reductions in carbon dioxide (CO2), carbon monoxide (CO), and nitrate oxide (NOx) emissions [Citation3]. US, Brazil, some European countries, China, India, and Canada are major players in bioethanol production [Citation4], of which fermentation is an essential step. Common feedstocks for first-generation bioethanol include sugar-based and starch-based biomass, such as sugarcane and corn [Citation5]. For sugar-based feedstocks, sugars are directly converted into ethanol by microorganisms via ethanol fermentation. On the other hand, starchy feedstocks are processed by simultaneous saccharification and fermentation (SSF) which combines enzymatic hydrolysis with fermentation in a single step [Citation6]. First-generation bioethanol has been plagued by the food-fuel debate, and it should be pointed out that most of the bioethanol currently produced still comes from edible feedstocks [Citation5,Citation7].
To improve the sustainability of bioethanol production, it is imperative to explore alternative feedstocks. Lignocellulosic biomass, an abundant renewable material, is a promising feedstock for long-term bioethanol production as it does not compete with food crops in land utilization [Citation8]. Lignocellulose is mainly composed of cellulose, hemicellulose and lignin. Cellulose and hemicellulose are formed by C6 and C6/C5 sugar units, respectively, that can be converted to ethanol through fermentation. However, interlocking structures of cellulose, hemicellulose and lignin make lignocellulose recalcitrant to decomposition [Citation9]. Although the use of SSF for lignocellulosic ethanol production has been studied [Citation10–19] (), its implementation is difficult and limited and, consequently, it faces significant challenges to reach production at an industrial scale. Despite various lignocellulosic feedstocks being investigated for ethanol production, the resulting ethanol concentration and productivity are underperforming sugar-based ethanol production (with ethanol productivity reaching 5–8 g/L/h [Citation10]). Separate hydrolysis and fermentation (SHF) can optimize these processing steps separately and is thus seen as a valuable short-term option to improve lignocellulosic ethanol production at an industrial scale. In fact, several companies, such as ACE ethanol LLC, Element LLC, and POET Biorefining, have demonstrated this at industrial scale. Instead of building a new infrastructure for cellulosic ethanol production, integrating cellulosic biomass into first-generation bioethanol plants is more feasible for the ethanol industry.
Table 1. Lignocellulosic ethanol production via simultaneous saccharification and fermentation using Saccharomyces cerevisiae.
To enhance the prospects of lignocellulosic ethanol production at an industrial scale, more efficient fermentation processes need to be developed. As ethanol productivity is greatest during exponential growth of Saccharomyces cerevisiae [Citation20], the most commonly used yeast for bioethanol production, there are incentives to develop processing strategies that extend this phase of growth, such as fed-batch fermentation [Citation21]. This approach consists of continuously or intermittently feeding essential nutrients to the fermenter in order to control and sustain cell growth and product formation [Citation22]. Fed-batch operation also helps overcoming substrate inhibition during the fermentation process and can lead to greater ethanol yield (ethanol-to-sugar ratio) [Citation23] and ethanol productivity (g/L/h) [Citation24], compared to batch fermentation. Interestingly, the above two studies have shown that feeding strategies play an important role in fermentation performance for ethanol production. This highlights the importance of developing effective feeding strategies for fed-batch fermentation. Once an efficient fermentation process is designed, bench-scale assessments can bring practical knowledge in transitioning the process from laboratory experiments to commercial production [Citation25]. For example, Kang et al. [Citation26] performed economic and sensitivity analyses based on a bench-scale plant for lignocellulosic ethanol production, providing feasible viewpoints to the development of a commercial production plant. Reproducibility of ethanol production by yeast between laboratory- and bench-scale is also crucial to understanding possible hindrances to industrial ethanol production [Citation27]. Nonetheless, most studies on the improvement of lignocellulosic ethanol production have been carried out at laboratory-scale. It is thus worth further investigating the efficient bioethanol fermentation processes at bench-scale to enhance the prospect of commercialization.
Overall, fed-batch fermentation has the potential to greatly improve bioethanol production. The feeding strategy is a key factor in fed-batch fermentation; it can be designed based on nutrient contents, cell growth, or other factors impacting metabolism and the final product [Citation28–30]. However, there are few studies on the role of operational design in improving ethanol productivity through fed-batch fermentation. The development of adapted feeding strategies enables better management of dynamic fermentation processes and improves both substrate utilization of the yeast and ethanol productivity. In this context, the present work aimed to develop adapted feeding strategies for sugar delivery to enhance ethanol production in cultures of S. cerevisiae at bench-scale. It compares the performance of a fixed and two adapted pulsing fed-batch strategies in terms of ethanol titer, yield, substrate utilization and ethanol productivity. All fermentations were performed in 5-L stirred tank fermenters with monitoring of multiple parameters related to ethanol fermentation, such as evolved gas flow rate, pH, dissolved oxygen, and agitation speed. This work highlights the potential of these feeding strategies for the improvement of the performance of industrial bioethanol production from first- and second-generation feedstocks.
2. Methods and materials
2.1. Yeast, medium, and cultivation
SuperstartTM active distillers dry yeast, Saccharomyces cerevisiae, was purchased from Lallemand Ethanol Technology (Milwaukee, WI, USA) and was used for ethanol fermentation in this study. Methods of yeast cultivation and preparation of glycerol stock followed Wang et al. [Citation31]. Briefly, a glycerol stock was thawed and streaked on a YPD agar (YPDA) plate. YPDA was made from 50 g/L yeast-peptone-dextrose medium (BD DifcoTM, Bergen Country, NJ, USA) and 15 g/L agar (Thermo Fisher Scientific, Waltham, MA, USA), and was sterilized by autoclaving (121°C/15 min). After culturing the yeast on YPDA at 30°C for 2–3 days, an isolated colony was selected, transferred to 20 mL of yeast nitrogen base (YNB) medium (50-mL shake flask), and cultured at 30°C with shaking at 230 rpm for 18–20 h to produce the first seed culture. YNB medium, prepared from 50 g/L glucose, 6.7 g/L YNB with amino acids (MilliporeSigma, Burlington, MA, USA) in 0.1 M sodium phosphate buffer (NaH2PO42 H2O/Na2HPO4
2 H2O, pH 6.0, Thermo Fisher Scientific), was sterilized by filtration (0.2 μm SartolabTM P20 Pressure Filters, Sartorius, Göttingen, Germany). The first seed culture was transferred into 200 mL of fresh YNB medium (500-mL shake flask) to reach an initial optical density at 600 nm (OD600) ~0.2, resulting in the second seed culture. It was then incubated at 30°C with shaking at 250 rpm until OD600 reached ~0.5. At this point, the active cell culture was ready to be inoculated into the fermenter.
2.2. Fermentation
2.2.1. Fermenter settings
All fermentation experiments were performed in 5-L stirred tank fermenters (Infors-HT, Bottmingen, Switzerland) based on the settings from Wang et al. [Citation32]. Dissolved oxygen probe (VisiFerm DO ECS 325 mm H0, Hamilton Company, Reno, NV, USA) and pH probe (EasyFerm Plus PHI K8 325 mm, Hamilton Company) were attached to the fermenter. 2 N NaOH (Thermo Fisher Scientific) was used for pH control (set point at pH > 4.0). Pall AcroTM 50 vent filters (Pall Canada ULC., Mississauga, ON, Canada) were connected to the gas inlet and outlet of the fermenter. A water trap was installed between the gas outlet and the mass flow meter (MW-200SCCM-D/5 M, Alicat Scientific Inc., Tucson, AZ, USA). The water trap was used to prevent environmental air from being introduced to the fermenter and maintain anaerobic conditions for ethanol fermentation. The flow meter was calibrated with pure CO2 by the supplier, and it was used to monitor the flow of gas released from the fermenter. Data from the gas flow meter was reported at standard atmospheric conditions (25°C, 1 atm) and the flow rate was reported as the average value over a 15-min timespan. An external condenser, set at 0°C, was used as the cooling system for the fermenter and vented gas. The bioreactor system was controlled by the software Iris 6.0 (Infors-HT). The initial working volume of all fed-batch operation was 2 L.
2.2.2. Fed-batch fermentation
The YNB fermentation medium was prepared, filter-sterilized (as mentioned above) and transferred to the fermenter aseptically. Before inoculation, the medium was flushed with N2 (99.998% purity, Praxair Canada Inc., Mississauga, ON, Canada) for 30 min through the bioreactor gas inlet/sparger. Yeast grown to OD600 ~0.5 in the second seed culture was added to the fermenter (8% (v/v) of the fermentation medium) as inoculum. Following inoculation, N2 was purged into the fermenter for 10 min to ensure anaerobic conditions. Fermentation was conducted at 30°C and 600 rpm using two Rushton impellers. The initial stage of fermentation acted as batch fermentation.
The outlet gas flow rate was used to monitor ethanol fermentation in real-time, as per Wang et al. [Citation32], and served as an indicator to trigger pulse feeding. When the gas flow rate approached zero, a pulse of fresh feed medium was fed to the fermenter to increase the sugar concentration to a given value, depending on the experiment. The feed medium was a modified YNB medium: 500 g/L glucose and 6.7 g/L YNB with amino acids in 0.1 M sodium phosphate buffer (pH 6.0). The fermentation broth was sampled throughout experiments for biological and chemical analyses described below.
2.2.3. Feeding strategies
Pulsed feeding was conducted in all fed-batch fermentations. Adapted feeding strategies were developed to improve sugar delivery and ethanol productivity in fed-batch fermentation, while fixed feeding strategies were used for comparison. We hypothesized that the adjustment of glucose addition based on the change in cell biomass by pulsed feeding operations would enhance substrate utilization and ethanol productivity, compared to fixed glucose additions. Overall, three strategies were developed.
In a fixed feeding operation, the fresh feed medium was fed to increase sugar concentration to either 50 or 100 g/L (described as 5% pulsed feeding and 10% pulsed feeding, respectively).
In linear adapted feeding operation, the first pulsed feed increased glucose concentration to 5% (50 g/L), while the subsequent pulsed feeds were adjusted based on the change of OD600, according to equation 1:
Eq. (1)
Eq. (1)
where Cf is glucose concentration (%, w/v) to reach in the fermenter, ODt is the OD600 reading at a given sampling time t, and ODt-1 is the OD600 reading at the previous sampling time.
3) In exponential adapted feeding operation, the pulsed feeding was adapted based on cell biomass changes and cell accumulation. The glucose addition was based on equation 2:
Equation 2 ensures the glucose concentration in the fermenter never exceeds 10%, generally regarded as the maximum concentration to avoid inhibiting yeast growth and ethanol production [Citation33,Citation34]. For all feeding strategies, the required fresh feed volume was calculated based on the volume in the fermenter and the glucose concentration in the fresh feed medium (500 g/L). In all cases, the final volume was found to range between 2.3 and 2.5 L.
2.3. Analytical methods
OD600 was measured for 1-ml samples using a UV–Vis spectrophotometer (Ultrospec 4300 Pro, Amersham Biosciences, Mississauga, ON, Canada). Cell dry weight (CDW) analysis was performed as follows. A 9-ml sample was filtered using a pre-weighed 0.45-μm filter paper (WhatmanTM type WCN cellulose nitrate membranes, Cytiva, Maidstone, Kent, UK). The retentate was washed by filtering through an equal volume of 10 mM sterile sodium phosphate buffer. The retentate and filter paper were then removed and dried at 60°C in an oven (Fisher Scientific Isotemp oven model 750F, Dubuque, IA, USA) until the mass was constant. The CDW was calculated and reported in g/L. During fed-batch fermentation, OD600 and CDW were used to evaluate yeast growth. Supplementary Figure 1 shows the strong correlation between these two parameters (R2 = 0.995).
Glucose and ethanol concentrations were determined by high-performance liquid chromatography (HPLC) and gas chromatography (GC), respectively [Citation35]. Briefly, glucose analysis was carried out on the Agilent 1200 series HPLC system (Agilent, Santa Clara, CA, USA) with an Aminex HPX-87 H column (3007.8 mm; Bio-Rad Laboratory, Hercules, CA, USA) held at 60°C and a refractive index detector. A 5 mM sulfuric acid solution was used as the mobile phase with a constant flow rate of 0.5 mL/min. Ethanol analysis was conducted in the Agilent 7890A series GC system (Agilent Technologies, Mississauga, ON, Canada) equipped with the Agilent 7693 series autosampler (injector temperature: 170°C; pressure: 7.5 psi; septum purge flow: 3 mL/min; split ratio: 10 to 1), RestekTM Stabilwax-DA column (30 m
0.53 mm
0.5 μm; Restek, Bellefonte, PA, USA) and a flame ionization detector (temperature: 190°C; airflow: 400 mL/min; hydrogen flow: 40 mL/min; make-up nitrogen flow: 25 mL/min). The following method was applied to the oven for ethanol analysis: temperature was kept at 35°C for 3 min, then increase by 20°C/min up to 190°C, which was held for 1 min. Helium was used as the carrier gas (constant pressure: 51.710 kPa), and 1% (v/v) 1-butanol was added as the internal standard in the sample.
2.4. Statistical analysis
All fermentation experiments were run in independent triplicates. Data were expressed as means ± standard deviation. Statistical analysis was performed by one-way analysis of variance (ANOVA) and Tukey’s honestly significant difference test, where p < 0.05 indicated the significant differences.
3. Results and discussion
3.1. Impact of adapted feeding strategies
Pulsed feeding is a general mode of operation commonly used in fed-batch fermentation, as it is simple and easy to control [Citation36,Citation37]. Optimizing feeding strategies can improve productivity which helps reduce production time and cost [Citation22] and increase cell biomass and ethanol concentration during fermentation [Citation38]. To improve sugar utilization and ethanol production in fed-batch fermentation, linear adapted and exponential adapted pulsed feeding strategies were developed and compared to fixed pulsed feeding strategies.
shows cell dry weight (CDW) for each strategy tested. CDW was greater in the adapted feeding strategies than in the fixed feeding strategies during fermentation. For example, CDW was ~2 g/L at the end of initial batches (at approximately 20 h of operation for each experiment); it remained relatively constant in the fixed pulsed feeding experiments. However, it reached ~4 g/L by the end of fermentations undergoing linear adapted feeding. Similar results were observed when using the exponential adapted feeding strategy. This suggests that adapted feeding strategies benefit cell growth during ethanol fed-batch fermentation compared to fixed feeding strategies. It has been shown that cell biomass concentration can be enhanced by increasing glucose addition [Citation23]; and this was observed in our two adapted feeding strategies in which glucose supplementation was gradually increased based on cell content and accumulation. On the other hand, Phukoetphim et al. [Citation24] reported that viable cell count did not increase following pulsed feedings, which might be due to high glucose addition causing osmotic pressure on the yeast. In this study, we limited the glucose addition to 10% (w/v) to reduce the effect of substrate inhibition on the yeast; as a result, cell growth greatly improved in both adapted pulsed feeding strategies. In addition, it should be noted that flocculation was observed during all fermentations carried out in this study. Indeed, flocculation is a common characteristic of many S. cerevisiae cultures, and is affected by pH, temperature, nutrients composition and other fermentation parameters [Citation39]. In our experiments, ethanol accumulation and pulsed feeding (rapid, punctual addition of sugars) might have contributed to flocculation. Yeast flocculation can offer a cost-effective way to separate yeast from the liquid fraction at the end of fermentation; a widely applied strategy in the brewery industry [Citation40]. It is worth exploring flocculation in fuel ethanol production as it may benefit the downstream process for ethanol recovery.
Figure 1. Changes in cell dry weight (a-d), glucose content (e-h), ethanol titer (i-l), and evolved gas flow rate (m-p) from 5% fixed (circle), 10% fixed (triangle), linear adapted (square), and exponential adapted (diamond) feeding strategies.

Changes in glucose () and ethanol () concentrations during 5% fixed, 10% fixed, linear adapted, and exponential adapted feeding strategies are shown in . Firstly, it should be noted that there was no residual glucose at the end of the fermentation, regardless of the fed-batch strategy used, indicating complete utilization of the glucose supplied. Although fixed pulsed feeding operations were easy to implement, it had the poorest performance of the methods tested. A faster glucose consumption rate was observed with the linear adapted and exponential adapted feeding strategies (1.9 g/L/h), compared to the fixed feeding groups (1.5–1.6 g/L/h) (). In all cases, the glucose concentration was kept below 10% (w/v) to avoid metabolic inhibition of yeast growth [Citation34,Citation41]. This implies that the differences in glucose consumption rates observed between the adapted and fixed pulses could be attributed to better substrate management by the yeast. Glucose concentration can play a critical role in the growth rate of S. cerevisiae [Citation42]. When substrate concentration is higher (but below the limit of 10% glucose), the cell growth rate is expected to be faster, as observed in . Similar phenomena were observed by Chang et al. [Citation38], where the specific growth rate of S. cerevisiae increased when glucose input was increased from 10 to 100 g/L, but the cell growth rate started decreasing when glucose exceeded 100 g/L. High sugar concentration can cause extra osmotic stress on yeast which may damage cellular structure and negatively impact fermentation performance [Citation43]. Our study also indicates that sugar delivery by pulsed feeding can influence the performance of ethanol fermentation in the fed-batch mode. With the same amount of total glucose consumed, the glucose consumption rate was enhanced when using the linear adapted feeding strategy compared to the fixed feeding strategies (). In addition, applying pulsed feeding can mitigate the effects of substrate inhibition and further improve ethanol productivity compared to batch fermentations [Citation38]. It is worth noting that as the target substrate concentration increases in the fed-batch fermentation, there may be a trade-off between high final ethanol concentration and high ethanol productivity [Citation44]. Out of the feeding strategies tested, exponential adapted feeding strategy was able to enhance both final ethanol concentration and productivity, compared to the fixed feeding strategies.
Table 2. Overall performance in ethanol fermentation by four feeding strategies.
As seen in , ethanol accumulated between each pulsed feeding for all modes of operations. The final ethanol titers reached 97, 97, 98 and 103 g/L for the 5% fixed, 10% fixed, linear adapted, and exponential adapted pulsed feeding strategies, respectively. In other words, between 9–10% (w/v) ethanol was achieved at the end of fermentation. In industrial operation, increasing final ethanol concentration can reduce the cost of ethanol recovery in the downstream process. The minimal concentration of ethanol for an economic recovery in industrial settings is 4% (w/v) [Citation45,Citation46]. Our work demonstrates that 9–10% (w/v) ethanol production is achievable in 5-L fermenters, increasing the probability of applying adapted pulsed feeding strategies at a larger scale. As an example, Fujii et al. [Citation47] expanded the bioethanol production from a laboratory scale to a bench-scale and achieved higher sugar concentration from saccharification and higher ethanol concentration in fermentation with the bench-scale settings. Similar benefits from scaling up the bioprocess were found when ethanol yield was improved by 11.77% through scale-up from working with 150-mL serum bottles to 5-L bioreactors [Citation48].
On the other hand, high ethanol accumulation might induce inhibitory effects on the yeast during fermentation. After the last feeding, as ethanol concentration exceeded 9% (w/v), the ethanol production rate decreased compared to previous feeding intervals (). Ethanol can influence the structure of membranes in cells, such as nuclear and mitochondrial membranes, and other cell organelles, and further impact yeast metabolism, like fatty acid synthesis [Citation49,Citation50]. Hence, when it comes to optimizing the fermentation process for bioethanol production, it is important to consider the ethanol tolerance of a strain [Citation51,Citation52]. Overall, based on the trends shown in , the two adapted feeding strategies improved glucose utilization and ethanol production, compared to the fixed feeding modes. Ethanol productivity was enhanced by 23–24% when applying the linear adapted and exponential adapted approaches, respectively, compared to the 10% fixed strategy.
The overall performance of fixed and adapted feeding strategies in ethanol fermentation is shown in . Ethanol productivity improved by approximately 20% in both linear and exponential adapted feeding strategies, compared to fixed feeding strategies. This demonstrates that adjusting substrate feedings based on cell biomass concentration can benefit both substrate utilization and ethanol production, and the improvements in ethanol productivity support our hypothesis. To deal with dynamic metabolic changes of the yeast and optimize the metabolite productivity in fed-batch fermentation, an adapted feeding strategy can be a more promising operation than a fixed feeding mode. This concept is consistent with the report of Alfenore et al. [Citation53] who developed an exponential feeding strategy for vitamin supplement in ethanol fermentation, which improved the viability of S. cerevisiae and ethanol productivity by 10–18%. Maximizing the product formation rate can benefit the entire process design by, for example, reducing the required bioreactor size which reduces the cost of equipment and its maintenance [Citation22] or facilitating separation. In this case, fed-batch fermentation can be an effective tool to boost ethanol productivity and titer, which is supported by Chang et al. [Citation38] and Narisetty et al. [Citation54]. In our work, two adapted feeding strategies enhancing ethanol productivity compared to fixed feeding modes were successfully developed.
In addition to ethanol productivity, ethanol yield (based on the maximum theoretical ethanol-to-sugar ratio) and final ethanol concentration are crucial metrics of the performance of ethanol fermentation. Firstly, as shown in , ethanol yield reached between 89% and 92% for the four feeding strategies tested, with no statistical difference. In ethanol fermentation, the maximum theoretical yield of ethanol is 0.511 g per g glucose. In this study, fed-batch operations with 10% (w/v) glucose improved the ethanol yield by 12.5% compared to the batch stage. Using pulsed fed-batch to extend the active growth phase of cultures enabled the extended conversion of glucose to ethanol and carbon dioxide [Citation20], leading to improvements in ethanol yield () and increases in evolved gas flow rate (). Similar results were observed by Laopaiboon et al. [Citation55], who found that ethanol yield improved by 14% in fed-batch fermentation with pulsed feeding operations, compared to batch fermentation, due to reduced byproduct formation. Secondly, in terms of ethanol content, the highest ethanol production was observed under the exponential adapted feeding (). By considering cell accumulation during fermentation, the exponential adapted feeding can provide more substrate for ethanol fermentation and achieve higher ethanol concentration at the end of fermentation. Dilution effects from feeding operation translated into a final ethanol content slightly lower than the overall ethanol production. However, all four feeding strategies led to final ethanol contents of around 9–10% (w/v). Fed-batch operations can regulate various feeding policies to relieve the potential substrate inhibition toward the microorganism and further enhance final ethanol concentration and yield, as compared to the batch [Citation38]. Based on these advantages, it would be promising to combine fed-batch operation with other fermentation modes, such as extractive fermentation, as such integrated fermentation systems could enable the reduction of both substrate and product inhibition effects [Citation56]. This is of importance as increasing ethanol concentration in the fermentation medium can play a significant role in the cost and energy spent on the downstream process [Citation46].
Optimizing ethanol productivity, yield, and final concentration is a trade-off for the bioethanol industry. Different decision points can result in vastly different approaches to process design. The economic balance between operational strategy and ethanol production needs to be considered [Citation57]. For instance, in a study evaluating different pulsed feeding strategies for ethanol production from sweet sorghum juice, Laopaiboon et al. [Citation55] determined that one of the strategies required less inoculum, potentially leading to reduced operational cost. Additionally, the economic balance can be better evaluated through scaling up of the bioprocess; for example, scaling up to the bench-scale enables the provision of operational [Citation47] and economic [Citation26] information from advanced bioethanol production, which may offer further insights into the potential commercial phase. In our current study, although exponential adapted feeding required more glucose, it showed higher final ethanol content than the linear adapted feeding strategy (). Importantly, both adapted feeding strategies could be implemented in the 5-L fermenters. Overall, we developed two adapted feeding strategies to improve ethanol production, which helps reduce the production costs associated with fermentation. The concept of adapted feeding strategies can be integrated into current ethanol production; for example, it can be applied to either sugar-based or lignocellulosic ethanol production. The bench-scale performance demonstrated in this work is promising for future pilot-scale processes. The work can thus benefit the economic viability of commercial bioethanol.
It is worth noting that although sugar-based and starch-based feedstocks are the main sources for current bioethanol production in the industry, many companies, such as GranBio, POET Biorefining, and Raízen, are working on cellulosic ethanol as well. To produce cellulosic ethanol at an industrial scale, a single SSF is currently difficult to apply; one of the restrictions is the limited fermentation performance, as shown in . On the other hand, separate hydrolysis and fermentation (SHF) has been used to degrade the complex structure of cellulosic materials and produce ethanol separately, aiming to optimize the individual steps [Citation51]. Further enhancement of the production efficiency of SHF requires overcoming some of the limitations of current fermentation practices. Based on the results shown in this study, two adapted feeding strategies have the potential to be applied to direct fermentation and SHF for boosting sugar-based ethanol and lignocellulosic ethanol production in the short term.
It is critical to design an efficient feeding strategy for ethanol fermentation. For instance, Phukoetphim et al. [Citation24] conducted batch and fed-batch fermentations for ethanol production from sweet sorghum juice in high gravity conditions, and their work indicated that the continuous feeding strategies could improve ethanol productivity compared to the batch, while the pulsed feeding strategies did not yield enhancements. The fermentation performance of the pulsed feeding strategies might be limited by periodical high sugar concentrations compared to continuous feeding, which could be caused by extra osmotic stress to the yeast in the former. Besides, feeding or controlling actions in fed-batch fermentation may impact the heterogeneity of fermentation media when it comes to scaling up the bioprocess to industrial scale [Citation58]. To further explore the feasibility of the adapted pulsed feeding strategies, studies should be conducted in larger-scale bioreactors. However, it is clear that continuously adapted feeding is a promising alternative that enables the mitigation of substrate inhibition and fermentation heterogeneity during feeding, potentially leading to greater ethanol production performance.
3.2. Monitoring of evolved gas during fed-batch fermentation
Appropriate monitoring of ethanol fermentation is important to optimize production, regulate dynamic changes during fermentation and reduce production costs [Citation59]. For example, spectroscopy has been studied for monitoring glucose and ethanol contents in the fermentation medium. However, specific concerns need to be taken into account for this technique, such as the applicability of the calibration model for the independent process [Citation60]. Additionally, instrument fluctuations may contribute to response errors when building the calibration model [Citation61]. Considering fed-batch fermentation introduces fresh substrate to bioreactors intermittently or continuously, spectroscopy may not be the most effective option to monitor real-time changes during fermentation. Alternative monitoring tools need to be explored for fed-batch fermentation.
Wang et al. [Citation32] showed that evolved gas flow rate could be used as a real-time monitoring parameter for ethanol fermentation in a self-cycling fermentation system. It was pointed out that this parameter could be used to monitor metabolic activity and determine the divide between the exponential phase and stationary phase of the yeast [Citation32]. In the presented study, we monitored the evolved gas flow rate and applied it to determine the appropriate feeding points for the different fed-batch operations. Trends in evolved gas flow rate during 5% fixed, 10% fixed, linear adapted, and exponential adapted feeding operations are shown in . When evolved gas flow rate decreased sharply and reached values close to zero – indicating a rapid decline in metabolic activity, fresh feed medium was added to the bioreactor to extend substrate availability. After each feeding, the gas flow rate increased strongly, indicative of the reestablishment of rapid metabolic activity. For example, greater evolved gas flow rates were observed after 10% fixed pulses compared to 5% fixed pulses, consistent with greater specific growth rate expected at higher substrate concentration.
When investigating the effects of the adapted feeding strategies on the evolved gas flow rate, we could see that this parameter increased rapidly after the pulse and reached greater values than for the fixed feeding strategies (with the exponential adapted operation reaching as much as 34 ccm). This is interesting as it indicates that the rate of conversion of substrate to gas was greater in the adapted strategies even if less glucose was fed than in the 10% fixed pulsed strategy. This suggests a more rapid and efficient utilization of glucose, and in turn faster production of ethanol and carbon dioxide. In the 10% fixed pulsed strategy, the substrate addition may push the influence of substrate inhibition on the yeast metabolic activity. This surmise can echo the report from Chang et al. [Citation38] which showed that the growth rate of the yeast starts decreasing when glucose concentration exceeds 100 g/L. For this reason, it is critical to consider the substrate inhibition in ethanol fermentation to prevent loss of product yield [Citation62]. The present study shows that adapted feeding strategies can further improve ethanol productivity through tailored sugar delivery ().
For all feeding strategies tested, the magnitude of the evolved gas flow rate decreased as the number of pulse feedings increased, and the curves showed that it took a long time to utilize the substrate (). This is likely due to the accumulation of ethanol in the fermentation broth. After the last feeding, ethanol contents increased from 74 to 97 g/L, from 50 to 97 g/L, from 61 to 98 g/L, and from 63 to 103 g/L in the 5% fixed pulsed, 10% fixed pulsed, linear adapted, and exponential adapted operations, respectively (). It is worth noting that pH was controlled to prevent it from decreasing below 4.0 during fermentation, which likely helped avoid metabolic inhibition caused by low pH. Considering that ethanol concentration reached 90–100 g/L, it is reasonable to expect that, by this point, most cells were not fully activated for ethanol production, especially compared to the earlier pulsed feedings. Zhang et al. [Citation63] reported that when the initial exogenous ethanol concentration exceeded 70 g/L, the yeast ceased growing and producing ethanol, even if sufficient substrate was present. We were able to push ethanol production toward the boundary of high ethanol accumulation through pulsed feeding operations. Under ethanol stress, the physiology and gene expression of yeast can change to balance cellular energy expenditure, resulting in cell growth inhibition [Citation50]. This aligns with the reduced evolved gas flow rate following the last feedings (). Overall, the evolved gas flow rate proved to be a valuable real-time monitoring parameter for the determination of pulsed feeding and the evaluation of ethanol production and potency of feeding strategies.
4. Conclusions
In this study, two adapted feeding strategies developed for fed-batch fermentation led to significant enhancements in ethanol productivity (increases of approximately 20%) compared to fixed pulsed feeding strategies. Evolved gas flow rate, a result of sugar utilization, from ethanol fermentation could be viewed as a determining factor for pulsed feeding operations in ethanol production by S. cerevisiae. In addition, ethanol yields of 91% and titers of 13% (v/v) were achieved by using these adapted feeding strategies. The results demonstrated the advantages of adjusting feeding based on cell biomass during fed-batch fermentation. This approach, which was successfully established in 5-L fermenters, improved the efficiency of ethanol production. To sum up, linear adapted and exponential adapted pulsed feeding strategies show great potential for integration into sugar-based ethanol and lignocellulosic ethanol production to enhance productivity. Further, in-depth investigation of continuous, adapted feeding strategies at bench-scale, which minimizes fermentation heterogeneity during feedings, could further improve both sugar utilization and ethanol production. Such strategies will then serve as stepping stones in improving productivity of large-scale, industry operation.
Author’s contributions
DCB, DS, MC, and Y-HRH conceived and designed experiments. Y-HRH performed experiments and analyzed data. Y-HRH and DS wrote the manuscript. DCB, DS and MC provided assistance in data analysis and troubleshooting, and edited the manuscript. All authors have read and agreed to the published version of the manuscript.
Supplemental Material
Download MS Word (29 KB)Acknowledgments
We would like to acknowledge Alicat Scientific Inc. for their technical support on the mass flow meter (MW-200SCCM-D/5 M).
Disclosure statement
No potential conflict of interest was reported by the author(s).
Data availability statement
The authors confirm that the data supporting the findings of this study are available within the article and/or its supplementary materials.
Supplementary material
Supplemental data for this article can be accessed online at https://doi.org/10.1080/21655979.2023.2250950.
Additional information
Funding
References
- IEA [Internet]. World energy outlook 2020. Paris: International Energy Agency; 2020. [cited January 11, 2022]. Available from: https://www.iea.org/reports/world-energy-outlook-2020, License: CC BY 4.0
- Ruan R, Zhang Y, Chen P, et al. Chapter 1-Biofuels: introduction. In: Pandey A, Larroche C, and Dussap C, editors. Biofuels: alternative feedstocks and conversion processes for the production of liquid and gaseous biofuels. London, United Kingdom: Academic Press; 2019. p. 3–13.
- Aditiya H, Mahlia TMI, Chong W, et al. Second generation bioethanol production: a critical review. Renew Sustain Energ Rev. 2016;66:631–653. doi: 10.1016/j.rser.2016.07.015
- Zabed H, Sahu J, Suely A, et al. Bioethanol production from renewable sources: current perspectives and technological progress. Renew Sustain Energ Rev. 2017;71:475–501. doi: 10.1016/j.rser.2016.12.076
- Dalena F, Senatore A, Iulianelli A, et al. Ethanol from biomass: future and perspectives. Ethanol: Elsevier; 2019. p. 25–59.
- Karp SG, Medina JD, Letti LA, et al. Bioeconomy and biofuels: the case of sugarcane ethanol in Brazil. Biofuels, Bioprod Bioref. 2021;15(3):899–912. doi: 10.1002/bbb.2195
- Rudel TK. Food versus fuel: extractive industries, insecure land tenure, and gaps in world food production. World Dev. 2013;51:62–70. doi: 10.1016/j.worlddev.2013.05.015
- Dos Santos LV, de Barros Grassi MC, Gallardo JCM, et al. Second-generation ethanol: the need is becoming a reality. Ind Biotechnol. 2016;12(1):40–57. doi: 10.1089/ind.2015.0017
- Toor M, Kumar SS, Malyan SK, et al. An overview on bioethanol production from lignocellulosic feedstocks. Chemosphere. 2020;242:125080. doi: 10.1016/j.chemosphere.2019.125080
- Vásquez MP, da Silva JNC, de Souza MB, et al. Appl Biochem Biotecnol. 2007;137:141–153. doi: 10.1007/978-1-60327-181-3_13
- Oberoi HS, Vadlani PV, Saida L, et al. Ethanol production from banana peels using statistically optimized simultaneous saccharification and fermentation process. Waste Manage. 2011;31(7):1576–1584. doi: 10.1016/j.wasman.2011.02.007
- Vargas F, Domínguez E, Vila C, et al. Agricultural residue valorization using a hydrothermal process for second generation bioethanol and oligosaccharides production. Bioresour Technol. 2015;191:263–270. doi: 10.1016/j.biortech.2015.05.035
- Siriwong T, Laimeheriwa B, Aini UN, et al. Cold hydrolysis of cassava pulp and its use in simultaneous saccharification and fermentation (SSF) process for ethanol fermentation. J Biotechnol. 2019;292:57–63. doi: 10.1016/j.jbiotec.2019.01.003
- da Costa Nogueira C, de Araújo Padilha CE, de Jesus AA, et al. Pressurized pretreatment and simultaneous saccharification and fermentation with in situ detoxification to increase bioethanol production from green coconut fibers. Ind Crop Prod. 2019;130:259–266. doi: 10.1016/j.indcrop.2018.12.091
- Akhtar N, Goyal D, Goyal A. Characterization of microwave-alkali-acid pre-treated rice straw for optimization of ethanol production via simultaneous saccharification and fermentation (SSF). Energ Convers Manage. 2017;141:133–144. doi: 10.1016/j.enconman.2016.06.081
- Gao Y, Xu J, Yuan Z, et al. Ethanol production from sugarcane bagasse by fed‐batch simultaneous saccharification and fermentation at high solids loading. Energy Sci Eng. 2018;6(6):810–818. doi: 10.1002/ese3.257
- Koppram R, Olsson L. Combined substrate, enzyme and yeast feed in simultaneous saccharification and fermentation allow bioethanol production from pretreated spruce biomass at high solids loadings. Biotechnol Biofuels. 2014;7(1):1–9. doi: 10.1186/1754-6834-7-54
- Mazaheri D, Orooji Y, Mazaheri M, et al. Bioethanol production from pomegranate peel by simultaneous saccharification and fermentation process. Biomass Convers Biorefinery. 2021;1–9. doi: 10.1007/s13399-021-01562-2
- Chohan NA, Aruwajoye G, Sewsynker-Sukai Y, et al. Valorisation of potato peel wastes for bioethanol production using simultaneous saccharification and fermentation: process optimization and kinetic assessment. Renewable Energy. 2020;146:1031–1040. doi: 10.1016/j.renene.2019.07.042
- Russell I. Chapter 9 - Understanding yeast fundamentals. In: Jacques K, Lyons T, and Kelsall D, editors The alcohol textbook: a reference for the beverage, fuel and industrial alcohol industries. 4 ed. Nottingham, United Kingdom: Nottingham University Press; 2003. p. 531–537.
- Zabed H, Faruq G, Sahu JN, et al. Bioethanol production from fermentable sugar juice. Sci World J. 2014;2014:1–12. doi: 10.1155/2014/957102
- Lim HC, Shin HS. Fed-batch cultures: principles and applications of semi-batch bioreactors. Cambridge University Press; 2013.
- Chang Y-H, Chang K-S, Huang C-W, et al. Comparison of batch and fed-batch fermentations using corncob hydrolysate for bioethanol production. Fuel. 2012;97:166–173. doi: 10.1016/j.fuel.2012.02.006
- Phukoetphim N, Salakkam A, Laopaiboon P, et al. Improvement of ethanol production from sweet sorghum juice under batch and fed-batch fermentations: effects of sugar levels, nitrogen supplementation, and feeding regimes. Electron J Biotechnol. 2017;26:84–92. doi: 10.1016/j.ejbt.2017.01.005
- Siqueira PF, Karp SG, Carvalho JC, et al. Production of bio-ethanol from soybean molasses by Saccharomyces cerevisiae at laboratory, pilot and industrial scales. Bioresour Technol. 2008;99(17):8156–8163. doi: 10.1016/j.biortech.2008.03.037
- Kang KE, Jeong J-S, Kim Y, et al. Development and economic analysis of bioethanol production facilities using lignocellulosic biomass. J Biosci Bioeng. 2019;128(4):475–479. doi: 10.1016/j.jbiosc.2019.04.004
- Amorim HV, Lopes ML, de Castro Oliveira JV, et al. Scientific challenges of bioethanol production in Brazil. Appl Microbiol Biotechnol. 2011;91(5):1267–1275. doi: 10.1007/s00253-011-3437-6
- Azhar SHM, Abdulla R, Jambo SA, et al. Yeasts in sustainable bioethanol production: areview. Biochem Biophys Rep. 2017;10:52–61. doi: 10.1016/j.bbrep.2017.03.003
- Lee J, Lee SY, Park S, et al. Control of fed-batch fermentations. Biotechnol Adv. 1999;17(1):29–48. doi: 10.1016/S0734-9750(98)00015-9
- Nilsson A, Taherzadeh M, Lidén G. On-line estimation of sugar concentration for control of fed-batch fermentation of lignocellulosic hydrolyzates by Saccharomyces cerevisiae. Bioprocess Biosyst Eng. 2002;25(3):183–191. doi: 10.1007/s00449-002-0293-x
- Wang J, Chae M, Sauvageau D, et al. Improving ethanol productivity through self-cycling fermentation of yeast: a proof of concept. Biotechnol Biofuels. 2017;10(1):193. doi: 10.1186/s13068-017-0879-9
- Wang J, Chae M, Bressler DC, et al. Improved bioethanol productivity through gas flow rate-driven self-cycling fermentation. Biotechnol Biofuels. 2020;13(1):14. doi: 10.1186/s13068-020-1658-6
- Ingledew WM. Continuous fermentation in the fuel alcohol industry: how does the technology affect yeast. In: Jacques KA, Lyons TP, Kelsall DR, editors. The alcohol textbook: a reference for the beverage, fuel and industrial alcohol industries. 4 ed. Nottingham, UK:Nottingham University Press; 2003. p. 135–143.
- Karapatsia A, Penloglou G, Chatzidoukas C, et al. Fed-batch Saccharomyces cerevisiae fermentation of hydrolysate sugars: adynamic model-based approach for high yield ethanol production. Biomass Bioenergy. 2016;90:32–41. doi: 10.1016/j.biombioe.2016.03.021
- Parashar A, Jin Y, Mason B, et al. Incorporation of whey permeate, a dairy effluent, in ethanol fermentation to provide a zero waste solution for the dairy industry. J Dairy Sci. 2016;99(3):1859–1867. doi: 10.3168/jds.2015-10059
- Bhargava S, Nandakumar M, Roy A, et al. Pulsed feeding during fed‐batch fungal fermentation leads to reduced viscosity without detrimentally affecting protein expression. Biotechnol Bioeng. 2003;81(3):341–347. doi: 10.1002/bit.10481
- Unrean P, Nguyen NH. Optimized fed-batch fermentation of Scheffersomyces stipitis for efficient production of ethanol from hexoses and pentoses. Appl Biochem Biotechnol. 2013;169(6):1895–1909. doi: 10.1007/s12010-013-0100-y
- Chang Y-H, Chang K-S, Chen C-Y, et al. Enhancement of the efficiency of bioethanol production by Saccharomyces cerevisiae via gradually batch-wise and fed-batch increasing the glucose concentration. Fermentation. 2018;4(2):45. doi: 10.3390/fermentation4020045
- Verstrepen K, Derdelinckx G, Verachtert H, et al. Yeast flocculation: what brewers should know. Appl Microbiol Biotechnol. 2003;61(3):197–205. doi: 10.1007/s00253-002-1200-8
- Bauer FF, Govender P, Bester MC. Yeast flocculation and its biotechnological relevance. Appl Microbiol Biotechnol. 2010;88(1):31–39. doi: 10.1007/s00253-010-2783-0
- Flevaris K, Chatzidoukas C. Optimal fed-batch bioreactor operating strategies for the microbial production of lignocellulosic bioethanol and exploration of their economic implications: a step forward towards sustainability and commercialization. J Clean Prod. 2021;295:126384. doi: 10.1016/j.jclepro.2021.126384
- D’Amato D, Corbo MR, Nobile MAD, et al. Effects of temperature, ammonium and glucose concentrations on yeast growth in a model wine system. Int J Food Sci Technol. 2006;41(10):1152–1157. doi: 10.1111/j.1365-2621.2005.01128.x
- Ávila-Reyes S, Camacho-Díaz B, Acosta-García M, et al. Effect of salt and sugar osmotic stress on the viability and morphology of Saccharomyces boulardii. IJEAB. 2016;1(3):593–602. doi: 10.22161/ijeab/1.3.43
- Sonego J, Lemos D, Cruz A, et al. Optimization of fed-batch fermentation with in situ ethanol removal by CO2 stripping. Energ Fuel. 2018;32(1):954–960. doi: 10.1021/acs.energyfuels.7b02979
- Koppram R, Nielsen F, Albers E, et al. Simultaneous saccharification and co-fermentation for bioethanol production using corncobs at lab, PDU and demo scales. Biotechnol Biofuels. 2013;6(1):1–10. doi: 10.1186/1754-6834-6-2
- Zacchi G, Axelsson A. Economic evaluation of preconcentration in production of ethanol from dilute sugar solutions. Biotechnol Bioeng. 1989;34(2):223–233. doi: 10.1002/bit.260340211
- Fujii T, Murakami K, Endo T, et al. Bench-scale bioethanol production from eucalyptus by high solid saccharification and glucose/xylose fermentation method. Bioprocess Biosyst Eng. 2014;37(4):749–754. doi: 10.1007/s00449-013-1032-1
- Ahmad Q, Manzoor M, Chaudhary A, et al. Bench‐scale fermentation for second generation ethanol and hydrogen production by Clostridium thermocellum DSMZ 1313 from sugarcane bagasse. Environ Prog Sustain Energy. 2021;40(2):e13516. doi: 10.1002/ep.13516
- Bai F, Anderson W, Moo-Young M. Ethanol fermentation technologies from sugar and starch feedstocks. Biotechnol Adv. 2008;26(1):89–105. doi: 10.1016/j.biotechadv.2007.09.002
- Stanley D, Bandara A, Fraser S, et al. The ethanol stress response and ethanol tolerance of Saccharomyces cerevisiae. J Appl Microbiol. 2010;109(1):13–24. doi: 10.1111/j.1365-2672.2009.04657.x
- Liu C-G, Xiao Y, Xia X-X, et al. Cellulosic ethanol production: progress, challenges and strategies for solutions. Biotechnol Adv. 2019;37(3):491–504. doi: 10.1016/j.biotechadv.2019.03.002
- Mussatto SI, Dragone G, Guimarães PM, et al. Technological trends, global market, and challenges of bio-ethanol production. Biotechnol Adv. 2010;28(6):817–830. doi: 10.1016/j.biotechadv.2010.07.001
- Alfenore S, Molina-Jouve C, Guillouet S, et al. Improving ethanol production and viability of Saccharomyces cerevisiae by a vitamin feeding strategy during fed-batch process. Appl Microbiol Biotechnol. 2002;60(1):67–72. doi: 10.1007/s00253-002-1092-7
- Narisetty V, Nagarajan S, Gadkari S, et al. Process optimization for recycling of bread waste into bioethanol and biomethane: a circular economy approach. Energ Convers Manage. 2022;266:115784. doi: 10.1016/j.enconman.2022.115784
- Laopaiboon L, Thanonkeo P, Jaisil P, et al. Ethanol production from sweet sorghum juice in batch and fed-batch fermentations by Saccharomyces cerevisiae. World J Microbiol Biotechnol. 2007;23(10):1497–1501. doi: 10.1007/s11274-007-9383-x
- Lemos DA, Sonego JL, Cruz AJ, et al. Improvement of ethanol production by extractive fed-batch fermentation in a drop column bioreactor. Bioprocess Biosyst Eng. 2020;43(12):2295–2303. doi: 10.1007/s00449-020-02414-5
- Seo H-B, Kim SS, Lee H-Y, et al. High-level production of ethanol during fed-batch ethanol fermentation with a controlled aeration rate and non-sterile glucose powder feeding of Saccharomyces cerevisiae. Biotechnol Bioprocess Eng. 2009;14(5):591–598. doi: 10.1007/s12257-008-0274-2
- Hewitt CJ, Nienow AW. The scale‐up of microbial batch and fed‐batch fermentation processes. Adv Appl Microbiol. 2007;62:105–135. doi: 10.1016/S0065-2164(07)62005-X
- Pinto AS, Pereira SC, Ribeiro MP, et al. Monitoring of the cellulosic ethanol fermentation process by near-infrared spectroscopy. Bioresour Technol. 2016;203:334–340. doi: 10.1016/j.biortech.2015.12.069
- Cozzolino D. State-of-the-art advantages and drawbacks on the application of vibrational spectroscopy to monitor alcoholic fermentation (beer and wine). Appl Spectrosc Rev. 2016;51(4):302–317. doi: 10.1080/05704928.2015.1132721
- Veale EL, Irudayaraj J, Demirci A. An on‐line approach to monitor ethanol fermentation using FTIR spectroscopy. Biotechnol Prog. 2007;23(2):494–500. doi: 10.1021/bp060306v
- Thatipamala R, Rohani S, Hill G. Effects of high product and substrate inhibitions on the kinetics and biomass and product yields during ethanol batch fermentation. Biotechnol Bioeng. 1992;40(2):289–297. doi: 10.1002/bit.260400213
- Zhang Q, Wu D, Lin Y, et al. Substrate and product inhibition on yeast performance in ethanol fermentation. Energ Fuel. 2015;29(2):1019–1027. doi: 10.1021/ef502349v