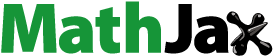
ABSTRACT
Microplastic (MP) is an emerging contaminant of concern due to its abundance in the environment. Wastewater treatment plant (WWTP) can be considered as one of the main sources of microplastics in freshwater due to its inefficiency in the complete removal of small MPs. In this study, a column-based MP removal which could serve as a tertiary treatment in WWTPs is evaluated using granular activated carbon (GAC) as adsorbent/filter media, eliminating clogging problems commonly caused by powder form activated carbon (PAC). The GAC is characterized via N2 adsorption–desorption isotherm, field emission scanning electron microscopy, and contact angle measurement to determine the influence of its properties on MP removal efficiency. MPs (40–48 μm) removal up to 95.5% was observed with 0.2 g/L MP, which is the lowest concentration tested in this work, but still higher than commonly used MP concentration in other studies. The performance is reduced with further increase in MP concentration (up to 1.0 g/L), but increasing the GAC bed length from 7.5 to 17.5 cm could lead to better removal efficiencies. MP particles are immobilized by the GAC predominantly by filtration process by being entangled with small GAC particles/chips or stuck between the GAC particles. MPs are insignificantly removed by adsorption process through entrapment in GAC porous structure or attachment onto the GAC surface.
Introduction
Microplastic (MP), an emerging contaminant that is present abundantly in the aquatic environment, has garnered a lot of interest because of the potential hazards and associated environmental issues due to its small size and affinity toward other contaminants [Citation1]. Several studies have reported the capability of MPs in adsorbing various contaminants such as pesticides, antibiotics, and heavy metals such as cadmium and copper [Citation2–5]. MPs are classified as plastic particles smaller than 5 mm and can be classified into two groups depending on their origin: (i) primary MPs, which consist of plastic particles specifically manufactured in micro-sizes and commonly utilized in personal care products e.g. toothpaste, scrubs, facial cleansers, etc. and (ii) secondary MPs, which are produced due to physical, chemical, or biological degradation of larger sized plastics [Citation6]. In 2016, the reported amount of plastics in the aquatic environment was between 19 and 23 million metric ton and the amount was estimated to exponentially increase to 53 million metric ton in 2030 [Citation7]. Previous studies have also specifically reported on the estimated amounts of MPs in the future based on modelling approaches. van Wijnen et al. [Citation8] estimated that the amount of MP particles in 2050 could be doubled if enhanced waste management is not applied or instead, halved if applied. On the other hand, Everaert et al. [Citation9] utilized meta-analysis using data available in the literature and reported that the amount of MP is expected to increase from 9.6 to 48.8 particles/m3 in 2100. The best course of action is to reduce the usage of plastics, especially those of single use; however, this initiative is only expected to produce a substantial impact in the long run. Therefore, significant efforts need to be taken to mitigate current MP pollution in the fresh and ocean waters.
Various studies reported that conventional wastewater treatment plants (WWTPs) are able to remove MP particles with removal efficiencies of greater than 90% [Citation10]. Most WWTPs are not built for the removal of small MP particles; however, large MP particles can be practically eliminated at the primary (screening and grit chamber) and secondary (biological) treatment stages [Citation11]. Nonetheless, due to their small and lightweight properties, smaller-sized MP particles (especially those below 100 μm in size) can be resistant to removal by conventional WWTP operations as these particles easily escape all treatment stages due to their miniscule size. Considering the huge influx of water treated daily in WWTPs, large amounts of MP particles are able to exit the effluent stream, thus making WWTP a consistent source of MP contamination in fresh water [Citation12]. The amount of MP particles present in the effluent stream is strongly dependent on the size and operation of the WWTP and the amount of wastewater treated, which significantly influences the amount of MP present in the influent stream. Two WWTPs in Adana, Turkey, reported that their influent wastewater contained 1–6.5 million of MP particles daily, which were reduced to 220,000–1.5 million particles in the effluent [Citation13]. Similarly, three WWTPs in South Carolina, USA, reported a discharge of 500–1000 million MP particles per day, which were reduced from 1000 to 20,000 million MP present in the effluent [Citation14]. Additionally, MPs retained by the WWTPs are contained in the generated sludge, which would result in the release of MP particles in soil as the sludge is commonly processed and reused as agricultural fertilizer [Citation15–17]. Proper MP removal method is desperately needed to reduce its release into the environment to complement or become an alternative treatment method to coagulation, flocculation. and sedimentation (CFS), filtration, and membrane bioreactor, which have been studied for MP removal with varying degrees of efficiency.
Column-based removal is an appealing and effective method in removing various pollutants e.g. heavy metals, dyes, pharmaceuticals, etc. from aquatic environments [Citation18–20]. Various types of media have been utilized in column-based pollutant removals e.g. activated carbon, biochar, zeolites, molecular sieves, etc. The utilization of these materials provides additional benefits to the overall process due to their affordability and accessibility. Commercial products such as granular activated carbon (GAC) are highly preferred as the particle size can be controlled to enhance MP removal, address clogging issues, and facilitate regeneration process. Parameters such as pollutant concentration and bed length are essential in the design of columns/reactors to achieve the optimized column removal of pollutants [Citation19,Citation21,Citation22]. Even though column-based removal is typically utilized for dissolved pollutants, various studies have reported the capability of this method in removing suspended solids present in the wastewater [Citation23,Citation24]. Based on this observation, MP particles which are commonly suspended in various aquatic environments theoretically can be eliminated via column-based removal.
Few studies have reported the removal of particles from aquatic environments via column-based methods with reported removal efficiencies between 83.5% and 100% [Citation12,Citation25–27]. The method consists of passing MP-containing water into columns filled with media, which acts to immobilize the MP particles, resulting in cleaner water in the effluent stream. MP particles may be immobilized by the media through two possible means: (i) by filtration as the MP particles are unable to pass through the media bed inside the column or (ii) by adsorption on the media surface through electrostatic attraction, hydrophobic interaction, and hydrogen bonding [Citation12,Citation28,Citation29]. However, the majority of the studies reporting on MP column-based removal does not include studies on the effects of different MP concentrations and media bed lengths, which are essential for the design of the column to achieve high performance. In this study, the removal of MP-containing wastewater using a laboratory-scale glass column with GAC as the filter media/adsorbent was evaluated in a continuous-flow experiment. The properties of the GAC media e.g. porosity, surface morphology, and hydrophobicity were characterized and analyzed to determine their role in facilitating the GAC to remove MP particles. Two parameters, i.e. MP concentration and GAC bed length, were varied to investigate their influence on the MP removal efficiency by the column-based removal method.
Materials and methods
Materials
MPs (polyethylene, Sigma Aldrich) of particle size between 40 and 48 μm were employed as the model MP in this study and used as received. GAC (charcoal activated granular, Bendosen) utilized as the adsorbent/filter media were commercially purchased. The GAC particle size is between 1.16 and 2.5 mm based on particle size analysis. Ethanol (denatured, 99.9%, R&M Chemicals) was utilized in the preparation of synthetic MP-containing wastewater.
Characterization of GAC
Surface area and porosity of the GAC were characterized via N2 adsorption–desorption isotherms (Tristar 3000) at 77.35 K, using Brunauer–Emmett–Teller (BET) and Barrett–Joyner–Halenda (BJH) model, respectively. The morphology of the GAC was identified using field emission scanning electron microscope (Zeiss Leo 1525) with type II secondary electron image (SE2) signal at magnification of ×5000.
The hydrophobicity/hydrophilicity of the GAC was determined using sessile droplet contact angle measurement [Citation30]. The contact angle was determined using ImageJ software with contact angle plugins. The measurement was conducted at different points on the GAC surface, and the value reported is an average of three measurements. Based on the contact angle, the GAC is classified as follows: hydrophilic (θ < 90°), hydrophobic (90° < θ < 150°), and superhydrophobic (>150°) [Citation31].
Synthetic MP-containing wastewater
The model synthetic wastewater utilized in this study was prepared by spiking a known concentration of polyethylene into a mixture of distilled water and ethanol (9:1). Ethanol was added to reduce the surface tension of the mixture and increase the dispersion of the MP particles throughout the solution [Citation32,Citation33]. The mixture was magnetically stirred for 30 min prior to the removal study to further enhance MP dispersion, and stirred throughout the study to maintain good dispersion of MP particles in the water sample.
Column-based removal
MP removal study was conducted in a column as shown in the schematic diagram of the experimental setup shown in .
The glass column (length = 20 cm, outer diameter = 15 mm) was filled with GAC as adsorbent/filter media at variable bed length and mass. The GAC was supported by glass beads (diameter = 5 mm) on top and bottom of the filter bed to facilitate the flow of water samples containing MP particles through the column. A very thin layer of wool was placed between the media and bottom glass beads to prevent the GAC being flushed into the effluent stream. Assuming that GAC particles are spherical, the bed porosity of GAC inside the column is 0.4240, calculated using EquationEquation 1(1)
(1) , where ε represents mean porosity, D represents column diameter (mm), and d refers to particle diameter (mm) [Citation34]. The bed porosity in this study is well within the typical range of bed porosity (ε = 0.2595–0.4764) utilized in various chemical engineering operations e.g. separation and reaction processes [Citation35].
MP-containing wastewater was passed from the upper side of the column to the bottom gravitationally with the assistance of a peristaltic pump (Cole Parmer Easy-Load MasterFlex 7518-00) at a flow rate of 7 ml/min. Two parameters evaluated in this study are the MP concentration (0.2–1.0 g/L) and GAC bed length (7.5–15 cm). The MP removal process was conducted continuously for 2 h, and the effluent was visually observed to determine the breakthrough time. Each experiment was conducted in triplicates and the average values were reported.
MP removal performance was determined by the gravimetric method where the effluent collected was filtered using a filter paper (Smith 102 Qualitative), dried at room temperature, and weighed with an analytical balance. The difference in the mass of filter paper before and after filtration is considered as the mass of MP present in the effluent. Due to the possible presence of impurities in the distilled water utilized, an identical amount of distilled water was filtered and considered as a blank sample. The removal efficiency of the process was calculated using EquationEquation 2.(2)
(2)
For quality control, several measures were taken throughout the experiment to prevent cross-contamination from the surroundings. The whole experiments were conducted in an enclosure, which can be accessed only by the hands of the laboratory personnel. The laboratory personnel have also worn pure cotton clothes and latex gloves to prevent fiber contamination from the worn attire. All experiments were conducted in triplicates, and the results are the average values obtained. All samples and related apparatus were covered with aluminum foil to reduce possible contamination with atmospheric MP.
Results
Characterization of GAC
Nitrogen adsorption–desorption isotherms of the GAC used are shown in . GAC exhibits a Type IV(a) isotherm with H4 hysteresis throughout the range of P/P0 tested, as shown in . This represents a material of microporous–mesoporous type with characteristics of capillary condensation as indicated by the hysteresis [Citation36]. The commercial GAC utilized in this study possesses a surface area of 682.24 m2/g, average pore width of 1.88 nm, and average pore volume of 0.032 cm3/g. Note that this implies that the pores of the GAC media are significantly smaller than the average particle size of the MP utilized in this study.
The surface morphology and the contact angle of the GAC surface are shown in , respectively.
The surface morphology of GAC shown in at magnification of ×5000 indicates that the surface of the GAC is very rough and porous, in agreement with the findings from N2 adsorption–desorption isotherm analysis, which shows that GAC possesses pores in the microporous and mesoporous regions with abundance of the former. The contact angle measurement shown in shows that the water contact angle of the GAC surface is 108.8°, implying that the surface is slightly hydrophobic in nature [Citation31].
Effect of MP concentration
The performance of MP removal at different initial concentrations (0.2–1.0 g/L) by a constant GAC bed length of 7.5 cm is shown in and summarized in .
Figure 4. Removal efficiencies of MP at varying initial concentrations (0.2–1.0 g/L) using GAC with a fixed bed length of 7.5 cm.

Table 1. The performance of MP removal by GAC at different MP concentrations (0.2–1.0 g/L).
As can be observed in and , the performance of GAC in eliminating MP decreases with an increase in MP concentration. MP removal obtained at the lowest MP concentration (0.2 g/L) is 95.5%, but this is immediately dropped to values below 90% with further increase in MP concentration and eventually to 59.2% with the highest MP concentration tested (1.0 g/L). As the GAC utilized in this study is in granular shape, the packing of the materials is less compact than smaller-sized media e.g. powder [Citation37,Citation38]. Having more space in the GAC bed results in the higher possibility of small-sized MPs passing through the available pathways. As the MP concentration increases, greater amounts of MP particles are able to pass through the GAC bed, leading to deterioration in the performance of MP removal. MP removal efficiency obtained in this study can be compared with other related column-based MP removal studies, summarized in .
Table 2. Comparison of MP removal efficiency of this study with previous column-based MP removal studies.
shows that the MP removal efficiency obtained in this study is comparable to other column-based MP removal work, although having slightly lower performance. It should be emphasized that the MP concentration utilized in this study is significantly higher than typical values in literature between 1 and 40 mg/L [Citation33,Citation42–44]. A higher working concentration was selected in this study by taking into account the predicted increase in the MP concentration in water bodies in the near future [Citation7]. Based on the observed performance, GAC column-based removal can be considered suitable for removing MP particles up to 0.2 g/L, which is five times greater than the maximum concentration utilized in other studies. In addition, optimizing other process parameters e.g. GAC bed length may further enhance the removal efficiency of the process.
Varying GAC bed length
Based on the previous section, an MP concentration of 0.8 g/L was selected to further evaluate the possible enhancement of MP removal by varying the GAC bed length. The removal efficiencies of MP at different GAC bed lengths (7.5–17.5 cm) are shown in and summarized in .
Table 3. Removal efficiencies of 0.8 g/L MP by GAC of different bed lengths (7.5–17.5 cm).
Initially, no significant change is observed by increasing the GAC bed length from 7.5 to 10 cm. Further increase of GAC bed length to 12.5 and 15 cm results in an enhancement of MP removal efficiencies up to 82.4% and 87.1%, respectively. The performance then slightly dropped to 86.7% with the highest GAC bed length of 17.5 cm; nonetheless, this is still higher than the other shorter GAC beds (7.5–12.5 cm). This implies that GAC is capable of retaining or adsorbing more MPs as the particles traveled through longer beds. The enhancement in the removal efficiency may be ascribed to the greater number of binding sites available and higher possible collisions between the MP and GAC particles with longer GAC bed length [Citation43]. Based on these results, the process parameters should be optimized prior to conducting column-based MP removal as a slight variation of these parameters (MP concentration, bed length, etc.) could result in a significant reduction in the performance of the method in retaining MP particles.
Discussion
As previously discussed, MP may be removed by column media through filtration, adsorption, or a combination of both processes. In this study, MP is conceivably immobilized and retained physically by the GAC media either by being (i) stuck between the GAC particles, (ii) entangled with small GAC particles or chips, (iii) trapped in the GAC porous structure, or (iv) adhered onto the GAC surface [Citation12].
Based on experimental observation, it is presumable that as the MP-containing water was flowing through the GAC bed, most of the MP particles were filtered as they were unable to pass through the available pores/pathways between the GAC packing [Citation37], as shown in . Most of the MP particles are stuck on the upper layer of the GAC bed, eventually resulting in cake formation.
The cake layer can be removed through a backwashing process where water, air, or a mixture of water–air is passed through the column in a direction opposite to that used in the MP removal study. The collected backwashed water can be filtered where the MP particles can be recovered and subsequently recycled and reutilized or properly removed if the plastic particles are non-reusable. The fact that backwashing can facilitate the recovery of MP particles retained by GAC particles also makes this method more favorable.
In addition, the study on the effect of GAC bed length on MP removal is highly important toward scaling up the process for practical application. To obtain an optimized MP removal efficiency and a high recovery of MP, bed expansion between 25% and 30% is suggested [Citation45,Citation46]. Therefore, an ideal length of the column should be at least between 18.75 and 19.5 cm based on the optimal GAC bed length (15 cm) and the suggested bed expansion.
As previously shown, the surface morphology of GAC is very rough and porous. This facilitates the entanglement of MP particles inside the grooves on the GAC particle surface. This is similar to the result shown by Shen et al. [Citation26], where MP particles were found to be trapped on the rough zeolite surface but not on the smoother surface of molecular sieve.
Based on GAC characterization and cake formation, the removal process may be predominantly occurring by the first two mechanisms. The size of MP utilized in this study is also significantly larger than the pore size of the GAC utilized in this study; thus, it is impossible for the MP particles to enter the pore structure and get trapped inside. The GAC surfaces are only slightly hydrophobic, indicating weak retention ability, and thus only small parts of MP are perceivably attached onto the GAC surface through adsorption [Citation26]. This can be further supported by the physical conditions of the GAC particles after undergoing an MP removal process as shown in after taken out from the column in comparison to the GAC prior to the removal process.
Based on the figure, only a small amount of MP particles is visibly attached onto the surface of the GAC particles compared to the amount of MP forming cake at the top of the GAC bed surface. As filtration is assumed to be the main mechanism of MP removal in this column-based method while adsorption is expected to only contribute to a small part of the removal process, the study on adsorption isotherm is not applicable.
In the context of filtration, various filtration membranes have been reported to successfully eliminate MP particles from wastewater. However, most of the membrane filters are produced from plastic-based materials such as polycarbonate (PC), polytetrafluoroethylene (PTFE), polyvinylidene fluoride (PVDF), and cellulose acetate [Citation47–49]. Prolonged usage of membrane filters made from these materials would result in abrasion of the membrane and consequently resulting in secondary pollution of plastic particles [Citation47,Citation50,Citation51]. On the other hand, the utilization of GAC in a column-based removal can prevent the possibility of a secondary pollution, making the method more appealing and advantageous.
For further improvement of the process, necessary modifications should be employed to enhance the removal performance, especially at higher MP concentrations. The column porosity could be slightly reduced by utilizing smaller-sized GAC to enable higher retention of MP particles by the GAC particles. It should be emphasized that a reduction in column porosity would lead to higher head loss, which would be detrimental toward water flow inside the column; therefore, a balanced condition between these two parameters should be achieved [Citation52,Citation53]. In addition, the surface of the GAC particles may be modified with suitable surfactants, which would allow better interaction between the GAC and MP particles through electrostatic attraction or hydrophobic interaction [Citation26].
Conclusion
In this work, synthetic water samples containing high MP concentration were subjected to a column-based continuous flow removal using commercial GAC as adsorbent/filter media. The method performed quite well with the highest MP removal of 95.5% at 0.2 g/L MP. Both initial MP concentration and GAC bed length were found to be significant factors in affecting the MP removal efficiency. The performance was reduced significantly with higher MP concentrations up to 1.0 g/L as the GAC became unable to accommodate and immobilize higher amounts of MP particles. At constant MP concentration (0.8 g/L), the MP removal performance was significantly enhanced to 87.1% by increasing the GAC bed length. Even though high removal efficiency is attainable through this study, the result implies that various other process parameters and proper designing should be considered for a column-based MP removal to be utilized and upscaled as the properties of MP-containing water may differ from one place to another.
Author contributions statement
Nik Nor Amirah Mohd Napi: Writing original draft and analysis and interpretation of the data. Naimah Ibrahim: Analysis and interpretation of the data and conceptualization, review, and editing. Muhammad Adli Hanif: Analysis and interpretation of the data. Masitah Hasan: Analysis and interpretation of the data. Farrah Aini Dahalan: Analysis and interpretation of the data. Achmad Syafiuddin: Analysis and interpretation of the data and conceptualization, review, and editing. Raj Boopathy: Analysis and interpretation of the data and conceptualization, review, and editing.
Disclosure statement
No potential conflict of interest was reported by the author(s).
Data availability statement
The data that support the findings of this study are available from the corresponding author upon reasonable request.
Additional information
Funding
References
- Lin L, Tang S, Wang X, et al. Hexabromocyclododecane alters malachite green and lead(II) adsorption behaviors onto polystyrene microplastics: interaction mechanism and competitive effect. Chemosphere. 2021;265:129079. doi:10.1016/j.chemosphere.2020.129079
- Li J, Zhang K, Zhang H. Adsorption of antibiotics on microplastics. Environ Pollut. 2018;237:460–11. doi:10.1016/j.envpol.2018.02.050
- Brennecke D, Duarte B, Paiva F, et al. Microplastics as vector for heavy metal contamination from the marine environment. Estuar Coast Shelf Sci. 2016;178:189–195. doi:10.1016/j.ecss.2015.12.003
- Wang F, Yang W, Cheng P, et al. Adsorption characteristics of cadmium onto microplastics from aqueous solutions. Chemosphere. 2019;235:1073–1080. doi: 10.1016/j.chemosphere.2019.06.196
- Wang T, Yu C, Chu Q, et al. Adsorption behavior and mechanism of five pesticides on microplastics from agricultural polyethylene films. Chemosphere. 2020;244:125491. doi:10.1016/j.chemosphere.2019.125491
- Ganie ZA, Khandelwal N, Tiwari E, et al. Biochar-facilitated remediation of nanoplastic contaminated water: effect of pyrolysis temperature induced surface modifications. J Hazard Mater. 2021;417:126096. doi:10.1016/j.jhazmat.2021.126096
- Borrelle SB, Ringma J, Law KL, et al. Predicted growth in plastic waste exceeds efforts to mitigate plastic pollution. Science. 2020;369(6510):1515–1518. doi: 10.1126/science.aba3656
- van Wijnen J, Ragas AMJ, Kroeze C. Modelling global river export of microplastics to the marine environment: sources and future trends. Sci Total Environ. 2019;673:392–401. doi:10.1016/j.scitotenv.2019.04.078
- Everaert G, Van Cauwenberghe L, De Rijcke M, et al. Risk assessment of microplastics in the ocean: modelling approach and first conclusions. Environ Pollut. 2018;242:1930–1938. doi: 10.1016/j.envpol.2018.07.069
- Rajala K, Grönfors O, Hesampour M, et al. Removal of microplastics from secondary wastewater treatment plant effluent by coagulation/flocculation with iron, aluminum and polyamine-based chemicals. Water Res. 2020;183:116045. doi:10.1016/j.watres.2020.116045
- Reddy AS, Nair AT. The fate of microplastics in wastewater treatment plants: an overview of source and remediation technologies. Environ Technol Innov. 2022;28:102815. doi:10.1016/j.eti.2022.102815
- Wang Z, Sedighi M, Lea-Langton A. Filtration of microplastic spheres by biochar: removal efficiency and immobilisation mechanisms. Water Res. 2020;184:116165. doi:10.1016/j.watres.2020.116165
- Gündoğdu S, Çevik C, Güzel E, et al. Microplastics in municipal wastewater treatment plants in Turkey: a comparison of the influent and secondary effluent concentrations. Environ Monit Assess. 2018;190(11):626. doi: 10.1007/s10661-018-7010-y
- Conley K, Clum A, Deepe J, et al. Wastewater treatment plants as a source of microplastics to an urban estuary: removal efficiencies and loading per capita over one year. Water Res X. 2019;3:100030. doi:10.1016/j.wroa.2019.100030
- Bretas Alvim C, Mendoza-Roca JA, Bes-Piá A. Wastewater treatment plant as microplastics release source – quantification and identification techniques. J Environ Manage. 2020;255:109739. doi:10.1016/j.jenvman.2019.109739
- Kay P, Hiscoe R, Moberley I, et al. Wastewater treatment plants as a source of microplastics in river catchments. Environ Sci Pollut Res. 2018;25(20):20264–20267. doi: 10.1007/s11356-018-2070-7
- Iyare PU, Ouki SK, Bond T. Microplastics removal in wastewater treatment plants: a critical review. Environ Sci Water Res Technol. 2020;6(10):2664. doi: 10.1039/D0EW00397B
- Silva MC, Spessato L, Silva TL, et al. H3PO4–activated carbon fibers of high surface area from banana tree pseudo-stem fibers: adsorption studies of methylene blue dye in batch and fixed bed systems. J Mol Liq. 2021;324:114771. doi: 10.1016/j.molliq.2020.114771
- Feizi F, Sarmah AK, Rangsivek R. Adsorption of pharmaceuticals in a fixed-bed column using tyre-based activated carbon: experimental investigations and numerical modelling. J Hazard Mater. 2021;417:126010. doi:10.1016/j.jhazmat.2021.126010
- Patel H. Batch and continuous fixed bed adsorption of heavy metals removal using activated charcoal from neem (Azadirachta indica) leaf powder. Sci Rep. 2020;10(1):16895. doi: 10.1038/s41598-020-72583-6
- Ajmani A, Patra C, Subbiah S, et al. Packed bed column studies of hexavalent chromium adsorption by zinc chloride activated carbon synthesized from Phanera vahlii fruit biomass. J Environ Chem Eng. 2020;8(4):103825. doi: 10.1016/j.jece.2020.103825
- Iheanacho OC, Nwabanne JT, Obi CC, et al. Packed bed column adsorption of phenol onto corn cob activated carbon: linear and nonlinear kinetics modeling. South Afr J Chem Eng. 2021;36:80–93. doi:10.1016/j.sajce.2021.02.003
- Patel P, Gupta S, Mondal P. Modeling of continuous adsorption of greywater pollutants onto sawdust activated carbon bed integrated with sand column. J Environ Chem Eng. 2022;10(2):107155. doi: 10.1016/j.jece.2022.107155
- Ranjan Rout D, Mohan Jena H. Synthesis of novel reduced graphene oxide decorated β-cyclodextrin epichlorohydrin composite and its application for Cr(VI) removal: batch and fixed-bed studies. Sep Purif Technol. 2022;278:119630. doi:10.1016/j.seppur.2021.119630
- Siipola V, Pflugmacher S, Romar H, et al. Low-cost biochar adsorbents for water purification including microplastics removal. Appl Sci. 2020;10(3):788. doi: 10.3390/app10030788
- Shen M, Hu T, Huang W, et al. Removal of microplastics from wastewater with aluminosilicate filter media and their surfactant-modified products: performance, mechanism and utilization. Chem Eng J. 2021;421:129918. doi:10.1016/j.cej.2021.129918
- Tong M, He L, Rong H, et al. Transport behaviors of plastic particles in saturated quartz sand without and with biochar/Fe3O4-biochar amendment. Water Res. 2020;169:115284. doi:10.1016/j.watres.2019.115284
- Abdoul Magid ASI, Islam MS, Chen Y, et al. Enhanced adsorption of polystyrene nanoplastics (PSNPs) onto oxidized corncob biochar with high pyrolysis temperature. Sci Total Environ. 2021;784:147115. doi: 10.1016/j.scitotenv.2021.147115
- Zhang Y, Luo Y, Guo X, et al. Charge mediated interaction of polystyrene nanoplastic (PSNP) with minerals in aqueous phase. Water Res. 2020;178:115861. doi: 10.1016/j.watres.2020.115861
- Kumarasiri A, Amarasinghe DAS, Attygalle D. Surface wettability Analysis of nichrome alloy based on the measurements of sessile droplet contact angles. MERCon 2020 - 6th International Multidisciplinary Moratuwa Engineering Research Conference, Proceedings, University of Moratuwa, Katubedda, 10400 Sri Lanka. 2020, pp. 160–164.
- Rius-Ayra O, Biserova-Tahchieva A, LLorca-Isern N. Surface-functionalised materials for microplastic removal. Mar Pollut Bull. 2021;167:112335. doi:10.1016/j.marpolbul.2021.112335
- Chen Y-J, Chen Y, Miao C, et al. Metal–organic framework-based foams for efficient microplastics removal. J Mater Chem A. 2020;8(29):14644–14652. doi: 10.1039/D0TA04891G
- Yuan F, Yue L, Zhao H, et al. Study on the adsorption of polystyrene microplastics by three-dimensional reduced graphene oxide. Water Sci Technol. 2020;81(10):2163–2175. doi:10.2166/wst.2020.269
- Guo Z, Sun Z, Zhang N, et al. Mean porosity variations in packed bed of monosized spheres with small tube-to-particle diameter ratios. Powder Technol. 2019;354:842–853. doi:10.1016/j.powtec.2019.07.001
- Zhang W, Thompson KE, Reed AH, et al. Relationship between packing structure and porosity in fixed beds of equilateral cylindrical particles. Chem Eng Sci. 2006;61(24):8060–8074. doi: 10.1016/j.ces.2006.09.036
- Thommes M, Kaneko K, Neimark AV, et al. Physisorption of gases, with special reference to the evaluation of surface area and pore size distribution (IUPAC Technical Report). Pure Appl Chem. 2015;87(9–10):1051–1069. doi: 10.1515/pac-2014-1117
- Wang Q, Hernández-Crespo C, Santoni M, et al. Horizontal subsurface flow constructed wetlands as tertiary treatment: can they be an efficient barrier for microplastics pollution? Sci Total Environ. 2020;721:137785. doi:10.1016/j.scitotenv.2020.137785
- Wang Q, Hernández-Crespo C, Du B, et al. Fate and removal of microplastics in unplanted lab-scale vertical flow constructed wetlands. Sci Total Environ. 2021;778:146152. doi:10.1016/j.scitotenv.2021.146152
- Na SH, Kim M-J, Kim J-T, et al. Microplastic removal in conventional drinking water treatment processes: performance, mechanism, and potential risk. Water Res. 2021;202:117417. doi: 10.1016/j.watres.2021.117417
- Zhang Y, Diehl A, Lewandowski A, et al. Removal efficiency of micro- and nanoplastics (180 nm – 125 μm) during drinking water treatment. Sci Total Environ. 2020;720:137383. doi:10.1016/j.scitotenv.2020.137383
- Kim KT, Park S. Enhancing microplastics removal from wastewater using electro-coagulation and granule-activated carbon with thermal regeneration. Processes. 2021;9(4):617. doi: 10.3390/pr9040617
- Arenas LR, Gentile SR, Zimmermann S, et al. Nanoplastics adsorption and removal efficiency by granular activated carbon used in drinking water treatment process. Sci Total Environ. 2021;791:148175. doi:10.1016/j.scitotenv.2021.148175
- Batool A, Valiyaveettil S. Surface functionalized cellulose fibers – a renewable adsorbent for removal of plastic nanoparticles from water. J Hazard Mater. 2021;413:125301. doi:10.1016/j.jhazmat.2021.125301
- Wang Z, Sun C, Li F, et al. Fatigue resistance, re-usable and biodegradable sponge materials from plant protein with rapid water adsorption capacity for microplastics removal. Chem Eng J. 2021;415:129006. doi:10.1016/j.cej.2021.129006
- Umar M, Singdahl-Larsen C, Ranneklev SB. Microplastics removal from a plastic recycling industrial wastewater using sand filtration. Water (Switzerland). 2023;15(5):896. doi: 10.3390/w15050896
- Funck M, Al-Azzawi MSM, Yildirim A, et al. Release of microplastic particles to the aquatic environment via wastewater treatment plants: the impact of sand filters as tertiary treatment. Chem Eng J. 2021;426:130933. doi: 10.1016/j.cej.2021.130933
- Golgoli M, Khiadani M, Shafieian A, et al. Microplastics fouling and interaction with polymeric membranes: a review. Chemosphere. 2021;283:131185. doi: 10.1016/j.chemosphere.2021.131185
- Akarsu C, Kumbur H, Kideys AE. Removal of microlasticsfrom wastewater throughelectrocoagulation-electroflotation and membrane filtration processes. Water Sci Technol. 2021;84(7):1648. doi:10.2166/wst.2021.356
- Pramanik BK, Pramanik SK, Monira S. Understanding the fragmentation of microplastics into nano-plastics and removal of nano/microplastics from wastewater using membrane, air flotation and nano-ferrofluid processes. Chemosphere. 2021;282:131053. doi:10.1016/j.chemosphere.2021.131053
- Ding H, Zhang J, He H, et al. Do membrane filtration systems in drinking water treatment plants release nano/microplastics? Sci Total Environ. 2021;755:142658. doi: 10.1016/j.scitotenv.2020.142658
- Pizzichetti ARP, Pablos C, Álvarez-Fernández C, et al. Evaluation of membranes performance for microplastic removal in a simple and low-cost filtration system. Case Stud Chem Environ Eng. 2021;3:100075. doi:10.1016/j.cscee.2020.100075
- Li Z, Wan J, Zhan H, et al. Particle size distribution on Forchheimer flow and transition of flow regimes in porous media. J Hydrol. 2019;574:1–11. doi:10.1016/j.jhydrol.2019.04.026
- van Lopik JH, Sweijen T, Hartog N, et al. Contribuição a perda de carga hidráulica devido penetração parcial e preenchimento anelar de poços: implicações para rebaixamento de nível freático e poços de recarga artificial. Hydrogeol J. 2021;29(2):875–893. doi: 10.1007/s10040-020-02228-5