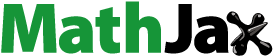
ABSTRACT
Microalgae are capable of generating numerous metabolites that possess notable biological activities and hold substantial promise for various industrial applications. Nevertheless, the taxonomic diversity of these photosynthetic microorganisms has not received thorough investigation. Using the 18S rRNA encoding gene, a recently discovered strain originating from the Tunisian coast (the governorate of Mahdia) was identified as a member of the Porphyridium genus. The growth response as well as the metabolite accumulation of Porphyridium sp. to different culture media (Pm, F/2, and Hemerick) was investigated over a period of 52 days. The highest biomass production was recorded with Pm medium (2 × 107 cell/mL). The apparent growth rates (µ) and the doubling time (Dt) were about 0.081 day−1 and 12.34 days, respectively. The highest chlorophyll a (0.678 ± 0.005 pg/cell), total carotenoids (0.18 ± 0.003 pg/cell), phycoerythrin (3.88 ± 0.003 pg/cell), and proteins (14.58 ± 0.35 pg/cell) contents were observed with F/2 medium. Cultivating Porphyridium sp. in both F/2 and Hemerick media yielded similar levels of starch accumulation. The Hemerick medium has proven to be the most suitable for the production of lipids (2.23% DW) and exopolysaccharides (5.41 ± 0.56 pg/cell).
1. Introduction
Aquatic ecosystems serve as vast reservoirs for cyanobacteria and microalgae, contributing significantly to the Earth’s primary production. As photosynthetic microorganisms, they synthesize numerous organic compounds utilizing solar energy and CO2. Therefore, microalgae serve as a renewable and environmental-friendly feedstock for the production of numerous high-value bio-products including proteins [Citation1], fatty acids [Citation2], pigments [Citation3], and carbohydrates [Citation4]. Red marine microalgae from the genera Rhodella and Porphyridium have attracted considerable attention among the microalgae utilized in industrial applications [Citation3,Citation5]. Especially, these microalgae accumulate multiple valuable molecules such as extracellular polysaccharides, proteins, phycobiliproteins (PBP), pigments, and long-chain poly-unsaturated fatty acids. Extracellular polysaccharides are one of the principal targeted substances known for the beneficial health effects due to their potential biological activities such as antibacterial, antiviral, anticancer, and antioxidant [Citation5–7]. Meanwhile, PBP are encouraging fluorescent labeling reagents that can be used in fluorescence microscopy, immuno-histochemistry, fluorescence immunoassay, flow cytometry, and other biomedical investigations [Citation8–10]. In Porphyridium, the main targets of their PBP molecules are phycoerythrin and allophycocyanin [Citation11]. Additionally, Porphyridium could accumulate a relatively great level of proteins (between 15% and 47% DW) or/and carbohydrates (between 23% and 52% DW) [Citation12–15]. Cultivation of Porphyridium species has been previously investigated in either pilot or laboratory scale. Currently, several information are accessible from the species of Porphyridium sordidum [Citation16,Citation17] Porphyridium purpureum [Citation13,Citation18], Porphyridium cruentum [Citation19–21], and Porphyridium aerugineum [Citation22,Citation23], including the biochemical compositions and growth characteristics. Briefly, the growth characteristics as well as the biochemical profile of Porphyridium family are varied depending on the species [Citation17] as well as the cultivation methods [Citation18]. Bioactive molecules derived from Porphyridium spp. have been integrated into skincare and facial products, including items such as HydrintenseTM featuring Porphyridium purpureum extract by Givaudan (Vernier, Switzerland), Renouvellance containing phycoerythrin and exopolysaccharides from Porphyridium cruentum by Microphyt (Baillargues, France), Silidine® offered by Greentech Biotechnologies (Saint Beauzire, France), comforting soothing cream featuring Porphyridium cruentum extract by algoVita (Tunis, Tunisia), and Ridium® incorporating bioactive exopolysaccharides sourced from Porphyridium cruentum provided by Xelliss (Capellen, Luxembourg).
In the production of microalgal metabolites, one of the main aims is to select an appropriate nutrient medium [Citation24]. The selection of this medium mainly depends on its chemical composition [Citation25]. Nutrients may be the limiting factors impacting the quality as well as the quantity of the biomass and metabolites produced by microalgae. Typically, there are two main categories of culture media employed for cultivating microalgae: (i) synthetic media and (ii) alternative culture media [Citation26]. Synthetic culture media are precisely formulated media created from chemical components, encompassing organic elements such as proteins, sugars, amino acids, thiamine, biotin, and riboflavin, as well as inorganic nutrients like trace metals and salts. Additionally, they may include non-nutrient factors such as hormones and growth-stimulating factors. Synthetic media are characterized by predetermined compositions and are readily accessible in the market. Examples of commercially available synthetic media include Blue Green 11 medium (BG11), Bold’s Basal medium (BBM), Acidified BBM, Zarrouk’s medium, Half Strength Chu 10, Fog’s medium, Modified Chu 13, and f/2, among others [Citation27,Citation28]. However, alternative culture media refer to those obtained from plant sources, swine wastewater, agricultural residues [Citation29], industrial wastewater [Citation30,Citation31], sewage water, municipal wastewater, and similar sources. The production of synthetic media is environmentally unsustainable, involving significant costs and resulting in high greenhouse gas emissions [Citation32,Citation33]. The pursuit of affordable alternative media is motivated by concerns about both sustainability and cost reduction, aiming to render algal products economically feasible for extensive production.
As the microalgal industry is expanding, there is a need to isolate and characterize high-performing strains. In this respect, we aimed in the current study at isolating and molecularly identifying a native microalga from Tunisian coast. Once isolated and identified, the most economically suitable medium for culturing this newly isolated strain was investigated in order to study its biotechnological potentialities for future industrial-scale valorization in biotechnological fields.
2. Materials and methods
2.1. Isolation of axenic monoclonal strain
The Rhodophyta strain was isolated from Mahdia seawater in central-eastern Tunisia (Latitude: 35°30′ N; Longitude: 11°04′ E). Seawater samples were filtered first using a 60 μm pore size membrane to remove protozoa. Then, the filtered seawater was passed through a 2.5 μm pore size membrane to remove bacteria [Citation34]. The membrane was immersed into 50 mL of modified Provasoli medium (Pm), previously described by Ben Hlima et al. [Citation35] consisting of NaCl (28 g/L), MgSO4, 7 H2O (7.2 g/L), NaNO3 (1.7 g/L), K2HPO4 (0.9 g/L), CaCl2, 2 H2O (1.55 g/L), 1 mL/L of trace metal solution composed of MnCl2,4 H2O (2 mg/L), H3BO3 (2 mg/L), (NH4)6Mo7O24,4 H2O (2 mg/L), CuSO4,5 H2O (0.08 mg/L), ZnSO4,7 H2O (213 mg/L), and CoSO4 (0.08 mg/L), and 1 mL/L of vitamin solution containing thiamine HCl (Vitamin B1) (0.1 g/L), cyanocobalamin (Vitamin B12) (0.5 mg/L), and Biotin (vitamin H) (0.5 mg/L). The pH of the medium was regulated to 7.6. The medium was filtered through sterilized 0.2 μm pore size filters. The samples were kept at 25 ± 1°C and under continuous light conditions (150 μmol photons/m2/s) and gentle stirring (130 rpm). Monoclonal culture was obtained by micromanipulation technique after repeated serial dilutions and consecutive plating on Pm algal growth medium solidified with 1.5% (w/v) agar. Single colonies appearing on plates were selected and subsequently purified with antibiotic treatment to get rid of bacterial contaminants. Routine microscopic examination and repeated streaking on the nutrient agar solid medium were performed periodically to ensure that contamination had not occurred.
2.2. DNA extraction, PCR amplification, sequencing, and taxonomic identification
Genomic DNA was extracted using NucleoSpin® Microbial DNA kit (MACHEREY-NAGEL, Duren, Germany) according to the manufacturer’s recommendations. Three primer sets were used for PCR reaction to amplify the 18S rRNA gene (). PCR was performed using the proofreading Pfu DNA polymerase (Promega®), in a T100 Thermal cycler (Biorad, France) according to the following procedure: denaturation by heating at 94°C for 3 min followed by 30 cycles of denaturation at 94°C for 45 s, annealing at 60°C for 1 min, extension at 72°C for 3 min. A final elongation step of 5 min at 72°C was carried out as the last step. The blunt-end PCR products were cloned into the SmaI site of the plasmid pGEM-7Zf (Promega®), and DNA sequencing reactions were performed using fluorescence-labeled dideoxynucleotide technology (Eurofins Genomics®). Taxonomic identification of this microalga was achieved through BLASTn analysis, comparing the obtained sequences to the sequences available in GenBank Database (https://blast.ncbi.nlm.nih.gov/). A phylogenetic tree was performed with MEGA11 software [Citation35], using Neighbor-Joining method [Citation36]. The evolutionary distances were computed using the p-distance method [Citation37]. The associated taxa were clustered together in the bootstrap test (500 replicates).
Table 1. List of primers used.
2.3. Growth media and culture conditions
Porphyridium sp. was grown axenically in Pm, F/2 [Citation38,Citation39] and Hemerick [Citation40] media previously described in the literature under controlled illumination (150 µmol photons/m2/s), temperature (18°C), and agitation (130 rpm) for approximately 52 days. The F/2 medium was composed per liter as follows: NaCl (27 g), MgCl2,6 H2O (5.6 g), CaCl2 (1.125 g), KNO3 (1 g), KH2PO4 (0.07 g), NaHCO3 (0.04 g), MgSO4,7 H2O (6.6 g), 1 mL NaNO3 (75 g/L), 1 mL NaH2PO4 (5 g/L), 0.5 mL vitamin solution, and 1 mL trace metal solution. The vitamin solution was prepared in MilliQ water containing vitamin B1 (0.2 g/L), 1 mL biotin(1 g/L), and 1 mL vitamin B12 (1 g/L) and sterilized by filtration at 0.2 µm. Trace metal solution was prepared in MilliQ water consisting of Na2EDTA,2 H2O (4.36 g/L), FeCl3,6 H2O (3.15 g/L), 1 mL Na2MoO4,2 H2O (6.3 g/L), 1 mL CuSO4,5 H2O(9.8 g/L), 1 mL ZnSO4,7 H2O (22 g/L), 1 mL CoCl2,6 H2O (10 g/L), and 1 mL MnCl2,4 H2O (180 g/L). The pH of the medium was regulated to 7.2 using 20 mL of Bis-TRIS Buffer (1 M, pH 7.6). Hemerick medium was comprised of NaCl (29 g/L), MgSO4,7 H2O (12.6 g/L), NaNO3 (175 mg/L), CaCl2,2 H2O (0.75 g/L), KCl (1.47 g/L), K2HPO4 (1.7 g/L), Fe-EDTA (0.05 g/L), and 1 mL/L of microelements solution prepared in MilliQ water and containing 2 mg/L H3BO3,1.8 mg/L MnCl2,4 H2O, 0.02 mg/L (NH46Mo7O24,4 H2O, 213 mg/L ZnSO4,7 H2O, 0.08 mg/L CuSO4,5 H2O, 43 mg/L O5SV,5 H2O and 91 mg/L CoSO4. The pH of Hemerick medium was regulated to 7.5. The media were sterilized by autoclaving at 121°C for 20 min. Cells inoculated at 8.2 × 105 cells/mL were maintained in 500 mL sterilized Erlenmeyer glass flask containing 250 mL of culture medium. Every experiment was carried out twice. Daily, 6 mL samples were taken from each biological duplicate. Immediately after sampling, the microalgae growth was followed by counting cells four times using a Malassez’s cell and an inverted microscope (Motic microscope AE 2000, Barcelona, Spain) and by measuring the optical density at 750 nm (OD750) using V-630 spectrophotometer (Jasco, Bouguenais, France). This wavelength was used as absorbance do not interfere with that of pigments [Citation4,Citation41]. The specific growth rate (μ) was calculated in exponential phase using Eq. (1):
where Nt and N0 are the cell concentrations (cells/mL) at time t and t0, respectively.
The doubling time or duplication time (Dt) was determined using Eq. (2):
2.4. Evaluation of nitrate and phosphate consumption
Nitrate uptake was measured on supernatant of culture samples obtained after centrifugation (8000 × g for 10 min at 20°C) using the method of Cawse [Citation42] modified by A.P.H.A. Briefly, 0.4 mL of sample was appropriately diluted and mixed with 3.6 mL of perchloric acid solution (5%). OD210 and OD275 were measured. NaNO3 solutions ranging in concentration from 0 to 0.1 g/L were examined using the same methodology, with the spectrophotometer zero set at 210 nm for a sample lacking NaNO3, and a standard curve established as [NaNO3] = f (OD210). For samples, the optical density reported on the standard curve is corrected using equation (3):
where OD210 and OD275 are raw values of samples at 210 and 275 nm, respectively, and OD275sc is the mean value of optical density at 275 nm for the NaNO3 solutions of the standard curve.
Inorganic phosphate assay was performed in triplicate according to Ames’s method [Citation43]. In an acid medium and in the presence of an excess of ammonium molybdate, the phosphates give a phosphomolybdic complex which, when reduced by reducing agents such as ascorbic acid, develops a molybdenum blue complex. The blue color intensity is proportional to the phosphate amount present in the sample solution [Citation44]. First, the following reagents were prepared as described: (1) Ascorbic acid (10% (m/v), kept at 4°C, (2) 0.42% Ammonium molybdate − 4 H2O in 0.5 M H2SO4 and (3) Mix (10 mL of reagent 1 and 60 mL of reagent 2 prepared just prior to use). Then, 300 µL of supernatant from culture samples (8,000 × g for 10 min at 20°C) and appropriately diluted were mixed with 0.7 mL of the Mix, vortexed and incubated in a water bath at 45°C for 20 min. A standard curve was established using monobasic potassium phosphate (KH2PO4) between 0 and 0.02 g/L following the same protocol and using a stock solution of KH2PO4 at 0.1 g/L. Finally, the OD820 was measured.
2.5. Exopolysaccharide concentration determination
Exopolysaccharides (EPS) content was evaluated by Dubois assay [Citation45]. Assays were performed on desalted cell-free broths obtained after culture sample centrifugation (8,000 × g, 10 min, 20°C) and treatments using Amicon® Ultra 4 mL centrifugal filter fitted with a 10 kDa NMWCO vertical membrane. The supernatant was concentrated three times before being diluted with MilliQ water to the initial volume. This procedure was carried out five times. Each sample was analyzed in triplicate. The calibration curve was made using d-glucose concentrations ranging from 0 to 0.1 g/L.
2.6. Starch content determination
An assay kit (Megazyme, Scotland, United Kingdom) was used to measure the starch content of Porphyridium sp. according to the supplier’s recommendations.
2.7. Pigment content determination
2.7.1. Phycobiliprotein content determination
The extraction of phycobiliproteins was carried out according to the method developed by Coward et al. [Citation46] with appropriate modifications. Briefly, 1 mL of culture was centrifuged at 8,000 × g for 10 min. Pellet was washed for 2 times with the culture medium and centrifuged again at the same speed and then immersed in 1 mL of 0.1 mol/L phosphate buffer (pH 6.0). Phycobiliproteins were extracted from the pellets using repeated freezing/thawing cycles followed by ultrasound treatment until the microalgal residue had no obvious red color. The supernatant was collected by centrifugation (8,000 × g, 10 min) and pigments were spectrophotometrically quantified by measuring the OD650, OD620, and OD565 according to Marcati et al. [Citation47] using the following equations (Eq. (4), Eq. (5), and Eq. (6)):
where APC is the allophycocyanin, R-PC is the phycocyanin, B-PE is the B-phycoerythrin, and OD is the optical density of the pigment at a particular wavelength.
2.7.2. Chlorophyll and carotenoids determination
The concentrations of chlorophyll a, chlorophyll b, and total carotenoids were estimated through spectrophotometry following the methodology previously published by Peña-Medina et al. [Citation48] and Gălan et al. [Citation49]. In brief, 1 mL of culture was centrifuged at 8,000 × g for 10 min. The pellet was resuspended in 1 mL ethanol 95% and treated by ultrasounds. After sonication, the cell debris was removed by centrifugation (8,000 × g for 10 min at room temperature) and pigments were quantified using the equations (7), (8) and (9) as described previously by Lichtenthaler [Citation50]
2.8. Soluble protein determination
The protein content of the ultrasonic-pretreated biomass was evaluated by Lowry assay [Citation51]. In alkaline conditions, proteins react with cupric sulfate and tartrate, leading to the formation of tetradentate copper protein complexes. The Folin – Ciocalteu reagent addition leads to the peptide bond oxidation by forming molybdenum blue with the copper ions. First, the following solutions were prepared in MilliQ water: (1) reagent A (5.18 g/L of sodium hydroxide and 28.62 g/L of sodium carbonate); (2) reagent B (14.232 g/L of copper II sulfate pentahydrate); (3) reagent C (28.60 g/L of potassium sodium tartrate tetrahydrate); (4) Lowry reagent (100 mL of reagent A, 1 mL of reagent B, and 1 mL of reagent C prepared just prior to use). To measure the protein content, 500 μL of the sample were added to 700 μL of Lowry reagent in a glass tube. The resulting mixture was vortexed and incubated for exactly 20 min at room temperature. After incubation, 100 µL of Folin – Ciocalteu Reagent was added, and the sample was vortexed and incubated for 30 min at room temperature in the dark. The calibration was prepared using Bovine Serum Albumin solutions from 0 to 100 mg/L. Finally, the optical density was measured at 750 nm. Each sample was analyzed in triplicate.
2.9. Lipid extraction
Lipid contents of microalgae were analyzed using a method adapted from Bligh and Dyer [Citation52]. Briefly, 200 mg of the freeze-dried biomass were weighed accurately into 15 mL centrifuge polypropylene tubes and were supplemented by 800 µL MilliQ water, 2 mL chloroform, and 1 mL methanol. The mixture was vigorously stirred for 120 s. Thereafter, additional 2 mL of chloroform and 2 mL MilliQ water were added followed by stirring for another 120 s. The suspension was then centrifuged at 8,000 × g for 10 min. The lower organic phase containing extracted lipids was recovered and transferred into tubes previously dried and weighed, while the remaining aqueous phase and also the biomass residues were re-extracted with 2 mL of chloroform for two more times [Citation53]. The chloroform phases were combined together, and the solvent was evaporated in a centrifugal evaporator Speed-Vac (Savant™ SPD111 SpeedVac™, Thermoscientific) at 40°C under a vacuum of 950 mbar, up to weight stabilization. This pellet is resuspended in 200 μL chloroform (for fatty acid analysis). The percentage of the lipid content was determined using Equation ((Eq. (10)):
2.10. Esterification and fatty acid composition analysis
The fatty acid composition analysis was performed using gas-phase chromatography after transformation in methyl esters according to Morrison and Smith’s method [Citation54]. Briefly, 100 µL of extracted lipids (into chloroform) were mixed with 800 µL of a methanol-BF3 solution (14%, p/p) in sealed glass tubes. The mixture was incubated in a dry-bath for 15 min at 100°C before being cooled to room temperature. One point five milliliter of MilliQ water and 0.75 mL of hexane (containing 1% (v/v) of heptadecan as internal standard) were added. Once vortexed for 2 min, the mixture was decanted. The methylated fatty acids were present in the top phase. The used gas chromatography was an Agilent 6850 Series II, equipped with an autosampler G4513A and a flame ionization detector. One microliter of sample was injected. The used column was a polar column HP 88 (112-88A7E) of 100 m × 0.25 mm × 0.2 μm. The oven was set at an initial temperature of 140°C for 5 min followed by a ramp of 4°C/min up to 240°C. Finally, the temperature was maintained at 240°C for 5 min. Nitrogen was used as an inert carrier gas, with the injector and the flame ionization detector temperature fixed at 260°C. Acquisition and data processing were done using ChemStation software. The fatty acid methyl esters were identified and quantified using standards, methylated according to the same methodology (25 injected standards).
2.10. Statistical analysis
To evaluate significant variations between the three tested media, a statistical analysis was performed using one-way analysis of variance (ANOVA) and Tukey’s post-hoc test at a confidence level of 95%. The statistical software employed for this analysis was SPSS 13 (SPSS Ltd., Woking, UK).
3. Results and discussion
3.1. Isolation and identification of the new microalgae strain
The exploration of natural environments could lead to the discovery of new microalgal species and strains with ameliorated or even innovative biotechnological potentials. However, the precise and the consistent taxonomic identification of microalgae newly isolated from any environment is a key to uncovering the possibilities for their future applications.
The importance of native microalgal strains was highlighted from many researchers [Citation55]. In fact, indigenous species demonstrate a better adaptability to environmental conditions prevailing in their original locality, especially in comparison to other commercial microalgal species. In this context, a native marine microalga was isolated in the present study from the coast of Tunisia (governorate of Mahdia), where microalgae diversity is poorly explored, using traditional protocols for isolation. More sophisticated methods, including fluorescence-activated cell sorting and automated single-cell isolation via flow cytometry, could drastically decrease the time needed for the isolation process [Citation56,Citation57]. However, these alternative techniques are costly and technically demanding.
Morphological analysis, using light microscopy, revealed that the isolated strain has spherical and non-flagellated cells with brownish-red color due to the presence of B-phycoerythrin and chlorophyll. As it can be seen in , the microalgal cells are solitary, and the cell size was ranged from 1 to 2 µm. Environmental conditions and their changes may alter the morphology (e.g. cell shape and size) and physiology of microalgae, thus making it hard to distinguish between groups that present very similar features. Therefore, the molecular tools have facilitated the problematic group identification and phylogenies construction, thus eliminating the possibility of erroneous morphological authentication of microalgal identity. In this respect, three regions of the 18S rRNA coding gene from the newly isolated strain were successfully amplified using three primer pairs (). The resulting 528-bp, 721-bp, and 385-bp fragments were sequenced, and sequences have been deposited in the GenBank data library under Accession Number (AE) OM281307 for the 528 bp fragment, OM281309 for the 721-bp sequence, and OM281323 for the 385-pb fragment. The 1602 bp full-length sequence of the 18S locus (Supplementary data S1), obtained from the three overlapping amplified regions, was submitted to BLASTn analysis (https://blast.ncbi.nlm.nih.gov/Blast.cgi), and has been addressed to the 18S rRNA gene of the Porphyridium sp. MBIC10451 strain, with a percentage of coverage and identity of 100%. No genus identity has been assigned to the studied strain, but the analysis of the phylogenetic tree () could suggest that the strain has diverged evolutionarily from Porphyridium purpureum.
Figure 1. Microscopic observation (at 40× magnification) of Porphyridium sp. isolated from the Tunisian coast.

The phylogenetic tree was performed using 24 sequences identified from Blastn analysis and showing the best scores of similarity with the sequence of the newly isolated strain. The percentage of replicate trees in which the associated taxa clustered together in the bootstrap test (500 replicates) is shown next to the branches. The isolated strain is shown surrounded by a rectangle.
3.2. Screening of appropriate medium for Porphyridium sp. growth
Porphyridium sp. was grown in various nutrient medium including Pm, F/2, and Hemerick medium in 500 mL Erlenmeyer flasks each containing 250 mL media. As shown in , the cell density increases during the growth time (52 days) for the three media. The cell concentrations of Porphyridium sp. exhibited significant variations when grown in three different media. It was examined that highest cell concentration of Porphyridium sp. was in Pm (2 × 107 cells/mL), followed by F/2 (1.67 × 107 cells/mL) and Hemerick (1.25 × 107 cells/mL) (p < 0.05). As illustrated in , the growth curve of Porphyridium sp., in all tested media, revealed a latent period of around 3 days of cultivation followed by a log phase reached within 4–6 days. This exponential growth stage continues until reaching the stationary phase within 48 days, for Pm and F/2 media. After that, growth was very sluggish. This can be explained by the high nitrogen content in Pm medium, which promotes the biomass growth increases [Citation58]. In fact, several studies have shown that increasing nitrate concentration may improve the final biomass production of many microalgae species, including Botryococcus braunii [Citation59–63], Porphyridium sp. [Citation64], and Isochrysis galbana [Citation65]. However, when nitrogen is limited, low cell density was found in the Hemerick medium. It was reported by Ji et al. [Citation66] that nitrogen deficiency adversely affected the configuration of chromophores and phycobilisomes, resulting in reduced photosynthetic efficiency and consequent hindrance to the growth of Porphyridium purpureum.
Figure 3. Screening of the most suitable culture medium for Porphyridium sp. growth. Pm medium (○), F/2 medium (∆) and Hemerick medium (□). Data are expressed as mean value ± standard deviation.

The apparent growth rates (µ) were calculated between the 10th and 27th days and were about 0.081 day−1, 0.072 day−1, and 0.043 day−1, and the doubling time (Dt) was of 12.34 days, 13.89 days, and 23.81 days for Pm, F/2, and Hemerick medium, respectively. Previous investigations showed that the growth rates of Porphyridium cruentum ranged between 0.05 and 0.20 day−1 [Citation67] and 0.14 and 0.22 day−1 for Porphyridium purpureum depending on culture conditions, whereas those of Porphyridium marinum were published between 0.54 and 0.56 day−1 [Citation34]. Recently, a research on Porphyridium purpureum and its comparison with Porphyridium sordidum has revealed apparent µmax at 0.22 and 0.17 day−1, respectively [Citation17]. Drira et al. [Citation16] found an average growth rate and a doubling time of 0.055 day−1 and 12.6 days, respectively, for Porphyridium sordidum cultured in Pm medium. However, because these parameters are not usually computed and because it is highly influenced by culture conditions as salinity and light availability, it is exceedingly challenging to compare results from one study to another [Citation68]. The growth rate of Porphyridium sp. was also followed by spectrophotometry (OD750) for 52 days (). According to previous investigation, the best wavelength for measuring cell growth in Porphyridium sordidum and Porphyridium purpureum was confirmed to be OD750 for both Porphyridium strains [Citation17], which was also previously reported to be utilized for Porphyridium purpureum [Citation69]. The optical density curves were quite similar to the trend observed in cell density profiles for all media. The optical density of Porphyridium sp. showed considerable differences when cultivated in three different media. The OD750 confirmed that the best growth of Porphyridium sp. was obtained in Pm medium(p < 0.05). Initial average OD750 of the culture was 0.04. The OD value increased gradually and reached a final average OD750 of 2.17. Thus, the OD value has been increased by approximately 55-fold by the end of culture time. Cell density does correlate well with OD750 for different culture media (data not shown). According to Medina-Cabrera et al. [Citation17], it was possible to compare the growth behavior of Porphyridium sordidum and Porphyridium purpureum indirectly by using the OD750 measurements. Results showed that the difference in the OD750 detected between the two Rhodophyta strains was mediated by the different cell sizes.
3.3. Nutrient uptake by Porphyridium sp.
The nutrient uptake was investigated for a detailed evaluation of microalgal growth characteristics. Nitrate was quantified as it is an essential nutrient for cell proliferation and protein production of microalgae [Citation70]. It is well known that low nitrate amounts favor the shift to the stationary phase, thereby inducing the production of EPS as a response to starvation [Citation71,Citation72]. , b, and c showed that only the Hemerick medium was fully utilized on day 41 of the experiment. The nitrate uptake was 0.11 ± 0.02 and 0.10 ± 0.03 mM per day for Pm and F/2 medium, respectively. Recently, it has been reported by Medina-Cabrera et al. [Citation17] that for Porphyridium purpureum and Porphyridium sordidum the nitrate uptakes were 0.22 mM/day and 0.06 mM/day, respectively. After 52 days of cultivation, Porphyridium sp. grown in Pm and F/2 metabolized 30 ± 0.57% and 46.47 ± 0.10% of the total nitrate, respectively. In the previous investigation, Porphyridium purpureum and Porphyridium sordidum metabolized, respectively, 68% and 17% of the total nitrate after 30 days of cultivation process [Citation17]. Thus, authors concluded that the nitrate consumption impacts Porphyridium purpureum growth much more than for Porphyridium sordidum owing to its higher uptake.
Figure 4. Nutrients uptake nitrate in Pm (a), F/2 (b) and Hemerick (c) and phosphate in Pm (d), F/2 (e) and Hemerick (f) for Porphyridium sp. over a 52 days cultivation process. Data are expressed as mean value ± standard deviation.

Although it presents only 1% of the total microalgal biomass, phosphorus is also an important nutrient for microalgae growth and the first limiting factor affecting their cultivation [Citation73]. Indeed, it enters in numerous cellular processes such as biosynthesis of nucleic acids, phospholipids, proteins, and pigments. Thus, phosphate uptake was also assessed as its starvation is closely related to a decline in generation of microalgal cells. In this respect, it was observed that in the first 44 days, phosphate was consumed by Porphyridium sp. cultivated in Pm medium, reaching a final concentration of 0.21 ± 0.01 mM. At the end of the cultivation process (day 52) 96.04 ± 0.13% of phosphate was consumed (). Porphyridium sp. consumed 0.008 mM/day of phosphate available in F/2 in the first 34 days (). For Porphyridium sp. grown in Hemerick medium, phosphate uptake was observed until the end of the cultivation process (35.51 ± 0.69%) (). It was observed by Medina-Cabrera et al. [Citation17] that in the first 10 days of cultivation process phosphate was consumed by Porphyridium purpureum and Porphyridium sordidum reaching 0.04 mM/day and 0.02 mM/day, respectively. After that, no significant phosphate consumption was detected till the end of the cultivation process.
3.4. Growth medium screening for polysaccharide production
Since the screening of the culture medium is a critical step to assess the capacity of microalgae to produce extracellular polysaccharides, kinetics of EPS production by Porphyridium sp. was also investigated with Pm, F/2, and Hemerick media. For all of the studied media, the EPS concentration rose continuously during the cultivation process. Overall, during the entire experiment, Porphyridium sp. produced EPS in each of the three media with a similar tendency (). In Hemerick medium, EPS production was at its highest level (p < 0.05). After 52 days, EPS concentration increased from 0.56 mg/L to 62.51 mg/L. The cultivation of Porphyridium sp. in both Pm and F/2 media resulted in comparable levels of EPS production, indicating no significant difference between the two tested media (p > 0.05). The maximum EPS production was about 5.41 ± 0.56 pg/cell in Hemerick medium reached on day 44. As previously mentioned, Pm (1.70 g/L of NaNO3) and F/2 (1 g/L of KNO3 and 1 mL of NaNO3 (75 g/L)) have much nitrate than the Hemerick medium (175 mg/L), and the lesser nitrogen concentration supplied by Hemerick medium could be directly responsible for the higher extracellular polysaccharides production by Porphyridium sp. All growth stages showed extracellular polysaccharides generation, with the highest production rates found at the end of the exponential growth phase and during the stationary period. Several microalgae have been reported to produce EPS throughout their stationary development period as a result of the depletion of various nutrients, like phosphorus and nitrogen, including Botryococcus braunii and Chlamydomonas mexicana [Citation74,Citation75]. Furthermore, in nitrogen deficiency conditions, red microalgae, such as Flintiella sanguinaria, Rhodella Violacea and Porphyridium marinum, accumulate extracellular polysaccharides [Citation76–79]. Besides nitrogen concentration, N/P molar ratio was also considered an important factor influencing the production of EPS. The calculation of N/P ratio for Hemerick medium gave 0.22, highlighting a nitrate starvation condition of the growth medium. Redfield [Citation80] suggested an N/P ratio of 16 for an ’equilibrated’ culture medium, where P and N will be depleted at the same time till ideally both are limiting simultaneously, resulting in great cell growth as well as high production of EPS. Soanen and colleagues [Citation78] evaluated the impact of a broad range of N/P ratios on EPS biosynthesis by Porphyridium marinum. The results demonstrated that the drop in N/P ratio (from 24.50 to 3.97) significantly enhanced the specific EPS productivity from 0.05 to 0.062 mg/106 cells. These findings are in accordance with another study of Razaghi et al. [Citation67], in which carbohydrate production by Porphyridium cruentum was conducted with various N/P ratios (50, 35, and 4.90). Low N/P ratio of 4.90 was shown to increase the accumulation of carbohydrates, whereas high N/P ratios of 35 and 50 (phosphorous limitation) promoted biomass production. It should be mentioned that several methods were employed to increase EPS synthesis and productivity in the genus Porphyridium. For instance, a renewal regime of culture allowed a maximum output rate of polysaccharide about 68.64 mg/L/day from Porphyridium cruentum grown in semi-continuously flat plate photobioreactor. Moreover, light quality was also studied by You and Barnett [Citation81] as a key factor for controlling the growth and polysaccharide production in Porphyridium cruentum. The researchers argued that blue light (400–500 nm) may be used to enhance the growth of cells and the production of polysaccharides reaching 4.63 g/L. Furthermore, Liqin and coworkers [Citation82] reported that natural light, green light, and yellow light are more appropriate for increasing the yield of extracellular polysaccharide production by Porphyridium cruentum than blue light and red light, whereas no significant difference in cell growth was observed for various spectra.
Figure 5. (a) kinetics of exopolysaccharides production during cultures of Porphyridium sp. with Pm medium (○), F/2 medium (□) and Hemerick medium (∆); (b) comparison between Hemerick, F/2 and Pm media in the production of starch (day 52). Data are expressed as mean value ± standard deviation. Different low case letters (a and b) present statistically significant differences (p < 0.05), according to one-way analysis of variance and Tukey’s post-hoc test.

At the end of the cultivation period, cultures were centrifuged to recover the biomass which was then lyophilized. Starch production by Porphyridium sp. was evaluated during the 52 days’ cultivation. As shown in , the descending order of starch production (day 52) for the three media was F/2 medium (g/100 g DW): 0.69 ± 0.016; Hemerick medium (g/100 g DW): 0.62 ± 0.050; Pm medium (g/100 g DW): 0.39 ± 0.040. Cultivating Porphyridium sp. in both F/2 and Hemerick media yielded similar levels of starch production, suggesting no significant difference between the two tested media (p > 0.05). Sheath et al. [Citation83] reported that a starch accumulation during the declining growth phase of Porphyridium purpureum in contrast to the findings of Ramus & Robins [Citation84] and Ramus [Citation23] who demonstrated that Porphyridium aerugineum begun active starch accumulation midway through the exponential phase. This dissimilarity may be due to possibly different culture conditions employed in each investigation or species differences. Furthermore, there was a significant difference in the cellular starch amounts of these two species. The authors found a maximum starch content of approximately 4 pg starch/cell [Citation83] and 0.6 pg starch/cell [Citation84] for Porphyridium purpureum and Porphyridium aerugineum, respectively. Under high light intensity (4300 lx), Porphyridium purpureum was shown to accumulate starch granules substantially more than under low light (430 lx) [Citation85]. Similar findings with other microalgae species showed that the starch concentration increased with mean light intensity [Citation86]. The results of an investigation by Ben Hlima et al. [Citation34] into the effects of NaCl content in the growing medium on Porphyridium marinum starch synthesis revealed that the accumulation of starch increased with decreasing salinity. The effect of nitrate concentrations in Pm growth medium (0.30–1.70 g/L) on starch biosynthesis was also studied in the same investigation. Results showed that nitrate content in culture medium does not affect the starch accumulation in microalgal cells. Nitrogen depletion in the growth medium, on the other hand, has been shown by Brányiková et al. [Citation87] to boost starch accumulation in Tetraselmis subcordiformis during the early stage of culture process. Nitrogen limitation was also used for enhancing starch production for other microalgae, such as Chlamydomonas reinhardtii [Citation86], Chlorella sp. [Citation86], and Chlamydomonas sp. [Citation88]. A previous study on Tetraselmis sp. has demonstrated that reducing phosphorus concentration enhances starch production [Citation89]. Specifically, starch biosynthesis in numerous plants and microalgae involves the conversion of ATP and glucose-1-phosphate to pyrophosphate and ADP glucose, a reaction catalyzed by ADP-glucose pyrophosphorylase (AGPase) [Citation90]. It is noteworthy that this enzyme is inhibited by free orthophosphate (Pi) but activated by the 3-phosphoglyceric acid (3-PGA) molecule. Consequently, the 3-PGA/Pi ratio plays a regulatory role in AGPase activity [Citation91]. Elevated photosynthetic activity contributes to increased 3-PGA production, fostering a higher 3-PGA/Pi ratio crucial for AGPase activation and, consequently, more efficient starch synthesis [Citation92].
3.5. Growth medium screening for pigment production
Pm, F/2, and Hemerick medium were also tested to select the most appropriate medium for pigment production. Kinetics of fat liposoluble pigments (Chlorophyll a, Chlorophyll b, and carotenoids) and phycobiliproteins (PE, R-PC, and APC) productions with the three inorganic media are given in the , respectively. Under photoautotrophic conditions, the amounts of chlorophyll a, carotenoids, PE, APC, and R-PC in the cells of Porphyridium sp. grown in Pm and F/2 started to increase from approximately the 8th day until the end of the cultivation time.
Figure 6. Chlorophyll a (○), chlorophyll b (□) and total carotenoids (∆) concentrations in cells of Porphyridium sp. grown at various growth media (a) Pm medium; (b) F/2 medium; (c) Hemerick medium. Data are expressed as mean value ± standard deviation.

Figure 7. R-PC (○), APC (□) and PE (∆) concentrations in Porphyridium sp. cells grown at (a) Pm medium; (b) F/2 medium; (c) Hemerick medium. Data are expressed as mean value ± standard deviation.

Culture with Hemerick medium showed an increase of chlorophyll a from the beginning of cultivation time reaching the highest chlorophyll a content of 0.49 ± 0.0010 µg/mL on day 31, and then chlorophyll a concentration did not increase anymore (). The obtained findings, summarized in , showed that Porphyridium sp. cultivated in Hemerick medium reached the highest PE content of 0.0063 ± 0.0001 mg/mL at the end of the experiment. Carotenoids and R-PC contents increased slowly during the cultivation time leading to final carotenoids and R-PC concentrations of 0.22 ± 0.012 µg/mL and 0.0018 ± 0.0002 mg/mL, respectively. On the other hand, the amounts of APC and chlorophyll b did not show any clear changes in all experiments. In our study, the highest phycoerythrin, chlorophyll a and total carotenoid concentrations were observed in F/2 medium (Chlorophyll a: 8.10 ± 0.10 µg/mL; Total carotenoid: 2.23 ± 0.026 µg/mL; PE: 0.046 ± 0.003 mg/mL). It should be noted that Porphyridium sp. grown in Pm, F/2, and Hemerick media were shown to contain mainly PE. Since the total amount of pigments could differ depending on the extent of biomass such as the number of cells, pigment content per unit of cell was further investigated. As with the previous findings, the highest chlorophyll a (0.678 ± 0.005 pg/cell), total carotenoids (0.18 ± 0.003 pg/cell), and PE (3.88 ± 0.003 pg/cell) content per cell were also observed in F/2 medium on day 34.
The photosynthetic pigments are crucial for gathering photons and transferring absorbed light to the photosynthetic reaction centers [Citation93], and their rate depends directly on photosynthetic efficiency [Citation94]. Various growth media with different chemical compositions have been used for culturing microalgae depending on the microalgal strains used in the cultivation process. In fact, the various mineral components included in microalgal culture medium may influence the growth as well as the metabolism of these photosynthetic microorganisms [Citation95]. Thus, optimizing growth medium and environmental culture conditions are of major importance for enhancing microalgal growth, as well as for photosynthetic pigment synthesis. After being exported to the cytoplasm, one of the two molecules of glutamate produced as a result of the microalgae’s nitrate uptake enters the biosynthetic route for phycobiliproteins. According to previous studies [Citation96–98], in nitrogen depletion conditions, the concentration of photosynthetic pigments in microalgal cells, including phycobiliproteins and chlorophyll, was decreased. Rhodomonas sp. does not immediately adjust to nitrogen deficiency, and for the first few days, the pigment content does not change. Phycoerythrin and chlorophyll concentrations decreased by 90% and 50%, respectively, after 6 days of nitrogen deficiency [Citation99]. According to Gargouch et al. [Citation100], increasing the nitrate content in the growth medium has improved the B-PE accumulation in Porphyridium marinum, while, phosphorus-deficient growth medium stimulates accumulation of the B-PE. This result was consistent with a study conducted by Kathiresan and colleagues [Citation101] that focused on the variables influencing Porphyridium purpureum‘s accumulation of B-PE. These researchers revealed that B-PE accumulation peaked at low phosphorus levels, pointing to phosphorus’ critical function in the biosynthesis of proteins like phycobiliproteins. As previously described, these phycobiliproteins are used by microalgal cells as a source of nitrogen, and some component depletion in the growth medium, like phosphorus, can encourage their accumulation if nitrogen is available. This conclusion was supported by comparing the growth media compositions employed in this investigation. In fact, for Porphyridium sp., B-PE accumulation rate was enhanced when microalga was maintained in F/2 medium compared to the cells cultivated in Pm and Hemerick media, which can be due to the high nitrate content and phosphate deficit in F/2 medium. In addition, it has been reported that increasing the concentration of the metal solution in the growth medium enhances the accumulation of B-PE [Citation100]. In fact, increasing metal ions enhances nitrogen absorption, which promotes cell development [Citation102], leading then to B-PE accumulation. Moreover, trace elements have a major importance in all biological systems since they have a catalytic role as co-factors [Citation103]. The biosynthesis of the co-factor heme, which is regarded as a crucial molecule in the bilin production pathway, is catalyzed by the presence of metal ions in an appropriate proportion [Citation95].
In microalgae, carotenoids constitute the crucial metabolites of the photosynthetic tissues, which are involved in light absorption and in the defense against oxidative stress and photooxidative damage [Citation104]. The relationship between chlorophyll and total carotenoids was computed by the carotenoid-to-chlorophyll ratio. The ratio of carotenoids to chlorophyll is a sensitive marker for distinguishing photooxidative damage and environmental stress in various cultures [Citation105]. If the culture exhibits poor cell development or is subjected to oxidative stress, such as illumination or nutritional limitation, the amount of carotenoids increases, resulting in a high carotenoid-to-chlorophyll ratio. In Hemerick medium, the nitrate was fully used by microalgal cells on day 44 of the cultivation period. Once nitrogen is depleted, it leads to a decrease in the photosynthetic rate. Therefore, the highest carotenoid-to-chlorophyll ratio of 0.4 on day 52 was observed in Hemerick medium.
On day 44 of cultivation in Hemerick medium, the nitrate was fully used by microalgal cells. This nitrogen depletion leads to a decrease in the photosynthetic rate. Therefore, the highest carotenoid-to-chlorophyll ratio of 0.4 on day 52 was observed in Hemerick medium.
3.6. Growth medium screening for soluble protein production
The soluble protein production by Porphyridium sp. in standard culture conditions was investigated. In all three media, the protein content increased continuously until the end of the culture period as illustrated in . The lowest protein content was obtained in Hemerick medium (0.062 ± 0.001 g/L). There was no significant difference in total protein content of Porphyridium sp. between the F/2 and Pm media (p > 0.05). When the soluble protein content was calculated on a per cell basis (), the maximum protein content was detected after 16 days of culture in F/2 medium reaching 14.58 ± 0.35 pg/cell. It was observed by Ben Hlima et al. [Citation34] that optimal conditions for maximal protein production by the marine red microalga Porphyridium marinum could be reached when the nitrogen concentration in the culture medium was moderate (1.2 g/L), its salinity was high (40 g/L) and light intensity around 190 μmol photons/m [Citation2]/s. The decline of chlorophyll and protein contents is well described in the literature as a general response to nitrogen depletion in green microalgae, including Chlorella minutissima, Chlamydomonas reinhardtii, and Chlorella zofingiensis [Citation106–109]. There is common understanding that nitrogen-rich molecules are broken and utilized as nitrogen cell reservoir to enable time-restricted growth processes when nitrogen is unavailable in the growth medium [Citation109,Citation110]. Under unfavorable environmental conditions or nutrient deprivation during their growth, many microalgae repress protein biosynthesis and redirect their metabolism toward hyper-accumulation of EPS or intracellular carbohydrates and lipids [Citation111].
3.7. Growth medium screening for lipid production
The effect of growth medium composition on lipid production by Porphyridium sp. was investigated by testing three different culture media. Comparing the lipid content produced by Porphyridium sp. shown in , it can be seen that the highest lipid content on day 52 was obtained in Hemerick medium (2.23% Dry weight). In agreement with the present findings, many studies have also noted that relatively low nitrogen amounts could be advantageous in enhancing lipid productivity in certain microalgae species [Citation112–116]. Also, Ji et al. [Citation66] reported that when subjected to nitrogen stress, Porphyridium purpureum exhibited a 65.2% increase in total lipid content. Nitrogen deficiency in microalgae has been documented to result in the enhanced activation of the pentose phosphate pathway, facilitating the provision of reducing power NADPH crucial for the biosynthesis of free fatty acids [Citation66,Citation116,Citation117]. In previous investigations involving Porphyridium cruentum [Citation118], lipid levels were measured between 9% and 14% per dry weight. However, in the study of Asgharpour et al. [Citation119], the lipid amounts of the Porphyridium cruentum cells were substantially lower (0.5%–1.6%). This is possibly due to differences in strains or growth medium compositions. As presented in , the major fatty acids from Porphyridium sp. on day 52 were C16:0 (palmitic acid; 69.63%–74.78%), C16:1 (palmitoleic acid; 6.16%–8.38%), C18:0 (stearic acid; 3.48%–7.79%), C18:2 (linoleic acid; 8.33%–13.33%), and C20:4 (ARA; 3.81%–8.88%). In addition, it contained low levels of C18:1-Cis-9 (oleic acid; 0%–1.29%), and cis-22:2n-6 (13,16-docosadienoic acid; 0.22%–0.28%). This result is attributed to the absence of the stearoyl acyl-carrier-protein desaturase enzyme in the plastid stroma of Porphyridium cells [Citation120–122]. This absence leads to the production of palmitic and stearic acids during lipid biosynthesis, serving as precursors for arachidonic and eicosapentaenoic acids. Based on the fact that the Porphyridium sp. was rich in palmitic acid, it is possible to investigate using it to make cosmetology products. In fact, palmitic acid helps to hydrate the skin and acts as a cleansing agent [Citation123]. It has been reported by Shanab et al. [Citation124] that the principal fatty acids detected in most of Porphyridium family include palmitoleic, palmitic, stearic, linoleic, oleic, arachidonic (ARA, C20:4, ω-6), and eicosapentaenoic acids (EPA, C20:5, ω-3), which can act as natural antifreeze and immune-suppressants. The lipid level and fatty acid composition of Rhodophyta genera Porphyridium are diversified depending on various factors. Nitrogen depletion is considered the most efficient strategy to enhance the level of neutral lipids in microalgae, predominantly constituted by the triglycerides with high saturation degree [Citation125,Citation126]. Kavitha et al. [Citation18] showed that a moderate increase in nitrate, chloride, phosphate, and sulfate amounts favored optimal production of biomass, total lipid production (17.90% w/w), and EPA content (34.60% w/w) of Porphyridium purpureum.
Table 2. Lipid content (% dry weight) in cells of Porphyridium sp. on day 52 grown at various growth media.
Table 3. Fatty acid compositions on day 52 of Porphyridium sp. strain (% of total fatty acids) grown in different growth medium.
4. Conclusion
An isolated microalga strain from the Tunisian Coast was identified as belonging to the Porphyridium genus based on morphological identification and 18S rRNA gene sequencing. The effect of three culture media on growth and metabolite production by Porphyridium sp. was investigated. Porphyridum sp. demonstrated marked differences in growth and bioactive compound accumulation in the three tested culture media. Pm medium favors the growth of Porphyridium sp., whereas F/2 medium supports the production of pigments and proteins. The EPS concentration and lipid content of Porphyridium sp. cultured with the Hemerick medium are the highest compared with other media. To conclude, Porphyridium sp. was of high importance as producer of valuable compounds like proteins, carotenoids, phycoerythrin, chlorophyll, and carbohydrate polymers. Under light of the findings obtained in the current study, the next steps will be related to the optimization of up-scaling culture process of the newly isolated microalga to explore its several valuable compounds in innovative applications.
Author contribution
Latifa Tounsi: Investigation, Validation, Writing – original draft, Writing – review & editing. Hajer Ben Hlima: Conceptualization, Validation, Resources, Data curation, Writing – original draft preparation, Writing – Reviewing and Editing. Hana Derbel: Formal analysis, Data curation, Methodology. David Duchez: Formal analysis, Data curation, Methodology. Christine Gadarin: Formal analysis, Data curation, Methodology. Pascal Dubessay: Formal analysis, Data curation, Writing – original draft. Marwa Drira: Formal analysis, Data curation, Writing-original draft. Imen Fendri: Conceptualization, Validation, Review & editing. Philippe Michaud: Conceptualization, Supervision, Resources, Funding acquisition, Writing – Reviewing and Editing. Slim Abdelkafi: Conceptualization, Supervision, Resources, Funding acquisition, Writing – Reviewing and Editing.
Supplemental Material
Download MS Word (283.8 KB)Acknowledgments
The authors of this work thank the Tunisian Ministry of higher education and scientific research for providing the funding for this research.
Disclosure statement
No potential conflict of interest was reported by the author(s).
Data availability statement
The data presented in this study are available on request from the corresponding author. The data are not publicly available due to privacy restrictions.
Supplementary material
Supplemental data for this article can be accessed online at https://doi.org/10.1080/21655979.2023.2294160.
Additional information
Funding
References
- Ruiz J, Olivieri G, de Vree J, Bosma R, Willems P, Reith JH, Eppink MHM, Kleinegris DMM, Wijffels RH, Barbosa MJ. Towards industrial products from microalgae. Energy & Environmental Science 2016; 9:3036–23.
- D’Alessandro EB, Antoniosi Filho NR. Concepts and studies on lipid and pigments of microalgae: A review. Renewable and Sustainable Energy Reviews 2016; 58:832–41.
- Elleuch F, Ben Hlima H, Barkallah M, Baril P, Abdelkafi S, Pichon C, Fendri I Carotenoids overproduction in Dunaliella sp.: Transcriptional changes and new insights through lycopene cyclase regulation. Applied Sciences – Switzerland 2019; 9(24): 5389.
- Tounsi L, Hentati F, Ben Hlima H, Barkallah M, Smaoui S, Fendri I, Philippe M, Abdelkafi S. Microalgae as feedstock for bioactive polysaccharides. International Journal of Biological Macromolecules 2022; 221:1238–1250.
- Gargouch N, Elleuch F, Karkouch I, Tabbene O, Pichon C, Gardarin C, Rihouey C, Picton L, Abdelkafi S, Fendri I, et al. Potential of Exopolysaccharide from Porphyridium marinum to Contend with Bacterial Proliferation, Biofilm Formation, and Breast Cancer. Marine Drugs 2021; 19:66.
- Raposo MF de J, de Morais AMMB, de Morais RMSC. Influence of sulphate on the composition and antibacterial and antiviral properties of the exopolysaccharide from Porphyridium cruentum. Life Sciences 2014; 101:56–63.
- Tannin-Spitz T, Bergman M, van-Moppes D, Grossman S, Arad S (Malis). Antioxidant activity of the polysaccharide of the red microalga Porphyridium sp. Journal of Applied Phycology 2005; 17:215–22.
- Trinquet E, Maurin F, Préaudat M, Mathis G. Allophycocyanin 1 as a Near-Infrared Fluorescent Tracer: Isolation, Characterization, Chemical Modification, and Use in a Homogeneous Fluorescence Resonance Energy Transfer System. Analytical Biochemistry 2001; 296:232–44.
- Waggoner A. Fluorescent labels for proteomics and genomics. Current Opinion in Chemical Biology 2006; 10:62–6.
- Tounsi L, Ben Hlima H, Hentati F, Hentati O, Derbel H, Michaud P, Abdelkafi S. Microalgae: A Promising Source of Bioactive Phycobiliproteins. Marine Drugs 2023; 21:440.
- Lauceri R, Chini Zittelli G, Torzillo G. A simple method for rapid purification of 662 phycobiliproteins from Arthrospira platensis and Porphyridium cruentum biomass. Algal Research 2019; 44:101685.
- Rebolloso Fuentes MM, Acién Fernández GG, Sánchez Pérez JA, Guil Guerrero JL. Biomass nutrient profiles of the microalga Porphyridium cruentum. Food Chemistry 2000; 70:345–53.
- Assunção MFG, Varejão JMTB, Santos LMA. Nutritional characterization of the microalga Ruttnera lamellosa compared to Porphyridium purpureum. Algal Research 2017; 26:8–14.
- Guihéneuf F, Stengel DB. Towards the biorefinery concept: Interaction of light, temperature and nitrogen for optimizing the co-production of high-value compounds in Porphyridium purpureum. Algal Research 2015; 10:152–63.
- Li T, Xu J, Wu H, Jiang P, Chen Z, Xiang W. Growth and Biochemical Composition of Porphyridium purpureum SCS-02 under Different Nitrogen Concentrations. Marine Drugs 2019; 17:124.
- Drira M, Elleuch J, Ben Hlima H, Hentati F, Gardarin C, Rihouey C, Le Cerf D, Michaud P, Abdelkafi S, Fendri I. Optimization of Exopolysaccharides Production by Porphyridium sordidum and Their Potential to Induce Defense Responses in Arabidopsis thaliana against Fusarium oxysporum. Biomolecules 2021; 11:282.
- Medina-Cabrera EV, Rühmann B, Schmid J, Sieber V. Characterization 678 and comparison of Porphyridium sordidum and Porphyridium purpureum concerning growth characteristics and polysaccharide production. Algal Research 2020; 49:101931.
- Kavitha MD, Kathiresan S, Bhattacharya S, Sarada R. Culture media optimization of Porphyridium purpureum: production potential of biomass, total lipids, arachidonic and eicosapentaenoic acid .Journal of Food Science and Technology 2016; 53:2270–8.
- Safi C, Charton M, Pignolet O, Pontalier P-Y, Vaca-Garcia C. Evaluation of the protein quality of Porphyridium cruentum. Journal of Applied Phycology 2013; 25:497–501.
- Lutzu GA, Zhang L, Zhang Z, Liu T. Feasibility of attached cultivation for polysaccharides production by Porphyridium cruentum. Bioprocess and Biosystems Engineering 2017; 40:73–83.
- Tounsi L, Ben Hlima H, Fendri I, Abdelkafi S, Michaud P. Photoautotrophic growth and accumulation of macromolecules by Porphyridium cruentum UTEX 161 depending on culture media. Biomass Conversion and Biorefinery 2023; 1–18
- Netanel Liberman G, Ochbaum G, Mejubovsky-Mikhelis M, Bitton R, (Malis) Arad S. Physico chemical characteristics of the sulfated polysaccharides of the red microalgae Dixoniella grisea and Porphyridium aerugineum. International Journal of Biological Macromolecules 2020; 145:1171–9.
- Ramus J. The production of extracellular polysaccharide by the unicellular red alga Porphyridium aerugineum. Journal of Phycology 1972; 8:97–111.
- Gong X, Chen F. Optimization of culture medium for growth of Haematococcus pluvialis. J Appl Phycol. 1997;9(5):437–444. doi: 10.1023/A:1007944922264
- Ben Amor F, Elleuch F, Ben Hlima H, et al. Proteomic analysis of the Chlorophyta Dunaliella new strain AL-1 revealed global changes of metabolism during high carotenoid production. Mar Drugs. 2017;15(9):293. doi: 10.3390/md15090293
- Chakraborty B, Gayen K, Bhowmick TK. Transition from synthetic to alternative media for microalgae cultivation: a critical review. Sci Total Environ. 2023;897:165412. doi: 10.1016/j.scitotenv.2023.165412
- Daneshvar E, Ok YS, Tavakoli S, et al. Insights into upstream processing of microalgae: a review. Biores Technol. 2021;329:124870. doi: 10.1016/j.biortech.2021.124870
- Zhu L, Wang Z, Shu Q, et al. Nutrient removal and biodiesel production by integration of freshwater algae cultivation with piggery wastewater treatment. Water Res. 2013;47(13):4294–4302. doi: 10.1016/j.watres.2013.05.004
- Oyebamiji OO, Boeing WJ, Holguin FO, et al. Green microalgae cultured in textile wastewater for biomass generation and biodetoxification of heavy metals and chromogenic substances. Bioresour Technol Rep. 2019;7:100247. doi: 10.1016/j.biteb.2019.100247
- Mohd Udaiyappan AF, Abu Hasan H, Takriff MS, et al. A review of the potentials, challenges and current status of microalgae biomass applications in industrial wastewater treatment. Water Proc Eng. 2017;20:8–21. doi: 10.1016/j.jwpe.2017.09.006
- Menegat S, Ledo A, Tirado R. Greenhouse gas emissions from global production and use of nitrogen synthetic fertilisers in agriculture. Sci Rep. 2022;12(1):14490. doi: 10.1038/s41598-022-18773-w
- Chai R, Ye X, Ma C, et al. Greenhouse gas emissions from synthetic nitrogen manufacture and fertilization for main upland crops in China. Carbon Balance Manag. 2019;14(1):20. doi: 10.1186/s13021-019-0133-9
- Derbel H, Elleuch J, Tounsi L, et al. Improvement of biomass and phycoerythrin production by a strain of Rhodomonas sp. Isolated from the Tunisian coast of Sidi Mansour. Biomolecules. 2022;12(7):885. doi: 10.3390/biom12070885
- Ben Hlima H, Dammak M, Karkouch N, et al. Optimal cultivation towards enhanced biomass and floridean starch production by Porphyridium marinum. Int j biol macromol. 2019;129:152–161. doi: 10.1016/j.ijbiomac.2019.01.207
- Tamura K, Stecher G, Kumar S, et al. MEGA11: Molecular evolutionary genetics analysis Version 11. Mol Biol Evol. 2021;38(7):3022–3027. doi: 10.1093/molbev/msab120
- Saitou N, Nei M. The neighbor-joining method: a new method for reconstructing phylogenetic trees. Mol Biol Evol. 1987;4(4):406–425. doi: 10.1093/oxfordjournals.molbev.a040454
- Abdelkafi S, Labat M, Casalot L, et al. Isolation and characterization of Halomonas sp. strain IMPC, a p-coumaric acid-metabolizing bacterium that decarboxylates other cinnamic acids under hypersaline conditions. FEMS Microbiol Lett. 2006;255:108–114. doi: 10.1111/j.1574-6968.2005.00061.x
- Guillard RRL. Culture of phytoplankton for feeding marine invertebrates. In: Smith W Chanley M, editors Culture of Marine invertebrate animals. Boston, MA: Springer US; 1975. pp. 29–60.
- Guillard RRL, Ryther JH. Studies of marine planktonic diatoms: I. Cyclotella nana hustedt, and Detonula confervacea (cleve) gran. Can J Microbiol. 1962;8(2):229–239. doi: 10.1139/m62-029
- Stein-Taylor JR. Culture methods and growth measurements. Handbook of phycological methods. Cambridge University Press; 1973.
- Griffiths MJ, Garcin C, van Hille RP, et al. Interference by pigment in the estimation of microalgal biomass concentration by optical density. J Microbiol Methods. 2011;85:119–123. doi: 10.1016/j.mimet.2011.02.005
- Cawse PA. The determination of nitrate in soil solutions by ultraviolet spectrophotometry. Analyst. 1967;92(1094):311. doi: 10.1039/an9679200311
- Ames BN. Assay of inorganic phosphate, total phosphate and phosphatases. In: Methods in Enzymology. Vol. 8. Elsevier; 1966. p. 115–118. doi: 10.1016/0076-6879(66)08014-5.
- Pradhan S, Pokhrel MR. Spectrophotometric determination of phosphate in sugarcane juice, fertilizer, detergent and water samples by molybdenum blue method. Sci World [Internet]. 2013;11(11):58–62. doi: 10.3126/sw.v11i11.9139
- Michel D, Gilles KA, Hamilton JK, et al. Colorimetric method for determination of sugars and related substances. Anal Chem. 1956;28(3):350–356. doi: 10.1021/ac60111a017
- Coward T, Fuentes-Grünewald C, Silkina A, et al. Utilising light-emitting diodes of specific narrow wavelengths for the optimization and co-production of multiple high-value compounds in Porphyridium purpureum. Biores Technol. 2016;221:607–615. doi: 10.1016/j.biortech.2016.09.093
- Marcati A, Ursu AV, Laroche C, et al. Extraction and fractionation of polysaccharides and B-phycoerythrin from the microalga Porphyridium cruentum by membrane technology. Algal Res. 2014;5:258–263. doi: 10.1016/j.algal.2014.03.006
- Peña-Medina RL, Fimbres-Olivarría D, Enríquez-Ocaña LF, et al. Erythroprotective potential of phycobiliproteins extracted from Porphyridium cruentum. Metabolites. 2023;13(3):366. doi: 10.3390/metabo13030366
- Gălan A-M, Vlaicu A, Vintilă ACN, et al. Microalgae strain Porphyridium purpureum for nutrient reduction in dairy wastewaters. Sustainability. 2022;14(14):8545. doi: 10.3390/su14148545
- Lichtenthaler HK. Chlorophylls and carotenoids: pigments of photosynthetic biomembranes. In: Methods in Enzymology. Elsevier; 1987. p. 350–382. doi: 10.1016/0076-6879(87)48036-1
- OliverH L, NiraJ R, Farr AL, et al. Protein measurement with the folin phenol reagent. J Biol Chem. 1951;193(1):265–275. doi: 10.1016/S0021-9258(19)52451-6
- Bligh EG, Dyer WJ. A rapid method of total lipid extraction and purification. Can J Biochem Physiol. 1959;37(1):911–917. doi: 10.1139/y59-099
- Abdelkafi S, Fouquet B, Barouh N, et al. In vitro comparisons between Carica papaya and pancreatic lipases during test meal lipolysis: potential use of CPL in enzyme replacement therapy. Food Chem. 2009;115(2):488–494. doi: 10.1016/j.foodchem.2008.12.043
- Morrison WR, Smith LM. Preparation of fatty acid methyl esters and dimethylacetals from lipids with boron fluoride–methanol. J Lipid Res. 1964;5(4):600–608. doi: 10.1016/S0022-2275(20)40190-7
- Hotos G, Avramidou D, Mastropetros SG, et al. Isolation, identification, and chemical composition analysis of nine microalgal and cyanobacterial species isolated in lagoons of Western Greece. Algal Res. 2023;69:102935. doi: 10.1016/j.algal.2022.102935
- Neofotis P, Huang A, Sury K, et al. Characterization and classification of highly productive microalgae strains discovered for biofuel and bioproduct generation. Algal Res. 2016;15:164–178. doi: 10.1016/j.algal.2016.01.007
- Terashima M, Freeman ES, Jinkerson RE, et al. A fluorescence-activated cell sorting-based strategy for rapid isolation of high-lipid chlamydomonas mutants. Plant J. 2015;81(1):147–159. doi: 10.1111/tpj.12682
- Li Z, Ma X, Li A, et al. A novel potential source of β-carotene: eustigmatos cf. polyphem (eustigmatophyceae) and pilot β-carotene production in bubble column and flat panel photobioreactors. Biores Technol. 2012;117:257–263. doi: 10.1016/j.biortech.2012.04.069
- Banerjee A, Sharma R, Chisti Y, et al. Botryococcus braunii: a renewable source of hydrocarbons and other chemicals. Crit Rev Biotechnol. 2002;22(3):245–279. doi: 10.1080/07388550290789513
- Dayananda C, Sarada R, Bhattacharya S, et al. Effect of media and culture conditions on growth and hydrocarbon production by botryococcus braunii. Process Biochem. 2005;40(9):3125–3131. doi: 10.1016/j.procbio.2005.03.006
- Lupi FM, Fernandes HML, Tomé MM, et al. Influence of nitrogen source and photoperiod on exopolysaccharide synthesis by the microalga Botryococcus braunii UC 58. Enzyme Microb Technol. 1994;16(7):546–550. doi: 10.1016/0141-0229(94)90116-3
- Yang S, Wang J, Cong W, et al. Utilization of nitrite as a nitrogen source by Botryococcus Braunii. Biotechnol Lett. 2004;26(3):239–243. doi: 10.1023/B:BILE.0000013722.45527.18
- Ashokkumar V, Rengasamy R. Mass culture of Botryococcus braunii Kutz. under open raceway pond for biofuel production. Biores Technol. 2012;104:394–399. doi: 10.1016/j.biortech.2011.10.093
- Arad S, Friedman O, Rotem A. Effect of nitrogen on polysaccharide production in a Porphyridium sp. Appl environ microbiol. 1988;54(10):2411–2414. doi: 10.1128/aem.54.10.2411-2414.1988
- Martel CM. Nitrogen-deficient microalgae are rich in cell-surface mannose: potential implications for prey biorecognition by phagotrophic protozoa. Braz J Microbiol. 2009;40(1):86–89. doi: 10.1590/S1517-83822009000100014
- Ji L, Li S, Chen C, et al. Physiological and transcriptome analysis elucidates the metabolic mechanism of versatile Porphyridium purpureum under nitrogen deprivation for exopolysaccharides accumulation. Bioresources Bioprocess. 2021;8(1):73. doi: 10.1186/s40643-021-00426-x
- Razaghi A, Godhe A, Albers E. Effects of nitrogen on growth and carbohydrate formation in Porphyridium cruentum. Open Life Sciences. 2014;9(2):156–162. doi: 10.2478/s11535-013-0248-
- Borjas Esqueda A, Gardarin C, Laroche C. Exploring the diversity of red microalgae for exopolysaccharide production. Mar Drugs. 2022;20(4):246. doi: 10.3390/md20040246
- Fleck-Schneider P, Lehr F, Posten C. Modelling of growth and product formation of Porphyridium purpureum. J Biotechnol. 2007;132(2):134–141. doi: 10.1016/j.jbiotec.2007.05.030
- Mostert ES, Grobbelaar JU. The influence of nitrogen and phosphorus on algal growth and quality in outdoor mass algal cultures. Biomass. 1987;13(4):219–233. doi: 10.1016/0144-4565(87)90061-8
- Nicolaus B, Panico A, Lama L, et al. Chemical composition and production of exopolysaccharides from representative members of heterocystous and non-heterocystous cyanobacteria. Phytochemistry. 1999;52(4):639–647. doi: 10.1016/S0031-9422(99)00202-2
- De Philippis R, Margheri MC, Pelosi E, et al. Exopolysaccharide production by a unicellular cyanobacterium isolated from a hypersaline habitat. J Appl Phycol. 1993;5(4):387–394. doi: 10.1007/BF02182731
- Steinberg CEW, Hartmann HM. Planktonic bloom-forming cyanobacteria and the eutrophication of lakes and rivers. Freshwater Biol. 1988;20(2):279–287. doi: 10.1111/j.1365-2427.1988.tb00452.x
- Kroen WK, Rayburn WR. Influence of growth status and nutrients on extracellular polysaccharide synthesis by the soil alga Chlamydomonas Mexicana (chlorophyceae)1. J Phycol. 1984;20(2):253–257. doi: 10.1111/j.0022-3646.1984.00253.x
- Díaz Bayona KC, Garcés LA. Effect of different media on exopolysaccharide and biomass production by the green microalga botryococcus braunii. J Appl Phycol. 2014;26(5):2087–2095. doi: 10.1007/s10811-014-0242-5
- Gaignard C, Macao V, Gardarin C, et al. The red microalga Flintiella sanguinaria as a new exopolysaccharide producer. J Appl Phycol. 2018;30(5):2803–2814. doi: 10.1007/s10811-018-1389-2
- Gaignard C, Laroche C, Pierre G, et al. Screening of marine microalgae: investigation of new exopolysaccharide producers. Algal Res. 2019;44:101711. doi: 10.1016/j.algal.2019.101711
- Soanen N, Da Silva E, Gardarin C, et al. Improvement of exopolysaccharide production by Porphyridium marinum. Biores Technol. 2016;213:231–238. doi: 10.1016/j.biortech.2016.02.075
- Villay A, Laroche C, Roriz D, et al. Optimisation of culture parameters for exopolysaccharides production by the microalga Rhodella violacea. Biores Technol. 2013;146:732–735. doi: 10.1016/j.biortech.2013.07.030
- Redfield AC. The biological control of chemical factors in the environment. Am Scientist. 1958;46:230A–221.
- You T, Barnett SM. Effect of light quality on production of extracellular polysaccharides and growth rate of Porphyridium cruentum. Biochem Eng J. 2004 12;19(3):251–258. doi: 10.1016/j.bej.2004.02.004
- Liqin S, Wang C, Lei S Effects of light regime on extracellular polysaccharide production by Porphyridium cruentum cultured in flat plate photobioreactors. In: 2nd International Conference on Bioinformatics and Biomedical Engineering. 2008. page 1488–1491.
- Sheath RG, Hellebust JA, Sawa T. Floridean starch metabolism of Porphyridium purpureum (Rhodophyta): I. Changes during ageing of batch culture. Phycologia. 1979;18(2):149–163. doi: 10.2216/i0031-8884-18-2-149.1
- Ramus J, Robins DM. THE CORRELATION OF GOLGI ACTIVITY AND POLYSACCHARIDE SECRETION IN Porphyridium 1 2. J Phycol. 1975;11(1):70–74. doi: 10.1111/j.1529-8817.1975.tb02750.x
- Viola R, Nyvall P, Pedersén M. The unique features of starch metabolism in red algae. Proc R Soc Lond B. 2001;268(1474):1417–1422. doi: 10.1098/rspb.2001.1644
- Eriksen NT, Riisgård FK, Gunther WS, et al. On-line estimation of O2 production, CO2 uptake, and growth kinetics of microalgal cultures in a gas-tight photobioreactor. J Appl Phycol. 2007;19(2):161–174. doi: 10.1007/s10811-006-9122-y
- Brányiková I, Maršálková B, Doucha J, et al. Microalgae—novel highly efficient starch producers. Biotechnol Bioeng. 2011;108(4):766–776. doi: 10.1002/bit.23016
- Maeda I, Seto Y, Ueda S, et al. Simultaneous control of turbidity and dilution rate through adjustment of medium composition in semi-continuous Chlamydomonas cultures. Biotechnol Bioeng. 2006;94(4):722–729. doi: 10.1002/bit.20884
- Dammak M, Hadrich B, Miladi R, et al. Effects of nutritional conditions on growth and biochemical composition of tetraselmis sp. Lipids Health Dis. 2017;16(1):41. doi: 10.1186/s12944-016-0378-1
- Ball SG, Morell MK. From bacterial glycogen to starch: understanding the biogenesis of the Plant starch granule. Annu Rev Plant Biol. 2003;54(1):207–233. doi: 10.1146/annurev.arplant.54.031902.134927
- Ball SGRegulation of starch biosynthesisThe molecular biology of chloroplasts and mitochondria in ChlamydomonasIn: Rochaix J-D, Goldschmidt-Clermont M Merchant SeditorsSpringer NetherlandsDordrecht1998,pp. 549–567.
- Yao C-H, Ai J-N, Cao X-P, et al. Characterization of cell growth and starch production in the marine green microalga tetraselmis subcordiformis under extracellular phosphorus-deprived and sequentially phosphorus-replete conditions. Appl Microbiol Biotechnol. 2013;97(13):6099–6110. doi: 10.1007/s00253-013-4983-x
- Kursar TA, Alberte RS. Photosynthetic Unit Organization in a red alga: relationships between light-harvesting pigments and reaction centers. Plant Physiol. 1983;72(2):409–414. doi: 10.1104/pp.72.2.409
- Yokoya NS, Necchi O, Martins AP, et al. Growth responses and photosynthetic characteristics of wild and phycoerythrin-deficient strains of Hypnea musciformis (Rhodophyta). J Appl Phycol. 2007;19(3):197–205. doi: 10.1007/s10811-006-9124-9
- Manirafasha E, Ndikubwimana T, Zeng X, et al. Phycobiliprotein: Potential microalgae derived pharmaceutical and biological reagent. Biochem Eng J. 2016;109:282–296. doi: 10.1016/j.bej.2016.01.025
- Chaloub RM, Motta NMS, de Araujo SP, et al. Combined effects of irradiance, temperature and nitrate concentration on phycoerythrin content in the microalga Rhodomonas sp (cryptophyceae). Algal Research. 2015;8:89–94. doi: 10.1016/j.algal.2015.01.008
- Zhao L-S, Su H-N, Li K, et al. Supramolecular architecture of photosynthetic membrane in red algae in response to nitrogen starvation. Biochim Biophys Acta Bioenerg. 2016;1857(11):1751–1758. doi: 10.1016/j.bbabio.2016.08.005
- Levy I, Gantt E. DEVELOPMENT of PHOTOSYNTHETIC ACTIVITY in Porphyridium purpureum (RHODOPHYTA) FOLLOWING NITROGEN STARVATION 1, 2. J Phycol. 1990;26(1):62–68. doi: 10.1111/j.0022-3646.1990.00062.x
- Dupre C, Guary J-C, Grizeau D. Effect of photon fluence rate, nitrogen limitation and nitrogen recovery on the level of phycoerythrin in the unicellular alga, rhodosorus marinus (rhodophyceae). Physiologia Plantarum. 1994;92(3):521–527. doi: 10.1111/j.1399-3054.1994.tb08846.x
- Gargouch N, Karkouch I, Elleuch J, et al. Enhanced B-phycoerythrin production by the red microalga Porphyridium marinum: a powerful agent in industrial applications. Int j biol macromol. 2018;120:2106–2114. doi: 10.1016/j.ijbiomac.2018.09.037
- Kathiresan S, Sarada R, Bhattacharya S, et al. Culture media optimization for growth and phycoerythrin production from Porphyridium purpureum. Biotechnol Bioeng. 2007;96(3):456–463. doi: 10.1002/bit.21138
- Lin Q, Gu N, Lin J. Effect of ferric ion on nitrogen consumption, biomass and oil accumulation of a Scenedesmus rubescens-like microalga. Biores Technol. 2012;112:242–247. doi: 10.1016/j.biortech.2012.02.097
- Tyihák E, Móricz ÁM. BioArena system for studying key molecules as well as ingredients in biological samples. In: Forced-Flow Layer Chromatography. Elsevier; 2016. pp. 397–485.
- Candan N, Tarhan L. Changes in chlorophyll-carotenoid contents, antioxidant enzyme activities and lipid peroxidation levels in Zn-stressed Mentha pulegium. Turk J Chem. 2003;27(1):21–30.
- Netto AT, Campostrini E, de OJ, et al. Photosynthetic pigments, nitrogen, chlorophyll a fluorescence and SPAD-502 readings in coffee leaves. Sci Hortic. 2005;104(2):199–209. doi: 10.1016/j.scienta.2004.08.013
- Zhu S, Huang W, Xu J, et al. Metabolic changes of starch and lipid triggered by nitrogen starvation in the microalga Chlorella zofingiensis. Biores Technol. 2014;152:292–298. doi: 10.1016/j.biortech.2013.10.092
- Siaut M, Cuiné S, Cagnon C, et al. Oil accumulation in the model green alga Chlamydomonas reinhardtii: characterization, variability between common laboratory strains and relationship with starch reserves. BMC Biotechnol. 2011;11(1):7. doi: 10.1186/1472-6750-11-7
- Msanne J, Xu D, Konda AR, et al. Metabolic and gene expression changes triggered by nitrogen deprivation in the photoautotrophically grown microalgae Chlamydomonas reinhardtii and Coccomyxa sp. C-169. Phytochemistry. 2012;75:50–59. doi: 10.1016/j.phytochem.2011.12.007
- Ördög V, Stirk WA, Bálint P, et al. Changes in lipid, protein and pigment concentrations in nitrogen-stressed Chlorella minutissima cultures. J Appl Phycol. 2012;24(4):907–914. doi: 10.1007/s10811-011-9711-2
- Lv J-M, Cheng L-H, Xu X-H, et al. Enhanced lipid production of chlorella vulgaris by adjustment of cultivation conditions. Biores Technol. 2010;101(17):6797–6804. doi: 10.1016/j.biortech.2010.03.120
- Zachleder V, Brányiková I. Starch overproduction by Means of algae. In: Bajpai R, Prokop A Zappi M, editors Algal biorefineries. Dordrecht: Springer Netherlands; 2014. pp. 217–240.
- Mujtaba G, Choi W, Lee C-G, et al. Lipid production by Chlorella vulgaris after a shift from nutrient-rich to nitrogen starvation conditions. Biores Technol. 2012;123:279–283. doi: 10.1016/j.biortech.2012.07.057
- Rodolfi L, Chini Zittelli G, Bassi N, et al. Microalgae for oil: strain selection, induction of lipid synthesis and outdoor mass cultivation in a low-cost photobioreactor. Biotechnol Bioeng. 2009;102(1):100–112. doi: 10.1002/bit.22033
- Illman AM, Scragg AH, Shales SW. Increase in chlorella strains calorific values when grown in low nitrogen medium. Enzyme Microb Technol. 2000;27(8):631–635. doi: 10.1016/S0141-0229(00)00266-0
- Li J, Han D, Wang D, et al. Choreography of transcriptomes and lipidomes of nannochloropsis reveals the mechanisms of oil synthesis in microalgae. Plant Cell. 2014;26(4):1645–1665. doi: 10.1105/tpc.113.121418
- Liu J, Sun Z, Mao X, et al. Multiomics analysis reveals a distinct mechanism of oleaginousness in the emerging model alga chromochloris zofingiensis. Plant J. 2019;98(6):1060–1077. doi: 10.1111/tpj.14302
- Jaeger D, Winkler A, Mussgnug JH, et al. Time-resolved transcriptome analysis and lipid pathway reconstruction of the oleaginous green microalga monoraphidium neglectum reveal a model for triacylglycerol and lipid hyperaccumulation. Biotechnol Biofuels. 2017;10(1):197. doi: 10.1186/s13068-017-0882-1
- Becker EW. Microalgae: biotechnology and microbiology. Eberhard-Karls-Universität Tübingen, Germany: Cambridge University Press; 1994. p. 293.
- Asgharpour M, Rodgers B, Hestekin JA. Eicosapentaenoic acid from Porphyridium Cruentum: increasing growth and productivity of microalgae for pharmaceutical products. Energies. 2015;8(9):10487–10503. doi: 10.3390/en80910487
- Sato N, Moriyama T, Mori N, et al. Lipid metabolism and potentials of biofuel and high added-value oil production in red algae. World J Microbiol Biotechnol. 2017;33(4):74. doi: 10.1007/s11274-017-2236-3
- Chang J, Le K, Song X, et al. Scale-up cultivation enhanced arachidonic acid accumulation by red microalgae Porphyridium purpureum. Bioprocess Biosyst Eng. 2017;40(12):1763–1773. doi: 10.1007/s00449-017-1831-x
- Bhattacharya D, Price DC, Chan CX, et al. Genome of the red alga Porphyridium purpureum. Nat Commun. 2013;4(1):1941. doi: 10.1038/ncomms2931
- Joshi S, Kumari R, Upasani VN. Applications of algae in cosmetics: an overview. Int J Innov Res Sci Eng Technol. 2018;7:1269–1278. doi: 10.15680/IJIRSET.2018.0702038
- Shanab SMM, Hafez RM, Fouad AS. A review on algae and plants as potential source of arachidonic acid. J Adv Res. 2018;11:3–13. doi: 10.1016/j.jare.2018.03.004
- Liu H, Fang HHP. Extraction of extracellular polymeric substances (EPS) of sludges. J Biotechnol. 2002;95(3):249–256. doi: 10.1016/S0168-1656(02)00025-1
- Ye Y, Clech PL, Chen V, et al. Evolution of fouling during crossflow filtration of model EPS solutions. J Membr Sci. 2005;264(1–2):190–199. doi: 10.1016/j.memsci.2005.04.040