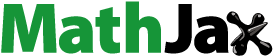
ABSTRACT
Cadmium (Cd) has become a severe issue in relatively low concentration and attracts expert attention due to its toxicity, accumulation, and biomagnification in living organisms. Cd does not have a biological role and causes serious health issues. Therefore, Cd pollutants should be reduced and removed from the environment. Microalgae have great potential for Cd absorption for waste treatment since they are more environmentally friendly than existing treatment methods and have strong metal sorption selectivity. This study evaluated the tolerance and ability of the microalga Tetratostichococcus sp. P1 to remove Cd ions under acidic conditions and reveal mechanisms based on transcriptomics analysis. The results showed that Tetratostichococcus sp. P1 had a high Cd tolerance that survived under the presence of Cd up to 100 µM, and IC50, the half-maximal inhibitory concentration value, was 57.0 μM, calculated from the change in growth rate based on the chlorophyll content. Long-term Cd exposure affected the algal morphology and photosynthetic pigments of the alga. Tetratostichococcus sp. P1 removed Cd with a maximum uptake of 1.55 mg g−1 dry weight. Transcriptomic analysis revealed the upregulation of the expression of genes related to metal binding, such as metallothionein. Group A, Group B transporters and glutathione, were also found upregulated. While the downregulation of the genes were related to photosynthesis, mitochondria electron transport, ABC-2 transporter, polysaccharide metabolic process, and cell division. This research is the first study on heavy metal bioremediation using Tetratostichococcus sp. P1 and provides a new potential microalga strain for heavy metal removal in wastewater.

Abbreviations:BP: Biological process; bZIP: Basic Leucine Zipper; CC: Cellular component; ccc1: Ca (II)-sensitive cross complementary 1; Cd: Cadmium; CDF: Cation diffusion facilitator; Chl: Chlorophyll; CTR: Cu TRansporter families; DAGs: Directed acyclic graphs; DEGs: Differentially expressed genes; DVR: Divinyl chlorophyllide, an 8-vinyl-reductase; FPN: FerroportinN; FTIR: Fourier transform infrared; FTR: Fe TRansporter; GO: Gene Ontology; IC50: Growth half maximal inhibitory concentration; ICP: Inductively coupled plasma; MF: molecular function; NRAMPs: Natural resistance-associated aacrophage proteins; OD: Optical density; RPKM: Reads Per Kilobase of Exon Per Million Reads Mapped; VIT1: Vacuolar iron transporter 1 families; ZIPs: Zrt-, Irt-like proteins.
1. Introduction
Heavy metals, including cadmium (Cd), have become a severe issue and attract expert attention because of their toxicity, accumulation, and biomagnification in living organisms, accumulating in aquatic species and entering the food chain [Citation1]. The primary sources of metal pollution are industrial production and waste, fossil fuel combustion, mining, electroplating, dye and pigment manufacturing, and agricultural fertilizers [Citation2]. Cd contaminant sources are commonly from mining, wastewater treatment, the galvanoplasty industry, and semiconductor alloys for household products [Citation3]. Cd can be transported to living organisms from the water and food chain and cause serious symptoms such as Itai-Itai disease in Japan [Citation4].
Since heavy metals are very toxic to the environment and living organisms, they must be decontaminated. Microalgal-based treatment has excellent prospects as an alternative technology for removing Cd from polluted water [Citation5]. Some microalgae have been reported to be able to remove Cd [Citation6], such as Desmodesmus pleiomorphus with 61.2 mg Cd g−1 dry biomass [Citation7], Chlorella pyrenoidosa (0. 27 mg g−1) and Scenedesmus acutus (0.25 mg g−1) [Citation8]. However, most studies used dried cells or focused on short-term exposure within less than 100 h [Citation9,Citation10]. Even though it was studied with living cells with long-term exposure, the application was mainly conducted under a neutral pH [Citation9]. However neutral pH can reduce the bioavailability of heavy metals, the tolerance of microalgae under low pH shows a substantial reaction to the actual effect at the concentration of the heavy metals [Citation11,Citation12]. A previous study reported that the acidic tolerant Stichococcus bacillaris has high Cd tolerance and removal at acidic pH, such as 5.0, although the optimum pH for growth was pH 8.0 [Citation13,Citation14]. More recently, a Stichococcus-like alga, Tetratostichococcus sp. P1 has been reported to show tolerance to Cd and acidic pH, with the optimum pH for growth at pH 3.0, unlike the Stichococcus bacillaris strain [Citation15,Citation16]. However, the mechanism of tolerance and accumulation has not been clarified, particularly for long-term exposure to Cd stress of more than 100 h.
Generally, the remediation mechanism of heavy metals by microalgae involves two processes, i.e., biosorption and bioaccumulation. Biosorption is a nonmetabolic and rapid process [Citation17]. It is mainly governed by the negative charge of the functional groups on the cell surface due to the presence of polysaccharides, proteins, and phenolic acids which are rich in hydroxyl (−OH), carboxyl (−COOH), phosphate (-PO4), amino (-NH2), and sulfhydryl (−SH) [Citation17,Citation18]. On the other hand, bioaccumulation is a metabolism-dependent process feasible only in live cells [Citation19]. The bioaccumulation process involves some genes that can be identified by transcriptomic analysis. Heavy metal transport from the membrane to the cytosol is supported by Group A transporters, and localization of the metal is facilitated by Group B transporters [Citation20]. Some upregulated Group A transporters have been reported under Cd, such as natural resistance-associated macrophage proteins (NRAMPs), Zrt-, Irt-like proteins (ZIPs), Fe TRansporter (FTR), and Cu TRansporter (CTR) families [Citation21]. Then localization of the Cd into intracellular organelles is facilitated by Group B transporters such as cation diffusion facilitator (CDF), P1B-type ATPases, ferroportinN (FPN), and Ca (II)-sensitive cross complementary 1 (ccc1) or vacuolar iron transporter 1 (VIT1) families [Citation20]. Metallothioneins, glutathione, and phytochelatins also reported to be upregulated in microalgae under metal exposure [Citation22]. Mg-protoporphyrin IX chelating genes (CHLI, CHLH, CHLD) involved in chlorophyll synthesis together with catalase (CAT) were reported to be downregulated under Cd in A. protothecoides UTEX234 [Citation21]. Similarly, inhibited expression of ChlD divinyl chlorophyllide, an 8-vinyl-reductase (DVR), was also detected in C. sorokiniana cells under Cd stress [Citation23]. Further transcriptomic study is essential to understand the tolerance and mechanism of microalgae to Cd, especially under acidic conditions, in the long term.
The Cd tolerance and accumulation mechanism study of microalgae under low pH is still scarce and limited to short-term responses. In this study, the effect of Cd on Tetratostichococcus sp. P1 was identified, and the genes related to Cd tolerance and accumulation were revealed in the long term, more than 100 h. The result showed that Tetratostichococcus sp. P1 was tolerant and able to accumulate high Cd concentrations. Furthermore, some genes related to Cd tolerance and accumulation were found to be upregulated. Therefore, this research provides a unique strain from an acidic environment of peatland with high tolerance to Cd that can remove Cd and determine the mechanism of tolerance and accumulation of Cd from Tetratostichococcus sp. P1 based on transcriptomic analysis.
2. Materials and methods
2.1. Cd exposure to Tetratostichococcus sp. P1
Tetratostichococcus sp. P1 [Citation15] was maintained in a sterilized 50 mL conical flask containing 30 mL BG11 at pH 3.0 ± 0.1 at 30°C under 50 μmol photons m−2 sec−1 continuous light. The experimental culture was conducted in glass test tubes (height 20 cm, diameter 3 cm) containing 50 mL of BG11 medium pH 3.0 with citrate buffer to maintain the pH [Citation15]. The initial optical density of the experimental culture was 0.2 at a wavelength of 730 nm (OD730), equivalent to approximately 3 × 106 cells mL−1. The optical density and cell density were checked by a UV spectrophotometer (UV-1900, Shimadzu, Japan) and Cell counter (CDA-1000, Sysmex, Japan), respectively. Stock solutions of 100 mM Cd2+ were prepared from CdCl2·H2O (Kishida Chemical, Japan). In brief, 201.33 mg of CdCl2·H2O was dissolved in 10 mL of Milli-Q water (Merck Millipore, Burlington, MA) and filtered using a sterile 0.22 μm disposable syringe filter (Sigma‒Aldrich Japan, Tokyo). The Cd2+ concentrations were 10 µM, 20 µM, 50 µM, 100 µM, and 200 µM, which represent 1.12 mg L−1, 2.24 mg L−1, 5.60 mg L−1, 11.24 mg L−1, and 22.48 mg L−1, respectively. Those Cd concentration were chosen according to the previous study of Cd tolerance in acid-tolerant microalgae [Citation11] Then, the algae were cultivated under aeration system with 1% CO2 enrich air according to a previous study [Citation15]. The exposure experiments and analysis were performed in triplicate.
2.2. Growth and photosynthesis pigment measurement
The daily growth was monitored by checking the chlorophyll content of microalgae following a method previously described at a fixed time in the day. Briefly, a 1 mL of microalgal culture was harvested and centrifuged at 18,000 × g. Chlorophyll was extracted from algal cells using 80% acetone The pigment content, i.e., chlorophyll a (Chl a), chlorophyll b (Chl b), and carotenoid (Car), were calculated by EquationEquation 1(1)
(1) , EquationEquation 2
(2)
(2) , and EquationEquation 3
(3)
(3) , respectively [Citation24]: Total chlorophyll was calculated from the Chl a and Chl b values.
A663, A645, and A470 are the optical absorbances at 663 nm, 645 nm, and 470 nm, respectively.
The specific growth rate (μ) was calculated based on the chlorophyll content by EquationEquation 4(4)
(4)
ΣChl1 is the initial total chlorophyll measured at the start of the exponential stage, ΣChl2 is the total chlorophyll calculated in the last exponential phase, and Δt is the total days of the exponential stage. The Half maximal inhibitory concentration (IC50) was calculated by EquationEquation 5(5)
(5) and the analysis was done by Microsoft Excel. Each experiment and measurement were repeated at least three times.
is the total chlorophyll under Cd2+ after 6 d cultivation, and
o is total chlorophyll under control after 6 d.
While to obtain dry biomass, the microalga was harvested and freeze-dried. Briefly, 10 mL of culture was centrifuged at 18,000 × g at 4°C for 10 min to remove the medium. Then the cell was dried by a freeze dryer (FDU-1100, EYELA, Japan) at − 50°C at 10 Pa for 48 h. Then the dried cell weight was measured. To identify the effect of Cd2+ on the strain, the morphological image of the strain under a microscope (BX53, Olympus, Japan) at 100×magnifcation was analyzed based on shape, size, and color with the attached digital camera system (DP22).
2.3. Biochemical response
Six days of algal biomass was used to analyze the biochemical response of Tetratostichococcus sp. P1 to Cd. Briefly, 30 mL of alga was collected by centrifugation at 18,000 × g at 4°C for 10 min. The alga was kept at −80°C for 3 h and then freeze-dried (FDU-1100, EYELA) at − 50°C at 100 Pa for 48 h. Then reflection-fourier transform infrared (ATR-FTIR) spectroscopy was performed. The spectra acquired by FTIR spectroscopy IRTreaser-100 (Shimadzu) were scanned in the mid-IR range (400–4000 cm−1) [Citation25].
2.4. Analysis of the removal of heavy metal ions
To assay heavy metal removal by Tetratostichococcus sp. P1, the heavy metal concentration in the medium was analyzed after 6 d of cultivation. Before being analyzed using Inductively Coupled Plasma (ICP) emission spectroscopy (ICPS-8100, Shimadzu), the samples were pretreated by the ashing method (reported by Rashid et al., [Citation26]) with some modifications. Briefly, 10 mL of culture medium was separated from the alga by centrifugation at 18,000 × g at 4°C for 10 min. The supernatant was filtered through a sterile 0.22 μm disposable syringe filter (Sigma‒Aldrich). The sample was concentrated by a freeze dryer (FDU-1100, EYELA) at − 50°C at 10 Pa for 48 h and dissolved in 2 mL of concentrated HNO3. The solution was kept on a hot plate at 100°C for 1 h for the ashing process. After cooling, the volume was increased to 10 mL by adding deionized distilled water and filtered through an a sterile disposable syringe filter (0.22 μm) before analysis.
The removal capacity of heavy metals in the medium was calculated by EquationEquation 6(6)
(6)
C0 and Ct are the initial heavy metal concentrations before and after treatment (mg L−1). The total metal uptake per dry biomass (qe, mg g−1) was determined by EquationEquation 7(7)
(7) [Citation27].
where C0 and Ct (mg L−1) are the initial element concentration in the medium, and the concentration after the treatment, V (L) is the medium volume, and m (g) is the microalgae mass in the medium after the treatment in dry weight.
2.5. Transcriptomic analysis
2.5.1. RNA extraction
To study the effect of Cd exposure on the transcriptomic level of Tetratostichococcus sp. P1, 20 mL of log stage (5 d) sample of 100 μM Cd2+ treatment and control cultures were pelleted by centrifugation at 18,000 × g. Total RNA was extracted using the NucleoBond RNA Soil Midi kit (Macherey-Nagel, Germany). RNA was eluted with 100 μL of water. RNA was then subjected to DNase treatment at 37°C using a Rapid Out DNA Removal Kit (Thermo Scientific, USA). DNase was removed using a DNase removal reagent according to the manufacturer’s protocol. Finally, the purified RNA was transferred to a new tube for downstream application. RNA quantification was analyzed using a Bioanalyzer RNA Analysis – Agilent RNA 6000 Nano Kit (Agilent, USA).
2.5.2. Sequencing preparation
Next-generation sequencing (NGS) and transcriptome analysis were performed at Apical Scientific Sdn. Bhd., Malaysia. NEBNext Ultra RNA Library Prep Kit for Illumina (New England Biolabs, USA) was used for library construction. Subsequently, the library was sequenced using the Novaseq 6000 Pair-end 150 bp format. From RNA sample extraction to the final data obtained, every step of the procedures containing sample testing, library construction, and sequencing was qualitative and quantitative. The sequence was filtered by removing reads containing adapter contamination, removing reads containing more than 10% unknown reads (N > 10%), and eliminating low-quality nucleotides (base quality value less than 5) that constituted more than 50% of the read. The original sequence of Tetratostichococcus sp. P1 under Cd treat and control was deposit in Sequence Read Archive (SRA) National Center for Biotechnology Information (NCBI) (BioProject accession number: PRJNA1049646).
2.5.3. Bioinformatic analysis
Seven databases were applied by Novogene (China) to achieve comprehensive gene functional annotation. The software and parameters used in each database were NT: NCBI blast. The e-value threshold was 1e−5 (each unigene showed the top 10 alignment results). NR, SwissProt, KOG: Diamond. The value threshold is 1e−5 (each unigene shows the top ten alignment results). PFAM (The prediction of protein structure domain): HMMER package, hmmscan. The E-value threshold was 0.01; GO (based on the protein annotation results of NR and Pfam): Blast2GO and novogene script. The e-value threshold was 1e−6.
2.6. Statistical analysis
SPSS Statistical Software (ver.25, IBM, USA) was used for statistical analysis, and the statistically significant differences between means were determined using one-way ANOVA post hoc Tukey’s test (p ≤0.05). For the statistical significance of the NGS results, genome-wide studies statistic software was used [Citation28].
3. Results
3.1. Effect of Cd on the growth and pigment of Tetratostichococcus sp. P1
In this study, the tolerance of Tetratostichococcus sp. P1 to Cd2+ exposure was assessed by cultivating the algae in Cd2+-supplied media at pH 3.0 ± 0.1. The results showed that Tetratostichococcus sp. P1 was tolerant to Cd2+, as indicated by the strain that grew under Cd2+ (). The reduction in total chlorophyll contents followed the Cd concentration in the medium; the higher the Cd2+ concentration, the more significant the chlorophyll decrease in the strain. The chlorophyll and carotenoid contents of Tetratostichococcus sp. P1 also decreased in the presence of Cd (). The Chl a, Chl b, and carotenoid contents were shown to be significantly lower (p < 0.05) in all Cd conditions compared to Cd-free conditions. After 6 d of cultivation, the total chlorophyll under the control was 46.5 ± 1.0 µg mL−1 with Chl a and Chl b, i.e., 32.6 ± 1.0 µg mL−1 and 13.9 ± 1.0 µg mL−1, respectively, while the amount of carotenoids was 9.4 ± 0.5 µg mL−1. The lowest Chl a, Chl b, and carotenoid contents were found under 100 µM, with values of 8.5 ± 0.5 µg mL−1, 3.2 ± 0.5 µg mL−1, and 2.9 ± 0.5 µg mL−1, respectively. Under 200 µM, the pigment was not measured since the sufficient algal biomass could not be obtained. Tetratostichococcus sp. P1 had a significantly (p < 0.05) lower μ value under Cd exposure than the control (). The control had an μ value = 6.6 ± 0.2 d−1, while under Cd treatments, the μ values were 5.4 ± 0.2 d−1, 5.2 ± 0.2 d−1, 3.46 ± 0.2 d−1, and 2.0 ± 0.2 d−1 at 10 µM, 20 µM, 50 µM, and 100 µM Cd, respectively. Based on these growth parameters, the half-maximal inhibitory concentration (IC50) of Cd to the strain was 57.0 ± 2.0 µM (Fig. S1).
Figure 1. The tolerance of Tetratostichococcus sp. P1 under the presence of Cd. (a) Daily total Chl. (b) Pigment content. (c) Daily growth rate Chl (µ). Statistically significant differences between means were determined using one-way ANOVA post-hock Turkey’s test (*=p ≤0.05) following IBM SPSS statistical software (ver.25). Error bar is standard deviation (n = 3).

3.2. Effect of Cd2+ exposure on morphology of Tetratostichococcus sp. P1
As evidenced by the chlorophyll content, the microscope assessment also showed changes in the cell morphology, color and organelle (). Under the Cd-free conditions, the cell body of Tetratostichococcus sp. P1 was mainly green and rod-shaped, with a typical length of 3–7 µm. (). However, some nongreen areas in the cells appeared in the presence of Cd, and the nongreen areas became more extensive as the concentration increased (). The length of the cell expansion increased with Cd2+ concentration. The typical cell size was 12–17 µm under 10 µM Cd, 12–21 µm under 20 µM Cd2+, and 14–28 µm under 50 µM Cd2+. The largest cell size was found at 100 µM, ranging from 15 to 40 µm. At 100 µM, most of the cells were impacted, with an empty chlorophyll appearance. The impact on morphology includes changes in cell shape and size.
Figure 2. Morphological image of Tetratostichococcus sp. P1 under a microscope at 100× magnification. (a) morphology under control. (b) Morphology under 10 µM of Cd. (c) Morphology under 20 µM of Cd. (d) Morphology under 50 µM of Cd. e - f. morphology under 100 µM of Cd. Yellow arrow showing vacuole-like organel. Blue arrow showing non green part of the cell. The picture was taken after 6 days of cultivation.

3.3. Biochemical response of Tetratostichococcus sp. P1 under Cd exposure
ATR-FTIR spectra showed biochemical changes in Tetratostichococcus sp. P1 after their growth in the presence of Cd (Fig. S2). Various biochemicals corresponding to the spectral peaks were identified, such as carbohydrates at 900–1200 cm−1, proteins at 1550 and 1650 cm−1, lipids/esters at 1728–1745 cm−1, alkynes at 2250 cm−1 and lipids at 2855–2925 cm−1. The cellular proteins found in the microalgal cultures used in this study fall into the categories of amide I (1650 cm−1) and amide II (1550 cm−1) [Citation29]. All the biochemicals identified were affected by Cd. Carbohydrates that serve as energy reserves and proteins of amide I and amide II were the predominant macromolecules in control cultures. Carbohydrates and proteins were lower in Cd-treated samples than in Cd-free samples. Another vital biochemical, lipids/ester and lipid, which determines the final value of the primarily generated algal biomass, resolved significantly at 140 and 2855–2925 cm−1. Interestingly, Tetratostichococcus sp. P1 significantly accumulated more lipids/ester when grown at higher Cd concentrations.
3.4. Cd2+ removal capacity of Tetratostichococcus sp. P1
After treatment for 6 d, the amount of Cd in the medium was measured. According to the findings, Tetratostichococcus sp. can remove Cd, as evidenced by the Cd elimination in the medium (). The remaining Cd concentration in the medium decreased from 2.24 mg L−1 to 1.45 mg L−1 in the presence of 20 µM Cd. This result demonstrated that Tetratostichococcus sp. P1 removed 0.79 mg L−1 (35.4%) of Cd. From 50 µM (5.60 mg L−1), the concentration remained at 4.06 mg L−1 or decreased by 1.54 mg L−1 (27.4%); at 100 µM (11.2 mg L−1), the remaining Cd concentration in the medium was 6.88 mg L−1, i.e. microalgae removed 4.35 mg L−1 (38.8%) of Cd. After 6 d of Cd treatment, the biomass of Tetratostichococcus sp. P1 was determined. The results demonstrated that the biomass was diminished at higher Cd concentrations. The total dry biomass of Tetratostichococcus sp. P1 under control, 20, 50, and 100 µM Cd were 0.29, 0.27, 0.17, and 0.13 g in 50 mL, respectively, the maximum biosorption capacity of Tetratostichococcus sp. P1 was, therefore, 1.55 mg g−1 dry weight biomass.
3.5. Transcriptomic analysis
3.5.1. Differentially expressed genes
After 5 d of exposure, the cells were analyzed for transcriptomics. The differentially expressed genes (DEGs) in Tetratostichococcus sp. P1 was assessed in response to Cd exposure. The control and Cd-treated had 844 different genes and shared 9324 of the same genes (Fig. S3a). Under Cd treatment, 327 genes were upregulated, and 9506 were downregulated (Fig. S3b and S3c).
3.5.2. GO enrichment analysis
Gene Ontology (GO) annotation was performed to identify the biological roles of the identified DEGs. GO annotation was grouped into three main GO domains: biological process (BP), cellular component (CC), and molecular function (MF) (). In the BP category, the dominant functions were cellular and metabolic processes. In CC, cellular, anatomical entity, intracellular, and protein-containing complexes were dominant. Binding and transporter activity proteins showed the highest number in MF. Compared with the control, directed acyclic graphs (DAGs) showed that most GO enrichment was abundant in the CC category (Fig. S4), with 13 enriched GO terms and one significantly enhanced GO term in the BP and MF categories. The enriched GO in CC involves intracellular organelles such as ribosomes, cytoplasm, cytosol, cell wall (binding), nucleus, and nuclear chromosomes. In the BP category, the enriched term was small molecule metabolism, while in MF, it was oxidoreduction activities.
3.6. Regulated genes under Cd exposure.
To further understand the molecular mechanism and the genes involved in Cd remediation by Tetratostichococcus sp. P1, the upregulation of specific genes under Cd treatment was identified based on the seven databases described in the Methods. The reads per kilobase of exon per million reads mapped (RPKM) values of the Cd-treated and control groups were compared (Tables S1-S5). From 327 upregulated genes, 70 genes were selected, where 28 upregulated genes were related to metal ion binding, such as metallothionein-like protein (Table S1), and 20 genes were related to metal transporter Groups A and B, such as potassium ion transport protein (Table S2). Other than metal binding and transporters, essential upregulated genes in this study are listed in Tables S3 – S5, i.e. glutathione, calmodulin, proline, thioredoxin, auxin response factor, ethylene-responsive transcription factor, heat shock protein gene, and antioxidant metabolism-related gene. In contrast, the most significantly downregulated genes (Table S6) were described as zinc finger (41 genes), photosynthesis-related genes (12 genes), bZIP (12 genes), mitochondria electron transport, ABC-2 transporter, polysaccharide metabolic process, and cell division.
4. Discussion
At neutral pH, some microalgae can tolerate Cd, such as Polyedriopsis spinulosa and Chlorosarcinopsis bastropiensis, with IC50 values of 76.24 and 86.44 mg L−1, respectively [Citation30], while Scenedesmus sp. IITRIND2 reprorted with IC50 = 32 mg L−1. Compared to neutral pH conditions, few algae have been reported to have a high Cd tolerance in acidic conditions. One reason is that Cd is very toxic under acidic conditions [Citation31,Citation32] for instance, Heterochlorella sp. MAS3 and Desmodesmus sp. MAS1 can only grow at a maximum of 2 and 5 mg L−1 at pH 3.5 [Citation11]. The toxicity of Cd was also reported to C. acidophila with an IC50 of 1.94 μM at pH 2 [Citation33], while the acidic tolerance of Chlamydomonas CPCC 121 showed high tolerance to Cd (up to 600 μM) at pH 4. Nevertheless, it was not efficient enough to be considered for the phytoremediation of Cd since it has low Cd accumulation (<0.1 mg Cd mg−1 of dry weight). Stichococcus bacillaris grow in the media with 10 mg L−1 Cd was close to that in the culture without Cd, although the algal growth was optimum at H 8.0 [Citation34]. This study showed that Tetratostichococcus sp. P1 has a high level of tolerance to Cd under acidic conditions. Based on chlorophyll growth, Tetratostichococcus sp. P1 showed an ability to grow up to 100 μM, and the IC50 was 54 μM. This result was lower than the IC50 of the growth OD (i.e. 110 μM) [Citation16]. Sbihi et al. [Citation35] reported that the chlorophyll fluorescence parameter was more sensitive than the growth (OD) parameter. Thus, the IC50 for chlorophyll was lower than the growth (OD). Since the cells were enlarged and nongreen areas were increased in the cells of Tetratostichococcus sp. P1, IC50 based on OD, i.e. biomass, might be rather suitable to evaluate the tolerance of Cd in this alga. Photosynthetic pigments were decreased under Cd treatment, which might indicate that the chloroplasts of Tetratostichococcus sp. P1 was affected by Cd2+. Cd, as a heavy metal, has been reported to inhibit the normal function of the thylakoid membrane and chlorophyll biosynthesis [Citation36,Citation37]. The same trend has also been reported by [Citation11], where the chlorophyll and carotenoid contents of Desmodesmus sp. MAS1 and Heterochlorella sp. MAS3 was significantly lower under Cd treatment than in the Cd-free. In environment, and the relevant concentrations of Cd have been reported to be 4.92–49.2 μg L−1 [Citation5]. This indicates that Tetratostichococcus sp. P1 is tolerant to more than 200 times the environmentally relevant concentrations of Cd according to the survivability at 100 µM.
Tripathi and Poluri [Citation19] reported that the toxicity of heavy metals in microalgae depends on the intracellular concentration of metals. The ‘bioaccumulation’ allows Cd2+ to enter the cells. Inside the cell, Cd2+ disrupts the photosynthesis process in the chloroplast. In this study, higher concentrations of Cd had a more decisive influence on the growth and chlorophyll content of Tetratostichococcus sp. P1. This result demonstrated a direct correlation between the concentration and the Cd2+ transport mechanism. The cell membrane metal transporters i.e Group A transporters such as CTR, ZIP, and FTR were highly upregulated under high concentrations of Cd. As a result, the high intracellular concentration of Cd significantly impacts the cell, reducing the photosynthetic activity and growth rate.
Additionally, compared to control cells, Cd-treated cells had a significantly increased number and size of organel possibly because of the accumulation of Cd. A similar result was reported by León-Vaz et al. [Citation38] and Carfagna et al. [Citation39], where the fluorescence of chlorophylls in Chlor. sorokiniana was lower in the cells, and the vacuole was larger under Cd. The Cd mechanism in microalgae involves cation transport to the vacuole, which is mediated by phytochelatins [Citation40]. Wang et al. [Citation41] reported that Cd exposure strongly modified the ultrastructure of Chlor. pyrenoidosa and led to decreased photosynthetic activities. Notably, under Cd treatment, the number of vacuole like organelle increased, and the pigment content decreased. This phenomenon suggests that the photosynthesis system was damaged. Then, membrane lipid peroxidation occurs, promoting cell damage and reducing cell growth, implying a potentially lethal effect [Citation42]. The current result was in agreement with this theory. This trend has also been reported in E. gracilis under a mix of Cd exposure and macroparticle [Citation24]. In addition, Tetratostichococcus sp. P1 morphology was changed as a response to environmental stress, i.e. 2% CO2 [Citation15].
Biochemical response of Tetratostichococcus sp. P1 under Cd showed decreased carbohydrates and proteins but a significant increase in lipid esters. The same result was demonstrated in acidophilic algae such as E. gracilis and the acid-tolerant algae Heterochlorella MAS when exposed to Cd; they accumulated less carbohydrate and protein, but lipid accumulation was higher [Citation11,Citation43]. However, some microalgae have suggested that Cd stress increases the number of carbohydrates at neutral pH, such as in Chlor. vulgaris [Citation11,Citation44]. Therefore, the biochemical responses of microalgae to Cd might be different under low pH from than that under neutral pH.
Tetratostichococcus sp. P1 showed the ability to remove Cd in the medium. The maximum Cd2+ removal yield demonstrated that Tetratostichococcus sp. P1 cells as a potentially efficient cadmium biosorbent under acidic conditions compared to previous studies using living cells under acidic conditions. Most studies have reported Cd accumulation/removal by microalgal strains grown at neutral pH or acidophilic microalgae at acidic pH – for instance, Desmodesmus sp. MAS1 and Heterochlorella sp. MAS3 accumulated 0.77 and 0.35 mg g−1 dry weight at pH 3.5 [Citation11]. D. armatus accumulated 159–341 μg g−1 dry weight of Cd from 93 μM Cd at pH 7 [Citation45].
Transcriptomic analysis was conducted further to understand Cd tolerance, accumulation mechanism, and effect from molecular insight in Teratostichococcus sp. P1. The top GO terms with the most abundant DEGs (Fig. S3) were selected from the three major functional categories, BP, CC, and MF. Interestingly, the most significant gene enrichment was transport (), such as transmembrane transport activity. In addition, ATPase activity, a Group B transporter, also showed significant enrichment. These data indicate that Cd2+ transporter-related genes were very highly expressed in Tetratostichococcus sp. P1. These data might explain the Cd transport and accumulation inside the cell, reducing Cd2+ in the medium (). The GO enrichment results support this theory, and the microscopic results show a more prominent vacuole-like organelle which was not stained by DAPI (data not shown) after Cd treatment (). Transmembrane transport, also the outflow of excess transition metal ions from the cytoplasm to the exterior of the cells or subcellular compartments, is another primary method of heavy metal detoxification by microalgae [Citation18]. Small molecule metabolic processes also showed enrichment in BP. In addition, the redox-related GO category oxidoreductase activity was enriched. The biological redox reaction converts the oxidation states of the uptaken heavy metals to allow further detoxification [Citation46,Citation47]. A previous study established that the expression of oxidative stress genes might be enhanced in microalgae under Cd exposure [Citation24].
Under Cd treatment, the most upregulated gene was the metallothionein gene, ie metallothionein-like protein and metallothionein family 11, with respective 155% and 95%; metallothionein is one of the famous genes related to heavy metal remediation located in the cytosol [Citation48]. Metallothionein is critical in supplying essential metals to the cell and transporting toxic metals into other organelles. It has also been proposed that they act as free-radical scavengers, protecting cells from oxidative stress [Citation49]. Metallothionein upregulation is also supported by the enriched GO term involving intracellular organelles, including the cytosol.
After binding in the cell, heavy metals are transported from the membrane to the cytosol, which is facilitated by Group A transporters [Citation9]. This study also found that the upregulated Group A transporters includingCTR, ZIP, and FTR. Those transporters are responsible for moving metal ions from the cell wall into the cytoplasm; those groups were found in the plasma membranes and the vacuole membranes and had the same function as assimilative transporters [Citation20]. Other than Group A transporters some transmembrane transporters Group B were also upregulated, such as V-type ATPase, CDF, MSC, and VIT/ccc1. CDF was shown to be the most upregulated among metal transporter proteins, with 142%. The CDF transporter will localize Cd to various intracellular compartments. The potassium ion transporter activities indicated CDF transporter regulation. ATPase transporter, which has the same function as CDF, was found to be upregulated. The MSC transporter, a mitochondrial transporter, was also upregulated, but the ABC-2 transporter was significantly downregulated. Downregulated ABC transporters in mitochondria might indicate the preventive uptake of Cd by mitochondria. Therefore, most of the Cd inside the cells was considered to be transported to the vacuole. Cd transport to the vacuole was supported by upregulating a specific metal transporter, VIT. This study also found downregulated mitochondrial electron transport, indicating that Cd exposure affected mitochondria and inhibited electron transport in mitochondria. Tripathi and Poluri [Citation19] stated that Cd ions inside the cells could disrupt the electron transport chain in mitochondria. Some necessary membrane transporters sequester these conjugates into vacuoles [Citation36]. These conjugates are further sequestered into vacuoles with the help of some crucial membrane transporters, such as phytochelatins.
Phytochelatins play a role in detoxifying and accumulating Cd by cation transport to the vacuole [Citation40]. The involvement of phytochelatins was also suggested in Cd tolerance under acidic conditions because Cd strongly induced the phytochelatin synthase CaPCS2 in the acidophilic green alga Chlamy. acidophila [Citation50]. This gene does not exist in non-Cd-resistant Chlamydomonas based on transcript annotations [Citation51]. The current study identified upregulated genes encoding phytochelatin proteins. This finding agreed with the microscopic result showing an increase in the vacuole-like organelle. This result might explain the increased organelle size phenomena in Tetratostichococcus sp. P1.
In contrast, this study found zinc fingers to be the most downregulated gene under Cd treatment in Tetratostichococcus sp. with 41 genes. Downregulated zinc finger in Tetratostichococcus sp. P1 might interfere with DNA transcription and repair; as a result, some proteins might lose their function and interfere with strain cell growth. The zinc finger gene is crucial in transcription factors and DNA repair proteins, mediating DNA-amino acid and protein binding [Citation52]. Zinc finger disruption may cause the restriction of cellular processes, including growth control, gene expression, and integrity [Citation52]. These transcriptome data also show downregulation of the cell division protein ZapB. The ZapB gene plays a vital role in forming the initial Z-ring, where the Z-ring is the first process in cell division [Citation53]. The downregulation of the zinc finger and ZapB genes might inhibit cell mitosis. Therefore, the Tetratostichococcus sp. P1 cell was expanded and more extensive under Cd stress than the control cells. It has also been reported that Cd causes a dose-dependent disturbance in growth, cell division, and morphogenesis, as well as the ultrastructure and function of microalgae organelles [Citation54].
Similar to zinc finger, basic leucine zipper (bZIP) transcription factors were found to be downregulated under Cd treatment. bZIP plays a crucial role in growth, metabolism, and environmental responses. It is one of the plant’s prominent transcription factor families [Citation55,Citation56]. The role of bZIP transcription factors in microalgae is still poorly understood. Ji et al. [Citation57] reported that 6 bZIP transcription factor genes were induced to change significantly in Chlamy. reinhardtii during salt stress when salt stress caused lower cell growth and photosynthesis but higher lipid accumulation. Bai et al. [Citation58] found that bZIP transcription factor genes played a role in pigment metabolism in Chlamy. reinhardtii. The disruption of bZIP impacted pigment synthesis and decreased the chlorophyll a, b, and carotenoid contents in Chlamy. reinhardtii. Therefore, bZIP downregulation might be responsible for the low pigment content in Tetratostichococcus sp. such as chlorophyll a and b and carotenoids. bZIP might be used as a gene target for genetic engineering projects in microalgae-related environmental responses. This is also supported by the downregulation of some photosynthesis-related genes in Tetratostichococcus sp. P1, such as Photosystem I reaction center, chloroplastic, and chlorophyll metabolic process. Disruption of the photosynthetic system in the chloroplast and inhibition of electron transport in mitochondria have caused ROS generation and ROS accumulation.
Ros accumulation stimulated the upregulation of glutathione and oxidative stress management agencies (Tables S3 – S4). Glutathione acts as an intrinsic suite of a mechanism comprising low- and high-molecular-weight antioxidants to survive the oxidative stress generated due to the invasion of heavy metals; in other words, glutathione plays a vital role in detoxifying heavy metals. Previous reports have shown significantly upregulated glutathione genes in microalgae such as Chlamy. reinhardtii and Dunaliella acidophila under Pb and Cd exposure [Citation59,Citation60]. At the same time, other genes play a role in managing oxidative stress caused by heavy metals in microalgae, such as reactive oxygen species (ROS). ROS production is known to be one of the major toxic effects on algal cells under Cd stress. A previous study reported that algae use ROS as signaling molecules to drive cellular responses to environmental changes [Citation61]. ROS generation is a common phenomenon that occurs within cells due to oxidative metabolism. Subcellular organelles in microalgae, such as chloroplasts and mitochondria, are more vulnerable to ROS due to the presence of essential metal ions and the production of superoxide ions during the oxygen reduction process in the electron transport chain [Citation22]. The boosting of some genes in antioxidant metabolism might help to explain the tolerance and detoxification of Cd by Tetratostichococcus sp. P1.
5. Conclusion
This is the first study on the molecular mechanism of tolerance and accumulation of Cd in Tetratostichococcus sp. P1 under acidic conditions. The results showed that Tetratostichococcus sp. P1 had a high tolerance and could eliminate Cd in the medium Cd exposure damages the morphology and biochemical of the algae. Transcriptomic analysis revealed that Cd tolerance and accumulation mechanisms in Tetratostichococcus sp. P1. Metallothionein was highly upregulated under Cd exposure. Group A transporters, i.e., CTR, ZIP, and FTR, transported the Cd ion to the cytosol. Then, from the cytosol, Group B transporters (V-type ATPase, CDF, MSC, and VIT/ccc1) facilitated Cd localization to some organelles, such as mitochondria, chloroplasts, and vacuoles. The Cd inside the cells disrupted the mitochondria and chloroplasts, which caused ROS generation and calcium ions, which acted as the second signal. ROS accumulation activated antioxidant genes to combat excessive ROS. Downregulation of some genes, such as zinc finger, ZapB, and cell division protein, interfered with cell growth and mitosis and caused morphological changes in Tetratostichococcus sp. P1. The full potential of the identified acid-tolerant microalgal strains will be substantially enhanced by future large-scale studies on the effects of mixed metals on growth and Cd removal. In addition, more research on genetic engineering study will greatly help in determining the full potential of the identified gene for Cd bioremediation.
Authors Contribution
Eri Sahabudin: Conceptualization, Data curation, Methodology, Investigation, Formal analysis, Writing Original draft preparation. Shohei Kubo, Kohei Yoneda, Yoshiaki Maeda: Resources, Project administration, Data Curation, Software, Validation. Fazrena Nadia Md Akhir, Nor’azizi Othman, Kengo Suzuki: Visualization, Investigation. Ali Muhammad Yuzir, Iwane Suzuki, Hirofumi Hara, and Koji Iwamoto: Supervision, Conceptualization, Funding acquisition, Reviewing, Editing
Supplemental Material
Download MS Word (1.3 MB)Acknowledgments
The authors thank Muhammad Fakhri (University of Tsukuba) and Shartika Jusoh (Universiti Teknologi Malaysia) for their excellent technical assistance.
Disclosure statement
No potential conflict of interest was reported by the author(s).
Data availability statement
All data generated or analyzed during this study are included in this published article (and its Supplementary Information files). The species sequence data used in this paper have been superseded by the 18S GenBank record [MT053478] (https://www.ncbi.nlm.nih.gov/nuccore/MT053478.1). The raw data that used for transcriptomic analysis was deposited in to BioProject, NCBI [PRJNA1049646] (https://www.ncbi.nlm.nih.gov/bioproject/?term=PRJNA1049646).
Supplementary material
Supplemental data for this article can be accessed online at https://doi.org/10.1080/21655979.2024.2314888
Additional information
Funding
References
- Baby J, Raj J, Biby E, et al. Toxic effect of heavy metals on aquatic environment. Int J Biol Chem Sci. 2010;4(4):939–16. doi: 10.4314/ijbcs.v4i4.62976
- Zakhama S, Dhaouadi H, M’Henni F. Nonlinear modelisation of heavy metal removal from aqueous solution using ulva lactuca algae. Bioresour Technol. 2011;102(2):786–796. doi: 10.1016/j.biortech.2010.08.107
- Leong YK, Chang JS. Bioremediation of heavy metals using microalgae: recent advances and mechanisms. Bioresour Technol. 2020;303:122886. doi: 10.1016/j.biortech.2020.122886
- Qi K, Ren L, Bai Z, et al. Detecting cadmium during ultrastructural characterization of hepatotoxicity. J Trace Elem Med Biol. 2020;62:126644. doi: 10.1016/j.jtemb.2020.126644
- Yu Z, Wei H, Hao R, et al. Physiological changes in Chlamydomonas reinhardtii after 1000 generations of selection of cadmium exposure at environmentally relevant concentrations. Environ Sci Process Impacts. 2018;20(6):923–933. doi: 10.1039/c8em00106e
- Perales-Vela HV, Peña-Castro JM, Cañizares-Villanueva RO. Heavy metal detoxification in eukaryotic microalgae. Chemosphere. 2006;64(1):1–10. doi: 10.1016/j.chemosphere.2005.11.024
- Monteiro CM, Castro PML, Malcata FX. Cadmium Removal by Two Strains of Desmodesmus pleiomorphus Cells. Water Air Soil Pollut. 2010;208(1–4):17–27. doi: 10.1007/s11270-009-0146-1
- Chandrashekharaiah PS, Sanyal D, Dasgupta S, et al. Cadmium biosorption and biomass production by two freshwater microalgae scenedesmus acutus and chlorella pyrenoidosa: an integrated approach. Chemosphere. 2021;269:128755. doi: 10.1016/j.chemosphere.2020.128755
- Kumar KS, Dahms HU, Won EJ, et al. Microalgae - a promising tool for heavy metal remediation. Ecotoxicol Environ Saf. 2015;113:329–352. doi: 10.1016/j.ecoenv.2014.12.019
- Zhu Q, Zhang M, Bao J, et al. Physiological, metabolomic, and transcriptomic analyses reveal the dynamic redox homeostasis upon extended exposure of Dunaliella salina GY-H13 cells to Cd. Ecotoxicol Environ Saf. 2021;223:112593. doi: 10.1016/j.ecoenv.2021.112593
- Abinandan S, Subashchandrabose SR, Venkateswarlu K, Perera IA, Megharaj M. Acid-tolerant microalgae can withstand higher concentrations of invasive cadmium and produce sustainable biomass and biodiesel at pH 3.5. Bioresour Technol. 2020;281:469–473. doi: 10.1016/j.biortech.2019.03.001
- Subashchandrabose SR, Megharaj M, Venkateswarlu K, et al. Interaction effects of polycyclic aromatic hydrocarbons and heavy metals on a soil microalga, Chlorococcum sp. MM11. Environ Sci Pollut Res. 2015;22(12):8876–8889. doi: 10.1007/s11356-013-1679-9
- Birungi ZS, Chirwa EMN. Interpretation of uptake kinetic of thallium and cadmium on surfaces of immobilized green algae as biosorbents. Chem Eng Trans. 2016;49:421–426. doi: 10.3303/CET1649071
- Skowroński T. Uptake of cadmium by Stichococcus bacillaris. Chemosphere. 1984;13(12):1385–1389. doi: 10.1016/0045-6535(84)90052-3
- Sahabudin E, Lee J, Asada R, et al. Isolation and characterization of acid-tolerant stichococcus-like microalga (Tetratostichococcus sp. P1) from a tropical peatland in Malaysia. J Appl Phycol. 2022a;34(4):1881–1892. doi: 10.1007/s10811-022-02762-7
- Sahabudin E, Othman NA, Suzuki I. High cadmium tolerance in stichoccocus-like microalgae (Tetratostichoccocus sp. P1) from Malaysia. IOP Conf Ser Earth Environ Sci. 2022b;1091(1):012045. doi: 10.1088/1755-1315/1091/1/012045
- Tripathi S, Arora N, Gupta P, et al. Microalgae: an emerging source for mitigation of heavy metals and their potential implications for biodiesel production, advanced biofuels: applications, technologies and environmental sustainability. Elsevier Ltd; 2019. doi: 10.1016/B978-0-08-102791-2.00004-0
- Priyadarshini E, Priyadarshini SS, Pradhan N. Heavy metal resistance in algae and its application for metal nanoparticle synthesis. Appl Microbiol Biotechnol. 2019;103(8):3297–3316. doi: 10.1007/s00253-019-09685-3
- Tripathi S, Poluri KM. Heavy metal detoxification mechanisms by microalgae: insights from transcriptomics analysis. Environ Pollut. 2021;285:117443. doi: 10.1016/j.envpol.2021.117443
- Blaby-Haas CE, Merchant SS. The ins and outs of algal metal transport. Biochim Biophys Acta, Mol Cell Res. 2012;1823(9):1531–1552. doi: 10.1016/j.bbamcr.2012.04.010
- Lu JJ, Ma YL, Xing GL, et al. Revelation of microalgae’s lipid production and resistance mechanism to ultra-high Cd stress by integrated transcriptome and physiochemical analyses. Environ Pollut. 2019;250:186–195. doi: 10.1016/j.envpol.2019.04.018
- Balzano S, Sardo A, Blasio M, et al. Microalgal metallothioneins and phytochelatins and their potential use in bioremediation. Front Microbiol. 2020;11:1–16. doi: 10.3389/fmicb.2020.00517
- Ding N, Wang L, Kang Y, et al. The comparison of transcriptomic response of green microalga chlorella sorokiniana exposure to environmentally relevant concentration of cadmium(II) and 4-n-nonylphenol. Environ Geochem Health. 2020;42(9):2881–2894. doi: 10.1007/s10653-020-00526-1
- Liao Y, Jiang X, Xiao Y, et al. Exposure of microalgae Euglena gracilis to polystyrene microbeads and cadmium: perspective from the physiological and transcriptional responses. Aquatic Toxicol. 2020;228:105650. doi: 10.1016/j.aquatox.2020.105650
- Abinandan S, Subashchandrabose SR, Pannerselvan L, et al. Potential of acid-tolerant microalgae, Desmodesmus sp. MAS1 and Heterochlorella sp. MAS3, in heavy metal removal and biodiesel production at acidic pH. Bioresour Technol. 2019;278:9–16. doi: 10.1016/j.biortech.2019.01.053
- Rashid MH, Fardous Z, Chowdhury MA, et al. Determination of heavy metals in the soils of tea plantations and in fresh and processed tea leaves: an evaluation of six digestion methods. Chem Cent J. 2016;10(1):7. doi: 10.1186/s13065-016-0154-3
- Jayakumar V, Govindaradjane S, Senthil Kumar P, et al. Sustainable removal of cadmium from contaminated water using green alga – optimization, characterization and modeling studies. Environ Res. 2021;199:111364. doi: 10.1016/j.envres.2021.111364
- Storey JD, Tibshirani R. Statistical significance for genomewide studies. Proc Natl Acad Sci U S A. 2003;100(16):9440–9445. doi: 10.1073/pnas.1530509100
- Wagner H, Liu Z, Langner U, et al. The use of FTIR spectroscopy to assess quantitative changes in the biochemical composition of microalgae. J Biophoto. 2010;3(8–9):557–566. doi: 10.1002/jbio.201000019
- Nanda M, Jaiswal KK, Kumar V, et al. Bio-remediation capacity for Cd(II) and Pb(II) from the aqueous medium by two novel strains of microalgae and their effect on lipidomics and metabolomics. Water Proc Eng. 2021;44:102404. doi: 10.1016/j.jwpe.2021.102404
- Fathi A. Effects of Ph on toxicity of cadmium, cobalt and copper to the green alga Scenedesmus bijuga. Egyptian J Phycol. 2004;5(1):107–117. doi: 10.21608/egyjs.2004.113991
- Xu Y, Shi D, Aristilde L, et al. The effect of pH on the uptake of zinc and cadmium in marine phytoplankton: possible role of weak complexes. Limnol Oceanogr. 2012;57(1):293–304. doi: 10.4319/lo.2012.57.1.0293
- Díaz S, de Francisco P, Olsson S, et al. Toxicity, physiological, and ultrastructural effects of arsenic and cadmium on the extremophilic microalga Chlamydomonas acidophila. Int J Environ Res Public Health. 2020;17(5):1650. doi: 10.3390/ijerph17051650
- Skowroński T, Szubiflska S, Pawlik B, et al. The influence of pH on cadmium toxicity to the green alga Stichococcus bacillaris and on the cadmium forms present in the culture medium. Environ Pollut. 1991;74(2):89–100. doi: 10.1016/0269-7491(91)90106-7
- Sbihi K, Cherifi O, El Gharmali A, et al. Toxicity and biosorption of chromium from aqueous solutions by the diatom Planothidium lanceolatum (brébisson) lange-bertalot. J Mater Environ Sci. 2012;3(1):27–38. doi: 10.5251/ajsir.2012.3.1.27.38
- Hanikenne M, Krämer U, Demoulin V, et al. A comparative inventory of metal transporters in the green alga Chlamydomonas reinhardtii and the red alga Cyanidioschizon merolae. Plant Physiol. 2005;137(2):428–446. doi: 10.1104/pp.104.054189
- Lamai C, Kruatrachue M, Pokethitiyook P, et al. Toxicity and accumulation of lead and cadmium in the filamentous green alga Cladophora fracta (O.F. Muller ex vahl) Kutzing: a laboratory study. Science Asia. 2005;31(2):121–127. doi: 10.2306/scienceasia1513-1874.2005.31.121
- León-Vaz A, Romero LC, Gotor C, et al. Effect of cadmium in the microalga Chlorella sorokiniana: a proteomic study. Ecotoxicol Environ Saf. 2021;207:111301. doi: 10.1016/j.ecoenv.2020.111301
- Carfagna S, Lanza N, Salbitani G, et al. Physiological and morphological responses of lead or cadmium exposed Chlorella sorokiniana 211-8K (chlorophyceae). Springerplus. 2013;2(1):1–7. doi: 10.1186/2193-1801-2-147
- Cobbett CS. Phytochelatins and their roles in heavy metal detoxification. Plant Physiol. 2000;123(3):825–832. doi: 10.1104/pp.123.3.825
- Wang S, Zhang D, Pan X. Effects of cadmium on the activities of photosystems of Chlorella pyrenoidosa and the protective role of cyclic electron flow. Chemosphere. 2013;93(2):230–237. doi: 10.1016/j.chemosphere.2013.04.070
- Mao Y, Ai H, Chen Y, et al. Phytoplankton response to polystyrene microplastics: perspective from an entire growth period. Chemosphere. 2018;208:59–68. doi: 10.1016/j.chemosphere.2018.05.170
- Santiago-Martínez MG, Lira-Silva E, Encalada R, et al. Cadmium removal by Euglena gracilis is enhanced under anaerobic growth conditions. J Hazard Mater. 2015;288:104–112. doi: 10.1016/j.jhazmat.2015.02.027
- Chia MA, Lombardi AT, da Melão MGG, et al. Phosphorus levels determine changes in growth and biochemical composition of Chlorella vulgaris during cadmium stress. J Appl Phycol. 2017;29(4):1883–1891. doi: 10.1007/s10811-017-1111-9
- Pokora W, Baścik-Remisiewicz A, Tukaj S, et al. Adaptation strategies of two closely related Desmodesmus armatus (green alga) strains contained different amounts of cadmium: a study with light-induced synchronized cultures of algae. J Plant Physiol. 2014;171(2):69–77. doi: 10.1016/j.jplph.2013.10.006
- Mitra M, Kirst H, Dewez D, et al. Modulation of the light-harvesting chlorophyll antenna size in Chlamydomonas reinhardtii by TLA1 gene over-expression and RNA interference. Philos Trans R Soc B. 2012;367(1608):3430–3443. doi: 10.1098/rstb.2012.0229
- Singh S, Kumar V. Mercury detoxification by absorption, mercuric ion reductase, and exopolysaccharides: a comprehensive study. Environ Sci Pollut Res. 2020;27(22):27181–27201. doi: 10.1007/s11356-019-04974-w
- Ziller A, Fraissinet-Tachet L. Metallothionein diversity and distribution in the tree of life: a multifunctional protein. Metallomics. 2018;10(11):1549–1559. doi: 10.1039/c8mt00165k
- Capdevila M, Atrian S. Metallothionein protein evolution: a miniassay. J Biol Inorg Chem. 2011;16(7):977–989. doi: https://doi.org/10.1007/s00775-011-0798-3
- Olsson S, Penacho V, Puente-Sánchez F, et al. Horizontal gene transfer of phytochelatin synthases from bacteria to extremophilic green algae. Microb Ecol. 2017;73(1):50–60. doi: 10.1007/s00248-016-0848-z
- Puente-Sánchez F, Díaz S, Penacho V, et al. Basis of genetic adaptation to heavy metal stress in the acidophilic green alga Chlamydomonas acidophila. Aquatic Toxicol. 2018;200:62–72. doi: 10.1016/j.aquatox.2018.04.020
- Hartwig A. Zinc finger proteins as potential targets for toxic metal ions: differential effects on structure and function. Antioxid Redox Signal. 2001;3(4):625–634. doi: 10.1089/15230860152542970
- Ebersbach G, Galli E, Møller-Jensen J, et al. Novel coiled-coil cell division factor ZapB stimulates Z ring assembly and cell division. Mol Microbiol. 2008;68(3):720–735. doi: 10.1111/j.1365-2958.2008.06190.x
- Volland S, Bayer E, Baumgartner V, et al. Rescue of heavy metal effects on cell physiology of the algal model system micrasterias by divalent ions. J Plant Physiol. 2014;171(2):154–163. doi: 10.1016/j.jplph.2013.10.002
- Gai WX, Ma X, Qiao YM, et al. Characterization of the bZIP transcription factor family in pepper (Capsicum annuum L.): CabZIP25 positively modulates the salt tolerance. Front Plant Sci. 2020;11:1–18. doi: 10.3389/fpls.2020.00139
- Han Y, Hou Z, He Q, et al. Genome-wide characterization and expression analysis of bZIP gene family under abiotic stress in Glycyrrhiza uralensis. Front Genet. 2021;12:1–17. doi: 10.3389/fgene.2021.754237
- Ji C, Mao X, Hao J, et al. Analysis of bZIP transcription factor family and their expressions under salt stress in Chlamydomonas reinhardtii. Int J Mol Sci. 2018;19(9):2800. doi: 10.3390/ijms19092800
- Bai F, Zhang Y, Liu J. A bZIP transcription factor is involved in regulating lipid and pigment metabolisms in the green alga Chlamydomonas reinhardtii. Algal Res. 2021;59:102450. doi: 10.1016/j.algal.2021.102450
- Puente-Sánchez F, Olsson S, Aguilera A. Comparative Transcriptomic Analysis of the Response of Dunaliella acidophila (Chlorophyta) to Short-Term Cadmium and Chronic Natural Metal-Rich Water Exposures. Microb Ecol. 2016;72(3):595–607. doi: 10.1007/s00248-016-0824-7
- Zheng C, Aslam M, Liu X, et al. Impact of Pb on chlamydomonas reinhardtii at physiological and transcriptional levels. Front Microbiol. 2020;11:1443. doi: 10.3389/fmicb.2020.01443
- Mullineaux PM, Exposito-Rodriguez M, Laissue PP, et al. ROS-dependent signalling pathways in plants and algae exposed to high light: comparisons with other eukaryotes. Free Radic Biol Med. 2018;122:52–64. doi: 10.1016/j.freeradbiomed.2018.01.033