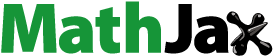
ABSTRACT
Hypervirulent Klebsiella pneumoniae isolates have been increasingly reported worldwide, especially hypervirulent drug-resistant variants owing to the acquisition of a mobilizable virulence plasmid by a carbapenem-resistant strain. This pLVPK-like mobilizable plasmid encodes various virulence factors; however, information about its genetic stability is lacking. This study aimed to investigate the type II toxin-antitoxin (TA) modules that facilitate the virulence plasmid to remain stable in K. pneumoniae. More than 3,000 TA loci in 2,000 K. pneumoniae plasmids were examined for their relationship with plasmid cargo genes. TA loci from the RES-Xre family were highly correlated with virulence plasmids of hypervirulent K. pneumoniae. Overexpression of the RES toxin KnaT, encoded by the virulence plasmid-carrying RES-Xre locus knaAT, halts the cell growth of K. pneumoniae and E. coli, whereas co-expression of the cognate Xre antitoxin KnaA neutralizes the toxicity of KnaT. knaA and knaT were co-transcribed, representing the characteristics of a type II TA module. The knaAT deletion mutation gradually lost its virulence plasmid in K. pneumoniae, whereas the stability of the plasmid in E. coli was enhanced by adding knaAT, which revealed that the knaAT operon maintained the genetic stability of the large virulence plasmid in K. pneumoniae. String tests and mouse lethality assays subsequently confirmed that a loss of the virulence plasmid resulted in reduced pathogenicity of K. pneumoniae. These findings provide important insights into the role of the RES-Xre TA pair in stabilizing virulence plasmids and disseminating virulence genes in K. pneumoniae.
Highlights
RES-Xre toxin-antitoxin loci knaAT are relatively widely present in the virulence plasmids of K. pneumoniae.
KnaAT encoded by the virulence plasmid is a typical type II TA module.
KnaAT enhances the stability of the mobilizable virulence plasmid in K. pneumoniae.
Introduction
Klebsiella pneumoniae, a Gram-negative pathogen, causes a wide range of hospital- and community-associated infections [Citation1]. K. pneumoniae infection results in high mortality rates and extended hospitalization, thereby burdening the healthcare sector [Citation2]. K. pneumoniae are generally classified as classical K. pneumoniae (cKP) and hypervirulent K. pneumoniae (hvKP) [Citation3]. cKP usually causes nosocomial infections and carries the plasmid(s) encoding for antimicrobial resistance (including carbapenem-resistant, CR), whereas hvKP is associated with hypermucoviscosity and hypervirulence phenotypes, and harbours a pLVPK-like mobilizable virulence plasmid that causes metastasis of severe infections, such as liver abscesses and bacteremia [Citation3]. Plasmid transfer and stability are vital for the bacteria to adapt and expand into new niches [Citation4, Citation5]. CR-KP and hvKP converge to form a hypervirulent carbapenem-resistant K. pneumoniae (hv-CRKP) strain or carbapenem-resistant hypervirulent K. pneumoniae (CR-hvKP) strain by acquiring resistance or virulence plasmids [Citation6–9]. However, little is known about the genetic stability of the virulence plasmid in K. pneumoniae.
Bacterial toxin-antitoxin (TA) modules comprise a toxin capable of arresting cell growth under certain conditions and an antitoxin capable of counteracting the toxic effect [Citation10, Citation11]; this has long been associated with plasmid maintenance and stabilization [Citation10, Citation12]. When a bacterium loses its plasmid, the TA modules may induce post- segregational killing (PSK), where progeny that does not retain the plasmid are eliminated [Citation10]. TA modules also aid bacterial pathogenicity by stabilizing virulence plasmids and ensuring the maintenance of virulence genes in the host [Citation12]. To date, TA modules have been classified into eight types (I-VIII) based on the nature and neutralization mechanisms of antitoxins [Citation13]. In the well-documented type II TA modules, both toxins and antitoxins are encoded by a bicistronic operon [Citation13–15]. The characteristics of a classical type II TA module include toxins that can suppress bacterial growth and even kill bacterial cells, antitoxins capable of neutralizing the toxin’s effect, and two proteins that are transcribed simultaneously [Citation13, Citation16]. The type II TA module RES-Xre consists of a toxin protein harbouring an arginine-glutamine-serine (RES) domain and an antitoxin protein carrying the N-terminal Xenobiotic Response Element (XRE) family helix-turn-helix (HTH) DNA-binding domain [Citation17]. It has recently been described in various bacterial species, including Sphingobium sp. YBL2 [Citation18], Mycobacterium tuberculosis [Citation19], Pseudomonas putida [Citation20, Citation21] and Pseudomonas resinovorans [Citation22]. Few functions ascribed to the RES-Xre TA pair include inhibiting the growth of Sphingobium sp. and P. putida [Citation18, Citation20, Citation21], reducing mycobacterial survival in macrophages and prolonging the survival of infected mice [Citation19], inciting solvent tolerance and regulating the stability of the megaplasmid in P. putida [Citation21] and conferring stability to a carbazole-degradative plasmid in P. resinovorans [Citation22]. However, whether the type II TA module affects the stability of the virulence plasmid in K. pneumoniae remains unclear.
Previously, we reported a bloodstream infection-causing hvKP isolate RJF293 (capsular serotype K2 and ST374), which contained a pLVPK-like 224-kb mobilizable virulence plasmid, pRJF293, belonging to the IncHI1B group [Citation7, Citation23]. In the present study, we characterized an instance of the RES-Xre TA pair, named KnaAT due to the capability of RES-domain toxins to deplete NAD+ in K. pneumoniae, and unveiled its association with maintaining the stability of the virulence plasmid. This study aimed to investigate the biological significance of the RES-Xre TA module and to contribute to a better understanding of the stability of large virulence plasmids in K. pneumoniae.
Materials and methods
Bioinformatics analysis
A total of 499 and 2,514 complete chromosomes and plasmid sequences of K. pneumoniae respectively, were downloaded from the NCBI RefSeq database in May 2020. The plasmids were assigned to three main types (virulence, antibiotic resistance, and others) based on the plasmid cargo gene functions predicted by VRprofile2 [Citation24]. The K. pneumoniae plasmid carrying iuc (coding for aerobactin), iro (coding for salmochelin siderophore), rmpA, and rmpA2 (coding for regulators of capsule production) was identified as a virulence plasmid [Citation24], whereas the plasmid carrying vim, oxa, ndm, kpc, and/or imp genes was identified as an antibiotic-resistant gene-carrying (ARG-carrying) plasmid. The remaining plasmids with no virulent genes or ARGs were classified as “others” for statistical comparison.
A standalone version of the TAfinder software was used to search for the type II TA loci [Citation25]. The amino acid sequences of the RES-Xre TA pair were extracted from the search output. Multiple sequence alignments and percentage identity matrices were completed using Clustal Omega [Citation26] and visualized using the pheatmap function in RStudio (http://www.rstudio.com/). Subsequently, the maximum likelihood phylogenetic tree was constructed using the IQ-TREE software [Citation27] and visualized using the Interactive Tree of Life (iTOL) website [Citation28].
Bacterial strains and growth conditions
K. pneumoniae RJF293, K. pneumoniae RJF293ΔknaAT, Escherichia coli DH5α, E. coli C600, plasmid pBAD33, pET28a, and pBluescript SK + (pSK) used in this study used are listed in the Supplementary Table S4. The strains and mutants were grown in LB broth, supplemented with appropriate antibiotics, at 37 °C with shaking at 200 rpm, supplemented with appropriate antibiotics. For solid cultivation, the LB medium was supplemented with 1.5% agar and the appropriate antibiotics to prepare an LB agar plate. The agar plates were then incubated at 37 °C overnight. The appropriate volumes of kanamycin (50 mg/mL), apramycin (50 mg/mL), chloramphenicol (50 mg/mL), hygromycin (200 mg/mL), glucose (2% m/v), arabinose (2% m/v), and IPTG (1 M) were added to either LB broth or LB agar to grow bacteria when required.
Plasmid construction
The plasmids for the phenotypic assays in E. coli DH5α were constructed by cloning the RES toxin gene (knaT) and Xre antitoxin gene (knaA) into the plasmid pBAD33 and pET28a, respectively. knaT and knaA were amplified from the genomic DNA of the strain RJF293 [Citation23]. knaT was cloned into the pBAD33 plasmid to produce pBAD33-KnaT, and KnaT expression was under the control of the PBAD promoter. Similarly, knaA was cloned into the pET28a plasmid to produce pET28a-KnaA, and KnaA expression was under the control of the PLac promoter. Further, 0.2% arabinose and 1 mM IPTG were used to activate the expression of these genes. In contrast, 0.2% glucose was used to repress the expression of these genes as a control. Finally, the generated plasmids were co-transformed into E. coli DH5α to perform growth and toxicity neutralization assays, respectively. All primers used in this study were synthesized by Sangon Biotech (Shanghai, China) and are listed in Supplementary Table S4.
Strain construction
All mutant strains generated from hvKP RJF293 were constructed using lambda red recombination and a flippase recombinase-based recombination approach, as described previously [Citation29, Citation30]. Briefly, two flanking fragments (500 bp) of the target gene and a hygromycin resistance cassette (hph) were amplified using the designed primers listed in Table S4 with the BsmBI restriction site. The generated PCR products were subsequently purified and used in a one-step golden gate to join the three fragments together after the purification. The joined fragments were used to replace the target gene or operon via homologous recombination. The plasmid pKOBEG [Citation31] containing the lambda red recombination system was induced with 0.2% arabinose to activate the homologous recombination. The transformants were selected using 200 μg/mL hygromycin and then checked via Sanger sequencing. The inserted hph was removed using the pFLP2 plasmid containing a flippase recombinase-based recombination system. The generated transformants were selected using 50 μg/mL apramycin and confirmed via PCR. The generated mutants were used for the plasmid stability and growth assays.
Growth assay and NAD+ measurement
Overnight cultures of E. coli DH5α were grown in LB broth supplemented with the appropriate antibiotics and 0.2% glucose at 37 °C. For the spot plate assay, the overnight cultures were diluted 1:100 in fresh LB broth supplemented with the appropriate antibiotics and 0.2% glucose. The diluted cultures were grown at 37 °C up to the mid-log phase. Subsequently, the regrown cultures were serially diluted (100–105). Five microliters of each dilution were spotted on LB agar plates containing the appropriate antibiotics with 0.2% arabinose (w/v) and 1 mM IPTG, or with 0.2% glucose (w/v). LB agar plates were incubated overnight at 37 °C. For the growth curve assay, overnight cultures were diluted to a final optical density 600 (OD600) of 0.01 in fresh LB broth containing the appropriate antibiotics. The diluted cultures were then moved to a 96-well microtiter plate and the OD600 was monitored at 10-min intervals by a Bioscreen C microplate absorbance reader for 7 h at 37 °C with shaking. The NAD+ assay was performed using the NAD+/NADH Colorimetric Assay Kit (Beyotime Biotechnology, Shanghai, China), according to the manufacturer’s instructions.
Co-transcription assay
Reverse transcription-polymerase chain reaction (RT–PCR) was performed to validate whether knaT and knaA were co-transcribed. Total RNA was extracted from hvKP RJF293 cells using the RNeasy Kit (Qiagen, Germany). The gDNA of the RNA sample was digested using DNase I. Subsequently, 500 ng of the RNA was converted to cDNA using PrimeScript™ RT Reagent Kit (Takara, Japan). Different primers ( and Table S4) were designed for the PCR amplification of cDNA, RNA, and gDNA.
Plasmid stability assay
The hvKP RJF293 harbouring a wild-type plasmid, pRJF293, or knaAT deletion plasmid, pRJF293ΔknaAT, was incubated on the LB agar plate containing 200 μg/mL hygromycin at 37 °C overnight. Thereafter, a single colony from each plate (wild-type and deletion mutant) was picked and cultivated overnight at 37 °C in 3 mL of LB broth supplemented with 200 μg/mL hygromycin. The overnight culture was then diluted in 1 mL of fresh LB broth without hygromycin and re-grown at 37 °C for 12 h. The 12-h incubation timeframe was estimated to pass 10 generations, and serial passaging was carried out every 12 h with a 1:1000 dilution ratio. To determine the plasmid loss, 100 μL of the cultures collected from the tube every 12 h was plated on fresh LB agar after serial dilution. A single colony was picked from the LB agar and subjected to replica plating by spotting the colony onto an LB agar plate with or without hygromycin. Finally, the colonies on both plates were counted. The plasmid-containing fraction was calculated as follows:
A similar procedure and calculation were performed in E. coli C600 by replacing pRJF293 with pSK::knaAT, pRJF293ΔknaAT with pSK and hygromycin with ampicillin. Data were pooled and presented every 50 generations for K. pneumoniae and every 20 generations for E. coli.
Animal experiments
A mouse lethality assay was performed to evaluate the hvKP strain RJF293 and its virulence plasmid-free derivative RJF293PF, following the protocol (A2022053) approved by the Animal Care and Ethics Committee at the Shanghai Institute of Immunity and Infection, CAS. The overnight culture of the K. pneumoniae strains under examination was inoculated into fresh LB broth at a ratio of 1:100 and incubated for 6 h in a shaking incubator at 37 °C. The cells were harvested, washed, and resuspended in 0.9% saline. Subsequently, RJF293 was diluted to the final concentrations of 5 × 103 and 5 × 104 CFU/mL, whereas RJF293PF was diluted to 5 × 103, 5 × 104, 5 × 105, and 5 × 106 CFU/mL. 100 μL of the bacterial suspension was intraperitoneally injected into a female 6-8-week-old BALB/c mouse. Each experimental group included 5 mice, with the 0.9% saline group serving as controls. All mice were monitored daily to assess survival rates. The Kaplan-Meier survival estimation was performed using the “survival” R package (https://www.r-project.org/).
Statistical analysis
The Shapiro–Wilk test was used to assess the normality of the data distribution. The Kruskal–Wallis one-way analysis of variance (ANOVA) test was used to calculate the mean differences between the different plasmid types (virulence, antibiotic resistance, and others). Dunn’s test, a nonparametric alternative to the Tukey test, was performed when the Kruskal–Wallis test was rejected to pinpoint the specific means that were significantly different from the others [Citation32]. The top six TA families containing non-zero mean values were selected for visualization. Subsequently, point-biserial correlation analysis was conducted to measure the strength of the association between the plasmid types and the status of the respective TA families. The point-biserial analysis is a specialized form of the Pearson correlation coefficient designed for situations in which one of the tested variables is dichotomous. Because the data type for the status of the TA families was dichotomous (either present or absent), point-biserial correlation analysis was chosen [Citation33]. All statistical analyses were performed and visualized using RStudio (http://www.rstudio.com/), and statistical significance was set at P < 0.001.
Results
Distribution of RES-Xre TA loci in the virulence plasmids of K. pneumoniae
A total of 364, 100, and 2,050 plasmids were classified as ARG-carrying, virulent, and other plasmids, respectively. Of the 2,514 plasmids, 3,164 putative type II TA loci have been predicted [Citation34]. Kruskal–Wallis one-way ANOVA showed that, overall, virulence plasmids had significantly (P < 0.001) more TA loci than ARG-carrying and other plasmids (). A similar trend was observed for the TA loci carrying the toxin families of PIN, RES (KnaT), RelE, and GNAT (). The PIN (PilT N-terminus) toxin exhibits ribonuclease activity and is neutralized by its cognate ribbon-helix-helix (RHH) antitoxin [Citation35]. The RelE toxin inhibits protein synthesis by cleaving mRNAs, whereas its RNAse activity is counteracted by the cognate RelB antitoxin [Citation36]. The Gcn5-related N-acetyltransferase (GNAT) toxin inhibits translation by acetylating aminoacyl-tRNAs and its cognate antitoxin carries a RHH domain [Citation37]. Nevertheless, only the TA loci from the RES-Xre family showed a high correlation with the virulence plasmid when compared with ARG-carrying plasmids (P < 0.001, rpb = 0.715) and other plasmids (P < 0.001, rpb = 0.535) (Table S1).
Figure 1. Type II TA families predicted in different types of K. pneumoniae plasmids. Based on plasmid cargo gene functions, the plasmids were grouped into three types: virulence (n = 100), antibiotic-resistant (n = 364), and others (n = 2,050). The mean values of putative TA pairs for each category of plasmids were plotted with error bars. The P-values were denoted as “ns’ for not significant (P > 0.05), “*” for P < 0.05, “**” for P < 0.01, “***” for P < 0.001, and “****” for P < 0.0001, respectively. A P-value of < 0.001 was considered statistically significant in this study. Further details and analyses are available in Table S1.

Among 3,164 putative type II TA loci predicted in the plasmids, 6% (n = 176) were RES-Xre, which ranked it as the fifth most common type II TA family in the K. pneumoniae’s plasmids[Citation34]. The predominant sequence types (STs) of K. pneumoniae carrying the virulence plasmids were ST11 (n = 32) and ST23 (n = 20) (Table S2). Furthermore, 72% (71/100) of the virulence plasmids of K. pneumoniae carry the RES-Xre TA loci (Table S2). Compared to the other types of TA loci, the carrying rate of the RES-Xre locus among the virulence plasmids were relatively high. This ranked second after PIN (90%), followed by RelBE (59%), GNAT (27%), MazEF (11%), HigBA (8%), unknown TA family (5%), and HipBA (1%). In contrast, only ten RES-Xre TA loci were predicted on the chromosomes of K. pneumoniae (supplementary Figure S1).
According to TAfinder prediction [Citation25], the RES toxin protein carries either a RES or a smart00953 domain, whereas the Xre antitoxin protein only has the Xre domain (Table S3). The RES and smart00953 domains are the members of cl02411, a RES superfamily [Citation38], whereas the Xre domain belongs to the DUF2384 superfamily (cl17718), the function of which is unknown [Citation38]. We propose that the RES-Xre TA loci in K. pneumoniae should be named KnaAT because the capability of RES-domain toxins to deplete NAD+ and affect nucleotide biosynthesis [Citation18–21]. We subsequently selected the RES toxins encoded by the chromosomes FU841_RS24115 and BMF28_RS04575, and the plasmid KnaT of pRJF293 of K. pneumoniae to compare their gene orders, protein sequences and structures with those experimentally validated ( and supplementary Figure S1). The RES toxin BLASTp identities between K. pneumoniae and other species were low, ranging from 22.58% to 35.53% (B). The multiple sequence alignment of KnaT with other RES toxins elucidates conserved amino acid residues at active sites – Arginine (R), Glutamic Acid (E), and Serine (S) – consistent with previous reports (B and C) [Citation18–20]. Furthermore, KnaT exhibited structural similarity to ParT [Citation18], RES_Pputida [Citation20], and MbcT [Citation19], with a reported RMSD < 2.7 (supplementary Figure S2).
Figure 2. Sequence and Structure analysis of RES toxin protein KnaT of K. pneumoniae. (A) Genetic organization of the RES-Xre loci from K. pneumoniae (KnaAT of the strain RJF293, RMF28_RS04570-75 of P1428 and FU841_RS24115-20 of QD23) and other reported species (MbcAT of M. tuberculosis H37Rv, SlvAT of P. putida S12, RES-Xre_Pputida of P. putida KT2440 and ParST of Sphingobium sp. YBL2). (B) The multiple sequence alignment of RES toxin proteins. Residues with deeper colours indicate higher identities. The letters R, E and S indicate the conserved amino acid residues Arginine, Glutamic Acid and Serine, respectively, which were previously reported as active sites in the RES toxin. (C) The protein tertiary structure of KnaT archived in the AlphaFold Protein Structure Database (accession: A0A377ZUI9, chain A). Shown are the close-ups of putative active sites of KnaT (Arg-27, Glu-48, and Ser-115).

knaAT in the virulence plasmid of K. pneumoniae codes for a type II TA module
To explore the knaAT operon (RJF2_RS26210-15) in the virulence plasmid pRJF293 of the hvKP RJF293 is a bona fide TA module, four E. coli DH5α derivatives [including pBAD33 + pET28a (vector control), pBAD33-KnaT + (toxin), pBAD33 + pET28a-KnaA (antitoxin) and pBAD33-KnaT + pET28a-KnaA (toxin + antitoxin)] were generated and used to perform the growth assay. The spot plate assay showed that arabinose and IPTG induction did not affect the growth of the vector control and antitoxin groups (A). The induction of KnaT significantly halted the growth of E. coli and its toxic effect could be neutralized by KnaA expression observed in the pBAD33-KnaT + pET28a-KnaA group. Subsequently, the growth curve assay showed that the expressed toxin KnaT stopped the growth of the strain after 110 min of induction, whereas the other three groups continued to grow (C). In addition, according to the spot plate assay result (supplementary Figure S3), the induced expression of KnaT repressed the growth of K. pneumoniae compared with RJF293ΔknaAT harbouring pBAD33 and RJF293ΔknaAT harbouring pBAD33-KnaAT. These results showed that the KnaA effectively restored the growth inhibition caused by toxin KnaT.
Figure 3. Growth assays to assess the toxicities of the RES toxin KnaT on E. coli. (A-B) Growth of the serially-diluted E. coli DH5α with different plasmid combinations. They were plated on LB agar complement with (A) 0.2% arabinose (w/v) and 1 mM IPTG or (B) 0.2% glucose (w/v). (C-D). Growth curves of E. coli DH5α with different plasmid combinations. (E) Genetic organization (i) and co-transcription (ii) of knaAT operon of K. pneumoniae RJF293. RT-PCR confirmed that the toxin knaT and antitoxin knaA are co-transcripted. (F) Measurements of cellular NAD+ levels in K. pneumoniae RJF293ΔknaAT expressing knaT, knaAT or empty pBAD33 vector. Data are presented as the mean ± standard deviation of three independent experiments.

RT–PCR analysis of the total RNA isolated from RJF293 cells showed that the knaAT gene pair was co-transcribed as a bicistronic operon (E). DNA bands covering knaA and knaT were detected, as was the case when the positive control genomic DNA was used as the template. In contrast, no band was obtained when negative control total RNA was used as the template (E). Overall, our data support the idea that knaA-knaT constituted a typical type II TA pair.
KnaT toxin arrests cell growth by depleting cellular NAD+
RES toxins reduce intracellular NAD+ level [Citation18–21]. To investigate the effect of the KnaT on the intracellular NAD+ content of K. pneumoniae, we separately measured the NAD+ levels of K. pneumoniae expressing knaT, knaAT, and empty pBAD33 vectors using the NAD+/NADH Colorimetric Assay Kit. Our results showed that the NAD+ level of K. pneumoniae expressing knaT was significantly reduced compared with the strains that harbour knaAT or empty vector (F).
KnaAT affects the virulence plasmid stability in K. pneumoniae
TA modules are closely related to the genetic stability of plasmids [Citation10, Citation12, Citation39], including those from the RES-Xre family [Citation22]. To investigate whether the knaAT operon is an addiction module that maintains plasmid stability, we assessed its role in the host plasmid, pRJF293. RJF293, which contains the knaAT locus on pRJF293, did not lose its plasmid even after the 400th generation (A). However, the RJF293 derivative that contains the knaAT-deleted plasmid (pRJF293ΔknaAT) loses its plasmid gradually, starting from the 200th generation.
Figure 4. The knaAT operon that maintains the stability of the virulence plasmid in K. pneumoniae. (A) hvKP strain RJF293 that carried its wild-type virulence plasmid pRJF293 or the knaAT deletion virulence plasmid pRJF293ΔknaAT was propagated in the antibiotic-free LB medium for 400 generations. The virulence plasmid-carrying mucoid phenotype regulator gene rmpA was used for control. Data are presented as the mean ± standard deviation of three independent experiments. (B) E. coli C600 that harboured pSK vector with or without the knaAT operon was passaged in the antibiotic-free LB medium for 100 generations. (C) The loss of virulence plasmid leads to a negative hypermucoviscosity phenotype of K. pneumoniae RJF293PF in comparison with the hvKP RJF293. (D) Kaplan-Meier survival curves generated for mice infected with the hvKP RJF293 and the virulence plasmid-free strain RJF293PF at various intraperitoneal concentrations. RJF293 exhibited statistically significant virulence compared to RJF293PF at dosages of 5 × 102 CFU (p = 0.0023) and 5 × 103 CFU (p = 0.0019). No mortality was observed among mice in either the RJF293PF or saline control groups throughout the seven-day observation period.

To confirm this observation in K. pneumoniae, we cloned the knaAT operon with its promoter and ribosome binding site into pSK (a plasmid with ampicillin resistance), resulting in pSK::knaAT. The first 100 generations of E. coli C600 containing the pSK::knaAT plasmid were examined by plating the cells on LB agar containing ampicillin. Consistent with the results for K. pneumoniae, we found that knaAT-carrying pSK remained more stable than pSK alone (B), further strengthening the finding that the knaAT operon enhance plasmid stability.
Loss of the virulence plasmid decreases the virulence of K. pneumoniae
We first performed a string test for hvKP RJF293 with a loss of virulence plasmid due to knaAT deletion. After overnight incubation on blood agar, a mucoviscous string over 4 cm in length was observed when lifting the wild-type RJF293 colony. However, the string test for the hypermucoviscosity phenotype of the virulence plasmid-free K. pneumoniae RJF293PF was negative (C).
The mouse lethality assay revealed that virulence plasmid-free K. pneumoniae RJF293PF exhibited a significant decrease in virulence compared to the hvKP strain RJF293 (D), particularly at inoculum levels of 5 × 102 CFU (p = 0.0023, determined by log-rank test) and 5 × 103 CFU (p = 0.0019, determined by log-rank test) in an intraperitoneally injected mouse model. Notably, no deaths were observed in the RJF293PF group, even at a dose of 5 × 105 CFU, over the seven-day observation period. These results suggested that knaAT contribute to the pathogenicity of hvKP by maintaining the stability of the virulence plasmid.
Discussion
K. pneumoniae is a reservoir of plasmids carrying various virulence and antibiotic resistance genes (ARGs). In this study we observed a disproportionate presence of the RES-Xre TA locus KnaAT in virulence plasmids (ranked second) of K. pneumoniae, despite it being the fifth most common type II TA family in the plasmids of this bacterium. Notably, the TA loci exhibited a higher correlation with virulence plasmids compared to ARG-carrying and other plasmids carrying neither ARG nor virulence genes. Moreover, our investigation revealed that knaAT displays characteristics typical of the RES-Xre TA loci. Overexpression of the RES toxin KnaT inhibits bacterial growth, and its cognate antitoxin KnaA effectively neutralizes these toxic effects. Additional experiments indicated that KnaAT plays a crucial role in maintaining the stability of virulence plasmids, thereby enhancing the likelihood of virulence gene transfer. Furthermore, the string test and mouse lethality assay underscored the significance of virulence plasmids in K. pneumoniae's pathogenicity.
Toxin-antitoxin loci were originally discovered to encode an “addiction module” that prevents plasmid loss from bacterial cultures. Notably, RES-Xre TA loci, such as slvAT and prcAT in P. putida S12 and Pseudomonas spp., respectively, have been found to regulate plasmid stability [Citation22]. Considering the correlation of the RES-Xre TA loci with the virulence plasmids of K. pneumoniae, we hypothesized that the RES-Xre TA loci on the pLVPK-like mobilizable virulence plasmid could influence the genetic stability of the virulence plasmid in K. pneumoniae (A and B). Interestingly, KnaAT appeared to maintain overall plasmid stability, regardless of the presence of plasmid-carrying virulence genes such as the mucoid phenotype regulator gene rmpA (A). However, except for the RES-Xre pair knaAT, the virulence plasmid pRJF293 encoded two other type II TA pairs (PIN-RHH and RelBE). Whether these two TA pairs are involved in the genetic stability of virulence plasmids requires further investigation. In addition, we have demonstrated that the virulence plasmid pRJF293, which hosts a knaAT TA locus, is crucial for K. pneumoniae to establish hypervirulence (D). The virulence plasmid-free hvKP strain (RJF293PF) was unable to kill the mice within seven days, even at an injection dosage of 5 × 105 CFU (D). Considering the relatively high correlation of RES-Xre TA loci with virulence plasmids and the critical role of virulence plasmid stability in establishing hypervirulence, KnaAT could be a potential therapeutic target for removing virulence plasmids from hvKP.
Recently, the ongoing evolution and acquisition of mobilizable virulence plasmids of hvKP have led to the development of CRKP strains that not only possess resistance but also retain hypervirulence, commonly referred to as hv-CRKP [Citation8, Citation24]. For example, the hv-CRKP strain 9949 has a pLVPK-like 237-kb virulence plasmid p9949_1 (NCBI accession: CP050281), which carries a RES-Xre locus, knaAT. Interestingly, the fusion of a p9949_1-like virulence plasmid with a p9949_2-like 185-kb antibiotic resistance plasmid (CP050282) results, in the formation of a 293-kb pCRHV-C2244 (MT644086) [Citation40]. pCRHV-C2244 is a hybrid plasmid encoding KPC and a virulence factor that carries a knaAT locus (DLNEJDNC_00203-04) that may maintain plasmid stability in ST11 hv-CRKP. In summary, our focus on understanding knaAT, a prevalent RES-Xre type II TA locus associated with the virulence plasmids hvKP and hv-CRKP, is crucial for unravelling the intricate relationships between the TA locus and plasmid stability in bacterial pathogens.
RES-Xre is a classical type II TA pair, with the RES toxin impeding bacterial growth through the depletion of cellular NAD+ and cognate Xre antitoxin neutralizing the toxic effect. The RES toxin MbcT of M. tuberculosis is an NAD+ phosphorylase, and its crystal structure shows that MbcT resembles secreted NAD + -dependent bacterial exotoxins, such as diphtheria toxin [Citation19]. The RES toxin ParT of Sphingobium is a mono-ADP-ribosyltransferase (mART) that specifically modifies phosphoribosyl pyrophosphate synthetase (Prs), an essential enzyme in nucleotide biosynthesis, and is conserved in all organisms [Citation18]. Moreover, the crystal structure of the intact P. putida RES-Xre TA complex reveals that the toxin core domain shows structural similarity to ADP-ribosylating enzymes, such as the diphtheria toxin, but has an atypical NAD + -binding pocket suggesting an alternative function [Citation20]. In this study, we observed that KnaT, which houses the conserved RES residues essential for toxicity, operates in a similar manner, leading to NAD+ degradation in K. pneumoniae cells. Growth and co-transcription assays reveal that KnaT toxicity was neutralized by the cognate Xre antitoxin, KnaA. KnaT shares conserved active-site residues (R, E, and S) and exhibits similar protein structures to reported RES toxins; however, the specific mechanism by which KnaT reduces NAD+ content remains to be characterized.
Summary
This study investigated the distribution and characteristics of RES-Xre TA loci in K. pneumoniae. The knaAT operon on the pLVPK-like virulence plasmid pRJF293 in hvKP RJF293 is a typical type II TA module. The RES toxin KnaT reduces the intracellular NAD+ content, and the knaAT operon significantly improves the genetic stability of the virulence plasmid of K. pneumoniae. Considering the role of KnaAT in preserving the stability of virulence plasmids during plasmid transfer and the association of virulence plasmids with hypervirulence, this study underscores the biological importance of the RES-Xre TA locus in K. pneumoniae.
Graphic_abstract20240107
Download PDF (296.3 KB)Supplementary_materials_R1_20240111
Download PDF (849.7 KB)Supplementary_Tables
Download Zip (100.9 KB)Acknowledgements
The graphical abstract was generated with the support of Biorender(BioRender.com).
Disclosure statement
No potential conflict of interest was reported by the author(s).
Additional information
Funding
References
- Navon-Venezia S, Kondratyeva K, Carattoli A. Klebsiella pneumoniae: a major worldwide source and shuttle for antibiotic resistance. FEMS Microbiol Rev. 2017;41(3):252–275. doi:10.1093/femsre/fux013
- Giske CG, Monnet DL, Cars O, et al. Clinical and economic impact of common multidrug-resistant gram-negative bacilli. Antimicrob Agents Chemother. 2008;52(3):813–821. doi:10.1128/AAC.01169-07
- Russo TA, Marr CM. Hypervirulent Klebsiella pneumoniae. Clin Microbiol Rev. 2019;32(3). doi:10.1128/CMR.00001-19
- Wein T, Hülter NF, Mizrahi I, et al. Emergence of plasmid stability under non-selective conditions maintains antibiotic resistance. Nat Commun. 2019;10(1):2595, doi:10.1038/s41467-019-10600-7
- Wyres KL, Holt KE. Klebsiella pneumoniae as a key trafficker of drug resistance genes from environmental to clinically important bacteria. Curr Opin Microbiol. 2018; 45:131–139.
- Yang X, Dong N, Chan EW, et al. Carbapenem resistance-encoding and virulence-encoding conjugative plasmids in Klebsiella pneumoniae. Trends Microbiol. 2021;29(1):65–83. doi:10.1016/j.tim.2020.04.012
- Xu Y, Zhang J, Wang M, et al. Mobilization of the nonconjugative virulence plasmid from hypervirulent Klebsiella pneumoniae. Genome Med. 2021;13(1):119, doi:10.1186/s13073-021-00936-5
- Gu D, Dong N, Zheng Z, et al. A fatal outbreak of ST11 carbapenem-resistant hypervirulent Klebsiella pneumoniae in a Chinese hospital: a molecular epidemiological study. Curr Opin Microbiol. 2018; 45:131–139.
- Tian D, Liu X, Chen W, et al. Prevalence of hypervirulent and carbapenem-resistant Klebsiella pneumoniae under divergent evolutionary patterns. Emerg Microbes Infections. 2022; 11(1):1936–1949.
- Harms A, Brodersen DE, Mitarai N, et al. Toxins, targets, and triggers: an overview of toxin-antitoxin biology. Mol Cell. 2018;70(5):768–784. doi:10.1016/j.molcel.2018.01.003
- Hayes F, Van Melderen L. Toxins-antitoxins: diversity, evolution and function. Crit Rev Biochem Mol Biol. 2011;46(5):386–408. doi:10.3109/10409238.2011.600437
- Lobato-Márquez D, Díaz-Orejas R, García-Del Portillo F. Toxin-antitoxins and bacterial virulence. FEMS Microbiol Rev. 2016;40(5):592–609. doi:10.1093/femsre/fuw022
- Jurėnas D, Fraikin N, Goormaghtigh F, et al. Biology and evolution of bacterial toxin-antitoxin systems. Nat Rev Microbiol. 2022;20(6):335–350. doi:10.1038/s41579-021-00661-1
- Leplae R, Geeraerts D, Hallez R, et al. Diversity of bacterial type II toxin-antitoxin systems: a comprehensive search and functional analysis of novel families. Nucleic Acids Res. 2011;39(13):5513–5525. doi:10.1093/nar/gkr131
- Page R, Peti W. Toxin-antitoxin systems in bacterial growth arrest and persistence. Nat Chem Biol. 2016;12(4):208–214. doi:10.1038/nchembio.2044
- Fraikin N, Goormaghtigh F, Melderen LV. Type II toxin-antitoxin systems: evolution and revolutions. J Bacteriol 2020;202(7):e00763–19. doi:10.1128/JB.00763-19
- Makarova KS, Wolf YI, Koonin EV. Comprehensive comparative-genomic analysis of type 2 toxin-antitoxin systems and related mobile stress response systems in prokaryotes. Biol Direct. 2009;4:19, doi:10.1186/1745-6150-4-19
- Piscotta FJ, Jeffrey PD, Link AJ. ParST is a widespread toxin-antitoxin module that targets nucleotide metabolism. Proc Natl Acad Sci USA. 2019;116(3):826–834. doi:10.1073/pnas.1814633116
- Freire DM, Gutierrez C, Garza-Garcia A, et al. An NAD(+) phosphorylase toxin triggers Mycobacterium tuberculosis cell death. Mol Cell. 2019;73(6):1282–1291.e8. doi:10.1016/j.molcel.2019.01.028
- Skjerning RB, Senissar M, Winther KS, et al. The RES domain toxins of RES-Xre toxin-antitoxin modules induce cell stasis by degrading NAD+. Mol Microbiol. 2019;111(1):221–236. doi:10.1111/mmi.14150
- Kusumawardhani H, van Dijk D, Hosseini R, et al. Novel toxin-antitoxin module SlvT-SlvA regulates megaplasmid stability and incites solvent tolerance in Pseudomonas putida S12. Appl Environ Microbiol. 2020;86(13). doi:10.1128/AEM.00686-20
- Takashima A, Kawano H, Ueda T, et al. A toxin–antitoxin system confers stability to the IncP-7 plasmid pCAR1. Gene. 2022;812:146068, doi:10.1016/j.gene.2021.146068
- Wang XL, Xie YZ, Li G, et al. Whole-Genome-Sequencing characterization of bloodstream infection-causing hypervirulent Klebsiella pneumoniae of capsular serotype K2 and ST374. Virulence. 2018;9(1):510–521. doi:10.1080/21505594.2017.1421894
- Tian D, Wang M, Zhou Y, et al. Genetic diversity and evolution of the virulence plasmids encoding aerobactin and salmochelin in Klebsiella pneumoniae. Virulence. 2021;12(1):1323–1333. doi:10.1080/21505594.2021.1924019
- Xie Y, Wei Y, Shen Y, et al. TADB 2.0: an updated database of bacterial type II toxin-antitoxin loci. Nucleic Acids Res. 2018;46(D1):D749–D753. doi:10.1093/nar/gkx1033
- Sievers F, Higgins DG. Clustal Omega for making accurate alignments of many protein sequences. Protein Sci. 2018;27(1):135–145. doi:10.1002/pro.3290
- Minh BQ, Schmidt HA, Chernomor O, et al. IQ-TREE 2: new models and efficient methods for phylogenetic inference in the genomic era. Mol Biol Evol. 2020;37(5):1530–1534. doi:10.1093/molbev/msaa015
- Letunic I, Bork P. Interactive Tree of Life (iTOL) v5: an online tool for phylogenetic tree display and annotation. Nucleic Acids Res. 2021;49(W1):W293–W296. doi:10.1093/nar/gkab301
- Qian HL, Yao QQ, Tai C, et al. Identification and characterization of acetyltransferase-type toxin-antitoxin locus in Klebsiella pneumoniae. Mol Microbiol. 2018;108(4):336–349. doi:10.1111/mmi.13934
- Bi DX, Jiang XF, Sheng ZK, et al. Mapping the resistance-associated mobilome of a carbapenem-resistant Klebsiella pneumoniae strain reveals insights into factors shaping these regions and facilitates generation of a ‘resistance-disarmed’ model organism. J Antimicrob Chemoth. 2015;70(10):2770–2774. doi:10.1093/jac/dkv204
- Chaveroche MK, Ghigo JM, d’Enfert C. A rapid method for efficient gene replacement in the filamentous fungus Aspergillus nidulans. Nucleic Acids Res. 2000;28(22):e97. doi:10.1093/nar/28.22.e97
- Dinno A. Nonparametric pairwise multiple comparisons in independent groups using Dunn’s test. Stata J. 2015;15(1):292–300. doi:10.1177/1536867X1501500117
- Bonett DG. Point-biserial correlation: interval estimation, hypothesis testing, meta-analysis, and sample size determination. Br J Math Stat Psychol. 2020;73(Suppl 1):113–144. doi:10.1111/bmsp.12189
- Goh YX, Li P, Wang M, et al. Comparative analysis of diverse acetyltransferase-type toxin-antitoxin loci in Klebsiella pneumoniae. Microbiol Spectr. 2022;10(4):e0032022.
- Arcus VL, McKenzie JL, Robson J, et al. The PIN-domain ribonucleases and the prokaryotic VapBC toxin-antitoxin array. Protein Eng Des Sel. 2011;24(1-2):33–40. doi:10.1093/protein/gzq081
- Pedersen K, Zavialov AV, Pavlov MY, et al. The bacterial toxin RelE displays codon-specific cleavage of mRNAs in the ribosomal a site. Cell. 2003;112(1):131–140. doi:10.1016/S0092-8674(02)01248-5
- Qian H, Yu H, Li P, et al. Toxin-antitoxin operon kacAT of Klebsiella pneumoniae is regulated by conditional cooperativity via a W-shaped KacA-KacT complex. Nucleic Acids Res. 2019;47(14):7690–7702. doi:10.1093/nar/gkz563
- Lu S, Wang J, Chitsaz F, et al. CDD/SPARCLE: the conserved domain database in 2020. Nucleic Acids Res. 2020;48(D1):D265–D268. doi:10.1093/nar/gkz991
- Qi Q, Kamruzzaman M, Iredell JR. The higBA-type toxin-antitoxin system in IncC plasmids is a mobilizable ciprofloxacin-inducible system. Msphere. 2021;6(3):e0042421. doi:10.1128/mSphere.00424-21
- Jin L, Wang R, Gao H, et al. Identification of a novel hybrid plasmid encoding KPC-2 and virulence factors in Klebsiella pneumoniae sequence type 11. Antimicrob Agents Chemother. 2021;65(6):e02435–20. doi:10.1128/AAC.02435-20