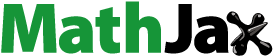
Abstract
In this study, Na-ZrO2 was prepared from two types of ZrO2 as solid base catalyst and the catalytic activity, selectivity, and mechanism of Na-ZrO2 for base catalyzed reactions in subcritical water were elucidated using aldol condensation between acetophenone and benzaldehyde as a model reaction. Based on the different selectivity for Cannizzaro reaction of benzaldehyde, a side reaction accompanied with the aldol condensation, two plausible mechanisms were proposed. One was that the doped Na2O could directly act as a base, deprotonating the acetophenone for catalyzing aldol condensation, and the other was that the doped Na2O could dissociate the water to provide OH− for catalyzing both aldol condensation and Cannizzaro reaction. The dominant mechanism varied depending on the nature of the ZrO2 support. The effect of the amount of subcritical water was also examined, showing that as reducing the loading of water, the yield of byproduct from the Cannizzaro reaction decreased due to the lower amount of dissociated OH−. On the other hand, the presence of water adversely affected the reaction equilibrium, but meanwhile sufficient water in the system also prevented the carbonization of organics and polymerization reaction on the catalyst surface, favoring for a ring balance.
1. Introduction
Subcritical water, defined as water at a temperature above the normal boiling point but below the critical point (374 °C), while maintaining in the liquid state under high pressure, has attracted much attention as an alternative medium for organic synthesis because of the rising concerns about the negative impact which organic solvents have on the environment (Ko et al. Citation2020). Subcritical water exhibits drastically different physicochemical properties with water in ambient condition (Kruse and Dinjus Citation2007). The lower dielectric constant and higher ion product (KW = [H+][OH−]) of water at a subcritical state facilitates the dissolution of organic compounds and makes it suitable for acid/base catalyzed reactions (Iwamura et al. Citation2014; Savage Citation1999). In the past, the feasibility of conducting organic reactions in subcritical water without catalysts was mainly investigated, and major types of reactions had been reviewed by several researchers (Iwamura et al. Citation2014; Kruse and Dinjus Citation2007; Kus Citation2012; Savage Citation1999). However, the limitation of the acid/base catalytic activity of subcritical water was also distinct.
To realize the enhancement and control of reactions in subcritical water while further reducing the generation of waste salts, the application of solid base catalysts for reactions in subcritical water had been studied and proved to be practical to achieve higher reaction rates, which could not be achieved by utilizing the acid/base catalytic activity of subcritical water alone (Akizuki and Oshima Citation2018). Investigation of the basicity and catalytic mechanism of solid catalysts was important to control the reactions in water at high temperature and pressure since water with unique properties at such conditions would affect the base catalytic properties on the catalyst surface. Watanabe and his group had investigated the acidity and basicity of some metal oxides (CeO2, MoO3, TiO2, and ZrO2) in supercritical water at 400 °C and 25–40 MPa using formaldehyde reaction as a test reaction (Watanabe et al. Citation2003). They elucidated the order of the basicity is CeO2 > ZrO2 > MoO3 > TiO2(rutile) > TiO2(anatase) and mentioned that the acidity and basicity on a metal oxide surface in supercritical water are probably due to adsorption of dissociated water molecules on the catalyst surface. Akizuki and his colleague studied the kinetics of ZrO2 catalyzed amide hydrolysis in subcritical water at 240–360 °C and 10–41 MPa, in which hydrolysis of amide was considerably promoted due to the basicity of ZrO2 (Akizuki and Oshima Citation2015). They found that compared with the reaction with NaOH as a homogeneous base catalyst, amino groups might hardly form hydrogen bond with water due to steric hindrance near the ZrO2 surface, thus affecting the reaction rate. However, these kinds of mechanism research were still limited and further investigation in the basicity and catalytic mechanism particularly for the supported catalyst, which was expected to exhibit higher basicity than simple metal oxide investigated before, would be necessary for controlling organic synthetic reactions.
Therefore, in this study, the basicity of ZrO2 would be enhanced by incorporating with active sodium compounds and the catalytic performance of such Na-ZrO2 in subcritical water would be evaluated. Crossed aldol condensation of acetophenone and benzaldehyde (Scheme 1) was used as a model of base catalyzed reaction. Since side reactions such as the Cannizzaro reaction of two benzaldehydes (Scheme 2), which was also base catalyzed, might occur, the selectivity of the reaction was expected to provide a deeper insight of base catalytic properties. We hope to elucidate the catalytic mechanisms of ZrO2 based catalysts in subcritical water, quantitatively analyze the catalytic activity and selectivity by establishing kinetic models and clarify how the amount of subcritical water affected the reaction.
2. Experimental
2.1. Materials
All solvents and chemicals were commercially available and used without further purification. Acetophenone, benzaldehyde, chalcone, benzyl alcohol, sodium nitrate and sodium hydroxide were purchased from FUJIFILM Wako Pure Chemical Industries, Ltd. Zirconium oxide, zirconium hydroxide, and acetonitrile were offered by Sigma-Aldrich. Dihydrochalcone was provided by Tokyo Chemical Industry Co., Ltd. Distillation water was prepared by water distillation apparatus, RFD240NC, produced by ADVANTEC Toyo Kaisha, Ltd.
2.2. Preparation of catalysts
Commercial ZrO2 and ZrO2 prepared by calcination of Zr(OH)4 at 500 °C for 3 hours were named as ZrO2 (type I) and ZrO2 (type II), respectively. Na-ZrO2 (type I and type II) were prepared by wet impregnation from their respective ZrO2 (type I and type II), which followed a procedure as listed below. The mixture of ZrO2 (type I or type II) and NaNO3 aqueous solution was stirred for overnight at ambient condition, followed by condensation with rotary evaporation under reduced pressure at 50 °C and drying at 100 °C for 1 h. The resulting solid was then calcined in a muffle furnace at 500 °C for 3 h under a standard atmosphere. In this research, Na-ZrO2 was prepared with 5.0 g of ZrO2 and 0.5 g of NaNO3.
2.3. Experimental procedures
To A mixture of acetophenone (4.3 mmol) and benzaldehyde (4.3 mmol) with distillation water in a 10 mL of 1/2-inch SS316 stainless steel tube batch reactor (Swagelok Co., tube length: 122 mm, outside diameter: 12.7 mm, thickness: 1.24 mm, fitted with a SS316 1/2-inch cap), 250 mg of solid catalyst was added, purging with nitrogen, preventing the oxidation of benzaldehyde to form benzoic acid with oxygen from the air. The reactor was then sealed and heated to 250 °C in sand bath and reacted for a range of time, from 0.5 h to 6 h. After the reaction, the reactor was cooled down to ambient temperature in a water bath and the reaction mixtures were diluted with acetonitrile, accompanied by the filtration of the catalysts. The filtrate was then subjected to GC analysis for identification of the product and determination of the yield and conversion.
2.4. Analysis
Quantitative analysis of the products was performed by using gas chromatograph-flame ionization detector (GC-2014, Shimadzu Corp.), equipped with a capillary column (GL Sciences, Inc., TC-1701, length: 30 m, film thickness: 0.25 μm, inside diameter: 0.25 mm). The initial temperature was set as 80 °C, holding for 25 min, and then the temperature was increased to 260 °C with a rate of 30 °C per min, holding at 260 °C for another 34 min. Identification of the side products was performed by gas chromatography–mass spectrometry (GC-2010, Shimadzu Corp.), equipped with the same capillary column as described above. Blank experiments were also performed to confirm that aldol condensation between acetophenone and benzaldehyde in acetonitrile would not take place in the GC system.
2.5. Characterization of catalysts
The crystal structure of the different catalysts was analyzed by a Rigaku SmartLab 9 kW X-ray diffractometer (XRD) using Cu-Kα radiation, wavelength of 0.154 nm, in the 2θ range from 10° to 90° with a scanning spend of 4° per minute and a step of 0.02 at room temperature.
The measurements of specific surface areas of the catalysts were performed based on the BET method in NOVA 2200e. Prior to the measurement, the samples were degassed at 200 °C for 2 hours and N2 adsorption/desorption isotherms were operated at −196 °C.
Temperature-programmed desorption with CO2, CO2-TPD, was applied to measure the base strength of the catalysts. 200 mg of catalyst were enclosed in a quartz tube, treated with a flow of 50 mL per min of Helium gas, while heating at a rate of 10 °C per min to 700 °C and holding at 700 °C for 1 h. After cooling down to 50 °C with helium gas flowing, the sample was saturated with 10% CO2/He mixture gas in a flow rate of 20 mL per min for 1 h. The sample was then purged with helium gas for an additional 1 h to remove physically adsorbed CO2. Finally, heating the samples to 700 °C with a ramp of 10 °C per min, and the desorbed CO2 was monitored with a thermal conductivity detector (TCD) and quantified with a TCD calibration curve.
To measure the quantity of sodium cation doped on ZrO2, the catalyst was treated by acid digestion in 66% H2SO4 acid at 200 °C overnight, followed by dilution for inductively coupled plasma-atomic emission spectroscopy (ICP-AES) analysis.
3. Results and Discussion
3.1. Characterization of catalysts
The XRD patterns of Zr(OH)4, ZrO2 and Na-ZrO2 were shown in . presented that ZrO2 (type II), obtaining from calcination of amorphous Zr(OH)4, contained both monoclinic and tetragonal phases, while ZrO2 (type I) contained only a monoclinic phase with higher crystallinity. However, a transformation of the tetragonal phase to the monoclinic phase was observed after modification of ZrO2 (type II), which was in agreement with the previous literature (Eom et al. Citation2015), while in the case of ZrO2 (type I), the modification did not lead to any phase changes. The detection of the peak of NaNO3 implied the existence of bulk-phase NaNO3 on Na-ZrO2, but didn’t show the presence of Na2O, indicating the formation of amorphous Na2O or the presence of dispersed Na2O undetectable by XRD (Aguila et al. Citation2016; Liu et al. Citation1998).
Figure 1. XRD patterns of catalysts. (a) ZrO2 (Type I), (b) Na-ZrO2 (Type I), (c) ZrO2 (Type II), (d) Na-ZrO2 (Type II).

The nomenclatures of the catalysts involved in this study and the corresponding physicochemical properties were summarized in , in which ZrO2 (Type II) presented much higher specific areas than ZrO2 (type I). In the case of the Na-ZrO2, compared with ZrO2, a significant decrease of the specific area was observed, probably being attributed to that the pores were covered by sodium compounds. To accurately measure how much Na+ was doped on the support, the fresh catalysts were rinsed with ambient water to eliminate the extra bulk phase NaNO3. With the removal of the undecomposed NaNO3, ICP analysis was performed for the Na-ZrO2, indicating 2.72 mol% and 2.93 mol% of Na+ was doped on the surface of ZrO2 (type I) and ZrO2 (type II) respectively.
Table 1. Physicochemical properties of the catalysts.
CO2-TPD was utilized to investigate the amount and the strength of basic sites of the catalysts. To accurately measure the basicity of Na-ZrO2, the same rinsing treatment was performed to remove the extra bulk phase NaNO3 covered on the ZrO2 support, followed by drying at 110 °C overnight before subjecting to CO2-TPD analysis. The amounts of basic sites were given in , and the desorption profiles were depicted in . According to the desorption temperature of CO2, the strength of basic sites could be roughly classified into four types, weak basic sites, (< 250 °C, I), medium basic sites (250–400 °C, II), strong basic sites (400 °C < 620 °C, III) and super strong basic sites (> 620 °C, IV) (Li et al. Citation2021). The profile of ZrO2 (type I) barely revealed a weak desorption peak below 250 °C, whereas the profile of ZrO2 (type II) showed that ZrO2 (type II) possessed medium and strong basic sites, along with a strong desorption peak in the range of weak basics sites. In the case of Na-ZrO2, compared with ZrO2, the profile presented prominent desorption peaks in the range of medium and strong basic sites with increased intensity, indicating an increased basicity of ZrO2 after modification. The generation of strong basic sites on ZrO2 was assumed to be primarily attributed to the formation of Na2O on the surface of ZrO2 during heat treatment (Liu et al. Citation2007).
3.2. Catalytic activities of ZrO2 and Na-ZrO2 in subcritical water
Initially, to investigate the catalytic activities of ZrO2 and Na-ZrO2 in subcritical water, the reaction was carried out with 3.0 g of distillation water under a condition mentioned in the procedures. To eliminate the possibility that the reaction was catalyzed by the undecomposed NaNO3, both the rinsed and fresh Na-ZrO2 were tested. Na-ZrO2 with rinsing treatment didn’t show a different catalytic performance with the fresh one ().
Figure 3. Yield of chalcone with and without catalysts at 250 °C vs. time. (■) Catalyst free, (●) ZrO2 (Type I), (▲) ZrO2 (Type II), (▼) Na-ZrO2 (Type I), (♦) Na-ZrO2 (Type II), (★) Na-ZrO2 (Type I) with rinsing treatment.

Chalcone was the major product produced from aldol condensation, and the yield of chalcone was quantitatively determined for the evaluation of the catalytic activity. Benzyl alcohol and benzoic acid were the major side products, yielded from the Cannizzaro reaction of benzaldehydes exhibited in Scheme 2. The catalytic selectivity for the Cannizzaro reaction was calculated based on the yield of benzyl alcohol, which was primarily monitored for its higher FID sensitivity. One minor side product, 1,3,5-triphenylpentane-1,5-dione, shown in Scheme 3, obtained from the Michael addition of acetophenone to chalcone, was traced by GC-MS. Other minor side products, such as dihydrochalcone, produced from the hydrogenation of double bond between the aliphatic carbons (Comisar and Savage Citation2004), were only in trace amounts.
and presented the yield of chalcone and benzyl alcohol with and without catalysts depending on time, respectively. The comprehensive experimental results were summarized in Tables S1–S5 in the supplementary materials. The uncertainty was experimentally determined by calculating the standard deviation. In the case of catalyst free, the yield of chalcone increased slowly with respect to the reaction time, and the benzyl alcohol was only traced. The reaction catalyzed by ZrO2 (type I) demonstrated that ZrO2 (type I) did not show clear catalytic activity for either aldol condensation or Cannizzaro reaction. On the other hand, the reaction catalyzed by ZrO2 (type II) showed a higher yield of both chalcone and benzyl alcohol.
Figure 4. Yield of benzyl alcohol with and without catalysts at 250 °C vs. time. (■) Catalyst free, (●) ZrO2 (Type I), (▲) ZrO2 (Type II), (▼) Na-ZrO2 (Type I), (♦) Na-ZrO2 (Type II).

The reactions catalyzed by Na-ZrO2 (type I and II) had a considerable increase in the yield for chalcone. However, Na-ZrO2 (type II) revealed significantly higher selectivity for Cannizzaro reaction to form benzyl alcohol than Na-ZrO2 (type I). A close highest yield of chalcone in 3 hours was likely indicating the aldol condensation reached an equilibrium.
To compare the catalytic performance of ZrO2 based catalysts with that of homogeneous catalyst in subcritical water, the reaction with 0.1 equivalent of NaOH was also carried out. showed the yield and conversion with respect to time. The detailed data was summed up in Table S6 in the supplementary materials. The production of chalcone and benzyl alcohol was considerable, indicating both aldol condensation and Cannizzaro reaction were promoted by strong OH− provided by NaOH.
3.3. Kinetics analysis with model and proposed mechanisms of ZrO2 based catalysts
To investigate the difference in the reaction rate quantitatively, kinetic analysis was conducted for reactions under different catalytic conditions. The reaction network involved in this system was presented in . Acetophenone (A) reacted reversibly with Benzaldehyde (B) to form Chalcone (C). Chalcone reacted further to form other products, such as 1,3,5-triphenylpentane-1,5-dione, dihydrochalcone and char. The Cannizzaro reaction of benzaldehyde formed benzyl alcohol (Bol) and benzoic acid. Based on this network with assumptions of first order for each reactant (second order for benzaldehyde in Cannizzaro reaction), the differential equations of concentrations depending on time during the reaction in a constant volume batch reactor were shown from EquationEqs. (1)–(4), which provided a mathematical model for this chemical reaction system. Since the amount of water did not change appreciably in the system compared with that of reactants and products, the concentration of water was assumed to be constant as 44.4 mol/L during the reaction (the density of subcritical water at 250 °C, saturated, was 799 g/L (Wagner and Pruß Citation2002). For the reason that 1,3,5-triphenylpentane-1,5-dione only became considerable when the loaded water was below 0.5 g, the rate constant k4 was supposed to be 0 when the system contained 1.0 g of water or more. To solve the differentia equations and estimate the kinetic rate constants, Python was used to perform the fitting of a system of ordinary differential equations.
(1)
(1)
(2)
(2)
(3)
(3)
(4)
(4)
Figure 6. Reaction network for the synthesis of chalcone via aldol condensations in subcritical water.

The experimental data of concentration with respect to time with models for reactions under different catalytic conditions were shown in . The estimated values of rate constants were summarized in . Since the reactions catalyzed by Na-ZrO2 better expressed a reaction equilibrium from the figures, the equilibrium constant, k1f/k1r, was derived from the reaction catalyzed by Na-ZrO2 via fitting to be 41.6, and such value was used to substitute into the rate constant for k1r as k1f/41.6 to fit the rate equation for other cases.
Figure 7. Comparison of model and experimental data for reaction in 3.0 g of water. (a) Catalyst free, (b) ZrO2 (Type I), (c) ZrO2 (Type II), (d) Na-ZrO2 (Type I), (e) Na-ZrO2 (Type II), (■) Chalcone, (●) Benzyl alcohol, (▲) Acetophenone, (▼) Benzaldehyde, (—) Model with R-squared values shown on the top-right corner.

Table 2. Estimated values of rate constants for reaction in 3.0 g of water.
To explain the discrepancy of catalytic activities, the mechanism of the basic model was proposed as . The reaction catalyzed by NaOH () provided evidence that the presence of strong OH− in subcritical water would induce both the aldol condensation and the Cannizzaro reaction. The larger values of k1f and k2 in the case of the ZrO2 (Type II) than ZrO2 (Type I) indicate that OH− on the ZrO2 (Type II) surface showed basicity. The CO2-TPD profiles suggested that the basic sites of ZrO2 (Type II) were much stronger than those of ZrO2 (Type I) in the gas phase, thus being more capable of dissociating the water into H+ and OH− on the catalyst surface in subcritical water.
In the case of Na-ZrO2 (both type I and II), the rate constant for the aldol condensation (k1f) was 4 times larger than that catalyzed by respective ZrO2 (type I and II) while the rate constant for the Cannizzaro reaction (k2) was 2 times larger than that catalyzed by respective ZrO2 (type I and II). Because k1f was increased rather than k2 by impregnation of Na species, there should be another mechanism to mainly promote aldol condensation. A plausible explanation composed of two different mechanisms of the basicity model in subcritical water are shown in . One mechanism was that the doped Na2O could directly act as a base, deprotonating the acetophenone for catalyzing aldol condensation, and the other was that the OH− provided by water dissociation catalyze both aldol condensation and Cannizzaro reaction. When comparing Na-ZrO2 (type I) with Na-ZrO2 (type II), Na-ZrO2 (type II) considerably promoted the Cannizzaro reaction than Na-ZrO2 (type I), which could be explained by the fact that ZrO2 (type II) support itself had high ability to provide OH−. Solid base catalyzed reactions in subcritical and supercritical water were considered to be promoted by OH− provided by the water dissociation (Akizuki and Oshima Citation2015; Watanabe et al. Citation2003) since the surface of catalysts are surrounded by high density water, but the possibility of contributing another kind of basic site even in subcritical water having high ion product was an interesting finding.
3.4. Effect of amount of water
To further investigate the effect of subcritical water on solid base catalysis, the effect of the amount of solvent water, in the reactor with a volume of 10 mL, was investigated by reducing from 3.0 g (condition used for the experiments above) to 0 (water free). The reaction was catalyzed by Na-ZrO2 (type I) for 3 h, and the results were depicted in .
Figure 9. Yield and conversion after 3.0 h reaction at 250 °C with Na-ZrO2 (type I) vs. the amount of water in 10 mL reactor. Yield: (■) Chalcone, (●) Benzyl alcohol, Conversion: (▲) Acetophenone, (▼) Benzaldehyde.

As the amount of loaded water decreased from 3.0 g to around 1.0 g, the yield of chalcone gradually increased, while the yield of benzyl alcohol slightly decreased. showed the time dependence of the yield of chalone and benzyl alcohol. The equilibrium yield of the chalcone when the amount of water was 1.0 g was larger than that when the amount of water was 3.0 g (). Since the equilibrium of aldol condensation shifted to the product side in low water concentration condition (Scheme 1), more benzaldehyde was available for aldol condensation and the yield of chalcone increased but that of benzyl alcohol decreased. To further confirm this, the kinetic model with the rate constants from was applied for this condition. showed the predicted values which generally matched the experimental results, indicating that the reaction mechanism was not changed as the amount of water decreased from 3.0 g to 1.0 g.
Figure 10. Yield of chalone and benzyl alcohol of reaction with 1.0 g of water in 10 mL reactor at 250 °C with Na-ZrO2 (type I) vs. time. Chalcone: (■) Chalcone, (●) Benzyl alcohol.

Figure 11. Comparison of predicted model and experimental data for reaction with 1.0 g of water in 10 mL reactor. (a) Na-ZrO2 (Type I), (■) Chalcone, (●) Benzyl alcohol, (▲) Acetophenone, (▼) Benzaldehyde, (—) Model.

On the other hand, as the amount of water decreased from 1.0 g to 0 (water free), the yield of benzyl alcohol continued to decline and reached practically 0 in the case of water free. It could be explained that less water in presence led to a lower amount of dissociated OH− on the catalyst surface, thus inhibiting the Cannizzaro reaction. In the case of water free, there would be no dissociated OH− on the catalyst surface and the catalytic mechanism could be the doped Na2O on the ZrO2 support directly function as a base, deprotonating the acetophenone for catalyzing aldol condensation. In addition, under a condition where the amount of water was below 1.0 g, the conversion of acetophenone was higher than benzaldehyde, indication that the formation of 1,3,5-triphenylpentane-1,5-dione and further products became noticeable. Furthermore, from Table S7 in the supplementary material, there is an obvious decreasing trend of the ring balance between the reactant conversion and product yield. Also, Figure S1, given in the supplementary materials, demonstrated a darkening trend of the reaction mixtures as reducing the amount of water involved in the reaction. Further investigation of the mechanism was necessary, but these results suggested that sufficient water could inhibit the polymerization reaction on the catalyst surface.
4. Conclusions
The basicity and catalytic mechanism of ZrO2 and Na-ZrO2 in subcritical water at 250 °C were investigated using aldol condensation of acetophenone and benzaldehyde and Cannizzaro reaction of two benzaldehyde as model reactions.
Among the two ZrO2 (Type I and II) catalyst, ZrO2 (Type II) showed higher base catalytic activity because stronger basic site on ZrO2 (Type II) promote water dissociation in subcritical water and produced OH− on the surface showed base catalytic activity.
On the other hand, for the Na-ZrO2 (Type I and II) catalyst, the existence of two catalytic mechanisms was suggested; one was that the doped Na2O could directly act as a base, and the other was that OH− provided by the dissociation of water act as a base. Since ZrO2 (type II) support itself had a high ability to provide OH−, Na-ZrO2 (type II) considerably promoted the Cannizzaro reaction while the selectivity for aldol condensation was high for Na-ZrO2 (type I) catalyst. The presence of other type of basic site than the OH− provided by the dissociation of water even in subcritical water having high ion product, and that their dominance can be changed depending on the catalyst support, is important from the viewpoint of control in reactions in subcritical water.
The effect of the amount of solvent water suggested that reducing the water loading from 3.0 g to 1.0 g in a 10 mL reactor did not cause a change in the catalytic mechanism. On the other hand, when the amount of water decreased from 1.0 g to 0, less water in presence lead to a lower amount of dissociated OH− on the catalyst surface. In addition, sufficient water might be able to inhibit the polymerization reaction on the catalyst surface.
Supplemental Material
Download MS Word (115.3 KB)Acknowledgement
BET measurements were performed at Otomo lab, School of Environment and Society, Tokyo Institute of Technology. XRD and ICP analysis were performed at the Institute for Solid State Physics, The University of Tokyo. The GC-MS analysis was conducted at Kashiwa Branch, Environmental Science Center and Tonokura lab, The University of Tokyo.
No potential conflict of interest was reported by the author(s).
Disclosure statement
Additional information
Funding
References
- Aguila G, Salinas D, Jiménez R, Guerrero S, Araya P. 2016. ZrO2-supported alkali metal (Li, Na, K) catalysts for biodiesel production. J Chil Chem Soc. 61:3233–3238. doi: 10.4067/S0717-97072016000400017.
- Akizuki M, Oshima Y. 2015. Kinetics of N-substituted amide hydrolysis in hot compressed water using ZrO2 catalyst. Ind Eng Chem Res. 54:3611–3617. doi: 10.1021/acs.iecr.5b00528.
- Akizuki M, Oshima Y. 2018. Solid acid/base catalysis in sub- and supercritical water. Ind Eng Chem Res. 57:5495–5506. doi: 10.1021/acs.iecr.8b00753.
- Comisar CM, Savage PE. 2004. Kinetics of crossed aldol condensations in high-temperature water. Green Chem. 6:227. doi: 10.1039/b314622g.
- Eom H-J, Kim M-S, Lee D-W, Hong Y-K, Jeong G, Lee K-Y. 2015. Zirconia catalysts (ZrO2 and Na-ZrO2) for the conversion of phenethyl phenyl ether (PPE) in supercritical water. Appl Catal, A. 493:149–157. doi: 10.1016/j.apcata.2014.12.052.
- Iwamura H, Sato T, Okada M, Sue K, Hiaki T. 2014. Organic reactions in sub-and supercritical water in the absence of any added catalyst. J Res Inst Sci Technol. 2014(132):132_1-132_9.
- Ko M-J, Nam H-H, Chung M-S. 2020. Subcritical water extraction of bioactive compounds from Orostachys Japonicus A. Berger. Sci Rep. 10:10890. doi: 10.1038/s41598-020-67508-2.
- Kruse A, Dinjus E. 2007. Hot compressed water as reaction medium and reactant. J Supercrit Fluids. 39:362–380. doi: 10.1016/j.supflu.2006.03.016.
- Kus NS. 2012. Organic reactions in subcritical and supercritical water. Tetrahedron. 68:949–958.
- Li J, Wang S, Li H, Tan Y, Ding Y. 2021. Zn promoted Mg-Al mixed oxides-supported gold nanoclusters for direct oxidative esterification of aldehyde to ester. Int J Mol Sci. 22:8668. doi: 10.3390/ijms22168668.
- Liu S, Huang S, Guan L, Li J, Zhao N, Wei W, Sun Y. 2007. Preparation of a novel mesoporous solid base Na–ZrO2 with ultra high thermal stability. Microporous Mesoporous Mater. 102:304–309. doi: 10.1016/j.micromeso.2006.12.052.
- Liu Z, Ji W, Dong L, Chen Y. 1998. Effect of supported Na+ ions on the texture properties of ZrO2. J Solid State Chem. 138:41–46. doi: 10.1006/jssc.1998.7752.
- Savage PE. 1999. Organic chemical reactions in supercritical water. Chem Rev. 99:603–622. doi: 10.1021/cr9700989.
- Wagner W, Pruß A. 2002. The IAPWS formulation 1995 for the thermodynamic properties of ordinary water substance for general and scientific use. J Phys Chem Ref Data. 31:387–535. doi: 10.1063/1.1461829.
- Watanabe M, Iida T, Aizawa Y, Ura H, Inomata H, Arai K. 2003. Conversions of some small organic compounds with metal oxides in supercritical water at 673 K. Green Chem. 5:539–544. doi: 10.1039/B304686A.