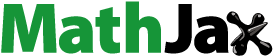
Abstract
A kind of highly dispersed ZrO2 modified Cs/SiO2-based catalyst was prepared via ligand–assisted impregnation strategy. The catalyst was then used to the aldol condensation reaction of methyl acetate (MAc) with formaldehyde (FA) to produce methyl acrylate (MA). The effect of highly dispersed ZrO2 promotion on Cs/SiO2 catalyst selectivity and activity was studied as well. Various techniques such as XRD, FT-IR, TEM, XPS, NH3-TPD, CO2-TPD, N2 physical adsorption, and solid-state MAS NMR were used to characterize the structural features, Cs species states, and acid–base properties of these catalysts. The use of Zr(acac)4 as a zirconium source for modifying Cs/SiO2 could be considered optimal for ligand-assisted impregnation. By modifying the highly dispersed ZrO2, more Cs–O–Si species and a suitable balance of acid–base sites were formed in the Zr–Cs/SiO2 catalyst. As a result, the catalyst showed significant improvements in selectivity and yield of MA under optimized reaction conditions. This study is beneficial for the development and optimization of catalysts for aldol condensation and provides a reference for future industrial applications.
Introduction
Vapor phase aldol condensation of methyl acetate (MAc) with formaldehyde (FA) to prepare methyl acrylate (MA) is regarded as a high-potential MA production process, because it can offer significant economic and environmental benefits compared with other traditional process routes such as the acetone cyanohydrin (ACH) process (Ma et al. Citation2021; Wang et al. Citation2022). The feedstock of this process can be acquired easily through a range of sources such as coal chemicals, petrochemical, biomass and so on, which makes this process generally better insulated from oil-price fluctuations and depletion of oil resources (Bao et al. Citation2018; Zhao et al. Citation2016).
Obviously, the aldol condensation of MAc with FA emerges as a much more thermodynamically favorable reaction with an apparent negative Gibbs free energy change (ΔrGTθ) of the reaction (Guan et al. Citation2020). However, it still needs to be carried out at 350–380 °C with the participation of suitable catalysts because of the more difficult α-deprotonation of MAc (Wang et al. Citation2022). Various attempts have been made to find suitable catalyst systems for this aldol condensation, which could be sorted into V-P-O base catalysts and Cs-base catalysts.
Due to its unique interfacial structure and properties, VPO-based catalysts can activate the C–H bonds of short-chain hydrocarbons and have been found to be one of the most effective catalysts in aldol condensation reactions (Feng et al. Citation2014; Hu et al. Citation2016). The composition of the active phase and the acidity and basicity of the V-P-O have a significant impact on the conversion and selectivity of aldol condensation, which can be controlled by the P:V atomic ratio, calcination temperature and activation atmosphere (Guo et al. Citation2017; Shen et al. Citation2019) Nevertheless, the applicability of the V-P-O base catalysts is unsatisfactory due to the restriction of the catalyst scale-up preparation and rapid deactivation (Wang et al. Citation2022).
Among a series of alkali metal catalysts, Cs-base catalysts possess excellent catalytic performance in the aldol condensation reaction. Due to the limitation of the low specific surface area, Cs species must be loaded on porous catalyst supports for high dispersion and stability (Ma et al. Citation2021). Common porous Cs species supports include SiO2 (Deng et al. Citation2022), Al2O3 (Wang and Cai Citation2020) and zeolite (Yan et al. Citation2015), which could provide the surface –OH to form Si–O–Cs or Al–O–Cs groups with Cs species to activate MAc (Wu et al. Citation2022). With the advancement of research, scientists have conducted a series of studies on acid–base bifunctional Cs base catalysts and found that the acid–base balance of the supported Cs species catalyst could be regulated effectively by adding promoter elements, for example Cs–P/γ–Al2O3 (Wang and Cai Citation2020), Cs–Zr/SiO2 (Li et al. Citation2019), Cs–Si/Al2O3 (Wu et al. Citation2022). The promoter elements not only promote the generation of Cs species on the surface of supports, but also increase the weak/strong acids and bases ratio which are crucial for MA production (Darabi Mahboub et al. Citation2018).
Among them, ZrO2, as an amphoteric metal oxide, is often used for surface modification of catalysts (Dagle et al. Citation2020). The forms and dispersion of ZrO2 will determine the quantity and distribution of surficial active sites on the catalyst, which are influenced by catalyst modification and preparation methods (Salinier et al. Citation2009). Ligand-assisted preparation method is an extremely efficient approach for controlling the size and dispersion of loaded metals or metal oxides (Costa and Rossi Citation2012; Zhang et al. Citation2017). Therefore, organic ligands are employed to hinder the growth process of ZrO2 particles, control the size of ZrO2, and maintain its high dispersibility through the steric hindrance effect (Salinier et al. Citation2009). However, the effect of highly dispersed ZrO2 on aldol condensation over Cs/SiO2 catalysts has rarely been studied.
Herein, we fabricated highly dispersed ZrO2 modified Cs/SiO2 catalysts by ligand–assisted impregnation strategy to enhance the catalytic activity of aldol condensation reaction of MAc with FA. Compared to traditional impregnation process using Zr(NO3)4, in ligand–assisted impregnation strategy, Zr(acac)4 and Zr(O-n-Bu)4 were used as zirconium sources. The physical − chemical features and acid–base properties were comprehensively analyzed through a series of characterization methods. Cs2O and ZrO2 loading and reaction conditions were optimized and the catalyst demonstrates excellent catalytic performance and stability. A comprehensive investigation was conducted to analyze and characterize the performance and properties of the catalyst.
1. Experimental Section
1.1. Materials and Chemicals
The spherical SiO2 support was purchased from Qingdao Hailang Silicone Co. Ltd. The spherical SiO2 was ground into 20–40 meshes and pretreated at 823 K for 6 h in ambient atmosphere before use. All the chemicals used were selected from the Sinopharm Chemical Reagent Company and used without further purification.
1.2. Catalyst Preparation
Normal ZrO2 modified SiO2 catalysts were prepared via incipient wetness impregnation with zirconium nitrate [Zr(NO3)4] in water for 10 h. Two kinds of highly dispersed ZrO2 modified SiO2 catalysts were prepared via ligand–assisted incipient wetness impregnation with solutions of zirconium acetylacetonate [Zr(acac)4] in methanol, zirconium n-butoxide [Zr(O-n-Bu)4] in n-butanol for 10 h, respectively. The obtained samples were treated by drying at 80 °C for 3 h and calcination 500 °C for 4 h in air with a 5 °C/min heating ramp rate.
Cs2O supported Zr/SiO2 catalysts were prepared by wet impregnation with aqueous cesium nitrate (CsNO3) solution for 10 h. Then, the samples after impregnation was dried at 100 °C for 3 h and calcined at 500 °C for 4 h in air with a 5 °C/min heating ramp rate.
The obtained samples were labeled as Zr(A)-xCs/SiO2, Zr(B)-xCs/SiO2, and Zr(N)-xCs/SiO2, where x represents the concentration of Cs2O (wt.%, g/g). A, B and N represents Zr(acac)4, Zr(O-n-Bu)4 and Zr(NO3)4, respectively.
1.3. Materials Characterization
XRD diffractometer (Rigaku Smart Lab) was employed for the crystalline analysis within the scan range of 5°–80°. X-ray photoelectron spectroscopy (XPS) analyses were performed using an ESCALAB 250Xi (Thermo Fisher Scientific) spectrometer with Al Kα radiation at θ = 90°. The binding energy of Zr 3d and Cs 3d spectra were calibrated by the C 1s peak at 284.8 eV. Fourier transform infrared (FTIR) measurements were conducted with a Nicolet-380 spectrometer (Thermo Fisher Scientific) utilizing KBr as a diluting agent. By employing a transmission electron microscope (TEM) (JEOL Ltd., JEM-2010), the microstructural characteristics of the sample could be observed. N2 adsorption–desorption isotherms were measured at 77 K using an ASAP 2020 system. Solid‐state magnetic resonance (NMR) spectra were tracked in an AVANCE III HD 500 MHz (BRUKER BIOSPIN AG, CH) instrument. To evaluate the acid–base sites of the samples, NH3/CO2-temperature-programmed desorption (TPD) were obtained through the utilization of a Micromeritics Auto Chem II 2920 device. During the CO2-TPD experiments, the introduced CO2 will not react with Cs2O to form CsCO3, which affects the experimental results, as demonstrated in Figure S1.
1.4. Catalytic Activity Evaluation
The above-prepared ZrO2 modified Cs/SiO2 catalysts were applied to the aldol condensation of MAc with FA. The stainless-steel micro-fixed bed integral reaction device was used to carry out catalyst evaluation experiments. 6.0 mL catalyst samples (3.0 g) were filled in the reactor with 10 mm inner diameter. Trioxane is used as a source of formaldehyde. A constant-flux pump was used to feed a mixture of MAc, FA, and CH3OH (molar ratio 1:1:2) with the reactor. The reaction was performed at 340–380 °C in the atmosphere, and liquid feed was pumped into the reactor with liquid hourly space velocity (LHSV) of 0.2–2 h−1. After the reaction reached steady-state (4 h), product was condensed and collected in a liquid storage tank. The reaction mixture was quantitatively analyzed using an Agilent 7890GC fitted with DB-WAX capillary column (30 m × 0.25 nm × 0.25 μm).
The conversion of MAc (XMAc), selectivity and yield of MA(SMA and YMA) can be calculated by the following equations:
(1)
(1)
(2)
(2)
(3)
(3)
In the above formulas, is the amount of substance of MAc in feed,
is the amount of substance of leftover MAc in the liquid phase products, and
is the amount of substance of generated MA in the liquid phase products.
2. Results and Discussion
2.1. Catalyst characterization
2.1.1. Structure and morphology
The XRD patterns of three kinds of ZrO2-modified Cs/SiO2 catalysts are shown in . All of the samples presented a broad peak at around the values of 22.0°, which were assigned to the characteristic of amorphous silica (Gao et al. Citation2018). 10Cs/SiO2 demonstrated well distinguished characteristic diffraction peaks at around the values of 19.86°, 28.28°, 34.84°, 40.37°, 45.40° and 50.08°, assigned to the (1 1 1), (1 1 2), (0 0 3), (2 2 2), (3 0 3) and (1 1 4) crystallographic planes of CsNO3 (PDF No. 09-0403), respectively (Ibrahim et al. Citation2019). After introducing a similar content of ZrO2 as shown in Table S1, the characteristic diffraction peaks of CsNO3 disappears in Zr(N)-10Cs/SiO2, Zr(B)-10Cs/SiO2 and Zr(A)-10Cs/SiO2 samples. The above results verified that the modification of ZrO2 tended to promote the decomposition of CsNO3 in the SiO2 during calcination and the homogeneous dispersion of Cs species. There was no new characteristic peak of ZrO2 appeared either, indicating the well dispersed Zr compound over SiO2 (Sohn and Lim Citation2005). By comparison, when the loading of Cs reached 15 wt.%, those CsNO3 peaks appeared again, and Zr(A)-15Cs/SiO2 displayed the weakest intensity of CsNO3 compared to Zr(N)-15Cs/SiO2 and Zr(B)-15Cs/SiO2. This demonstrated that using Zr(acac)4 as zirconium source was more conducive to the process of ZrO2 modification than using Zr(O-n-Bu)4 and Zr(NO3)4, which could promote the CsNO3 decomposition and Cs species formation and dispersion.
Figure 1. XRD patterns of prepared SiO2, 10Cs/SiO2, Zr(A)-xCs/SiO2, Zr(B)-xCs/SiO2, and Zr(N)-xCs/SiO2.

Similar findings can be arrived at by the spectral analysis of FT IR (). The strong absorption peaks of all the samples in 470, 805, 1,105, and 1,231 cm−1 were attributed to the presence of amorphous silica (Yan et al. Citation2015), which implied the main structure of SiO2 remain unchanged after introducing Zr and Cs primarily. The characteristic peak of the Si − OH groups band at 970 cm−1 disappeared after the incorporation of Cs and Zr species, probably corresponding to the consumption of Si − OH to formation of Si − O−Zr and Si − O−Cs species (Gao et al. Citation2018). The N − O bonding antisymmetric stretching vibrations in the CsNO3 could be observed at 1,384 cm−1 in the 10Cs/SiO2 sample, but disappeared in ZrO2 modified 10Cs/SiO2, which suggested the introduction of ZrO2 could promote the decomposition of CsNO3 in the SiO2. As the content of Cs increased to 15 wt.%, this characteristic signal appeared again, and Zr(A)-15Cs/SiO2 displayed the weakest relative intensity. The above results further implied using Zr(acac)4 as zirconium source to modify SiO2 was in favor of the uniformity of Cs species and interaction of Cs and SiO2, consistent with that of XRD analysis.
Figure 2. FT IR spectra of prepared SiO2, 10Cs/SiO2, Zr(A)-xCs/SiO2, Zr(B)-xCs/SiO2, and Zr(N)-xCs/SiO2.

The surface morphological characteristics were observed by TEM to discover the effect of ZrO2 modification on Cs/SiO2 catalysts. revealed that 10Cs/SiO2 did not exhibit crystal characteristics which was consistent with amorphous SiO2 phase. After ZrO2 modification, the crystal particles of ZrO2 can be observed on the surface of SiO2 in . The different organic ligand could influence the morphology of Zr species in the Cs/SiO2 catalyst, and using organic Zr(acac)4 and Zr(O-n-Bu)4 as zirconium source was more propitious to form highly dispersed ZrO2 on the surface of SiO2 compared with using inorganic Zr(NO3)4. Moreover, highly dispersed ZrO2 was instrumental in strengthening interaction between Cs species and SiO2, which has been proved to be contributed to the enhanced catalytic performance by numerous studies (Salinier et al. Citation2009).
2.1.2. Chemical states analysis
The surface chemical states of three kinds of ZrO2 modified Cs/SiO2 catalysts were revealed by XPS spectra. As shown in , the high-resolution Cs 3d spectrum showed two peaks at 738.7 eV and 724.7 eV, which were referred to Cs 3d3/2 and Cs 3d5/2 of Cs+, respectively (Wu et al. Citation2022). For three kinds of ZrO2 modified Cs/SiO2 catalysts, these peaks shifted slightly to the higher binding energy. The shift can be ascribed to the decreased electron density on Cs, which indicated the ZrO2 modification enhanced the interaction between Cs and SiO2. The Zr(A)-10Cs/SiO2 and Zr(B)-10Cs/SiO2 samples displayed a more noticeable binding energy shift of Cs and Zr than Zr(N)-10Cs/SiO2. This phenomenon indicated that when the organic Zr(acac)4 and Zr(On-Bu)4 were used as zirconium sources, the modified ZrO2 will show good dispersion, thus leading to the superior interaction of Cs with ZrO2-modified SiO2 than that with pristine SiO2. In the Zr 3d narrow scan spectrum (), the peaks at 184.9 eV and 182.5 eV can be in accord with to the Zr 3d3/2 and Zr 3d5/2 of Zr4+ (La Parola et al. Citation2022). The ZrO2 content in Cs/SiO2 catalysts modified using different Zr resource was obtained by XRF characterization, as shown in Table S1. The binding energy of Zr 3d in Zr(A)-10Cs/SiO2, Zr(B)-10Cs/SiO2 and Zr(N)-10Cs/SiO2 was quite similar, which can be attributed to these three catalysts having a similar ZrO2 loading.
Figure 4. Cs 3d spectra(a), Zr 3d spectra(b) of prepared 10Cs/SiO2, Zr(A)-10Cs/SiO2, Zr(B)-10Cs/SiO2, and Zr(N)-10Cs/SiO2.

To further investigate the chemical environment of Cs in ZrO2 modified Cs/SiO2 catalysts, solid-state magic angle spinning (MAS) NMR analysis was conducted. shows the chemical shift of 133Cs for 10Cs/SiO2 (−8 ppm), Zr(A)-10Cs/SiO2 (−35 ppm), Zr(B)-10Cs/SiO2 (−32 ppm), and Zr(N)-10Cs/SiO2 (−20 ppm). Generally, a more negative chemical shift of 133Cs indicates stronger chemical combination between Cs and OH of SiO2 (Kim et al. Citation2015). Accordingly, the more negative shift of 133Cs for the Zr(A)-10Cs/SiO2 sample implied that highly dispersed ZrO2 modification was better for the strong combination between Cs species and
OH in SiO2 carriers, which matched with the conclusion of XPS spectra of Cs 3d.
2.1.3. BET and acid–base property analysis
The Brunauer–Emmett–Teller (BET) specific surface area and pore structure information of all catalysts are displayed in . The SiO2 carriers processed a substantial BET specific surface area (269 m2 g−1) and pore sizes (9.2 nm). Due to the introduction of the Cs, the 10Cs/SiO2 showed a relatively smaller BET specific surface area and pore volume, while average pore sizes displayed the opposite tendency. This trend can be explained by the aggregation of excessive Cs species, thereby filling some porous structures. Interestingly, after modification of ZrO2, the impact of Cs on the SiO2 carrier is significantly suppressed. Three kinds of ZrO2 modified Cs/SiO2 catalysts possessed a larger BET specific surface area and pore volume, and smaller average pore sizes, which is more advantageous to the aldol condensation reaction. Due to the similar ZrO2 content, the Zr(A)-10Cs/SiO2, Zr(B)-10Cs/SiO2 and Zr(N)-10Cs/SiO2 show the similar specific surface area and pore structure. The results above implied that modification of ZrO2 was beneficial for inhibiting the aggregation of Cs on the surface of SiO2, and slowing down the destructive effect on SiO2 pore structure. Highly dispersed ZrO2 modification is more conducive to improving pore structure of Cs/SiO2.
Table 1. The BET specific surface areas and pore structure information of prepared SiO2, 10Cs/SiO2, Zr(A)-10Cs/SiO2, Zr(B)-10Cs/SiO2, and Zr(N)-10Cs/SiO2.
It has been validated that the acidity and alkalinity of catalysts play a crucial role in aldol condensation reactions (Guan et al. Citation2020). Herein, NH3-TPD and CO2-TPD tests were performed to characterize the strength of acid and base properties, as shown in . Generally, the strength of acid and base sites of catalyst could be reflected by the temperature range during the desorption process of NH3 and CO2. The acid and base sites in the range of 100–250 °C, 250–400 °C, 400–600 °C are defined as follows weak sites, middle-strong sites and strong sites, respectively (Zheng et al. Citation2022). In , it could be discovered that the base sites signal of 10Cs/SiO2 were in the form of two broad peaks at 120–200 °C and 200–400 °C, indicating that the generation of weak and medium-strong base sites in 10Cs/SiO2 catalyst. With the modification of ZrO2, the total base amount of Zr(A)-10Cs/SiO2, Zr(B)-10Cs/SiO2, and Zr(N)-10Cs/SiO2 catalysts showed an obvious increase, especially weak base sites, which could be assigned to the alkalinity of ZrO2. In , 10Cs/SiO2 catalyst displayed a broad peak indicating the non-uniform of the acid sites. Moreover, medium strong acid sites occupied a big proportion of acid sites, which could speculatively be referred to the Lewis acid of Cs+. After introducing ZrO2 into 10Cs/SiO2, all catalysts displayed an obvious desorption peak in the range of 120 °C to 250 °C, corresponding to the appearance of weak acid sites offered by ZrO2 species. Meanwhile, it was obvious that using Zr(acac)4 as zirconium source to modify SiO2 could provide more acid and base sites than that of using Zr(O-n-Bu)4 and Zr(NO3)4. Therefore, it was deduced that modification of highly dispersed ZrO2 could tune the acid − base property and provide more weak acid and base sites, which is more conducive to the adsorption and activation of reactants during the aldol condensation reaction.
2.2. Aldol condensation evaluation
2.2.1. Effect of ZrO2 modification
The representative ZrO2-modified Cs/SiO2 catalysts with different zirconium source were evaluated for the aldol condensation of MAc and FA. The feed mixture of FA, MAc and CH3OH (molar ratio = 1:1:2) was fed with a LHSV of 0.6 h−1, and the reaction was conducted at 360 °C. CH3OH was used to prevent the hydrolysis of MAc and MA. Except for MA, various byproducts such as acetic acid, acrylic acid and acrolein could also be detected in the liquid-phase products according to the GC analysis results, as shown in Table S2. Very few gas-phase products could be separated out, which were mainly composed of CO, CO2, ethylene and hydrogen.
The activity of the 10Cs/SiO2 and three kinds of ZrO2 modified Cs/SiO2 catalysts is recorded in . ZrO2 modified 10Cs/SiO2 catalysts exhibited higher conversion of MAc and selectivity of MA than 10Cs/SiO2. In particular, Zr(B)-10Cs/SiO2 demonstrated the highest conversion of MAc (34.16%), and Zr(A)-10Cs/SiO2 also had similar performance (32.16%). Zr(A)-10Cs/SiO2 possessed the highest selectivity of MA (88.97%) among all catalysts. The difference in catalytic activities can be attributed to the balance of basic and acid sites in the catalysts. Based on the mechanism of aldol condensation, the deprotonation of α-H of MAc will proceed on the base sites to form the intermediate enol species, while the FA will be activated on the acid sites (Wang et al. Citation2022; Bao et al. Citation2017). The acid and base site densities of all catalysts are summarized in . After the modification with ZrO2, more base and acid sites would appear on the Cs/SiO2 sample. Compared to the 10Cs/SiO2, three types of ZrO2-modified Cs/SiO2 catalysts show a higher density of weak base sites, which is more favourable for the activation and transformation of MAc (Hattori Citation2015). For the Zr(B)-10Cs/SiO2 catalyst with the highest concentration of base sites (0.33 mmol/gcat), the numerous weak base sites would contribute to activation of MAc, resulting in the highest conversion among all the samples (Ma et al. Citation2021). Beyond that, with ZrO2 modification, the total acid sites amount of Zr(A)-10Cs/SiO2 catalyst reached maximum values of 1.03 mmol/gcat and the percentage of weak acid sites reached 62.82%. An appropriate number of weak acid sites in Zr(A)-10Cs/SiO2 catalyst was beneficial for the formation of activated FA, which further converted the α-carbanion into MA, resulting in the higher selectivity among all the samples. A small amount of medium and strong acid sites over the surfaces of ZrO2-modified Cs/SiO2 could suppress accumulation of excess surface activated FA which can be involved in the aggregation to form carbon deposits (Wu et al. Citation2022), "thus resulting in the highest selectivity of MA among all the catalysts. Taking an overview, these results revealed that Zr(A)-10Cs/SiO2 sample displayed the best performance in selectivity and yield of MA, further illustrating using Zr(acac)4 as zirconium source to modify Cs/SiO2 could be considered as optimal for aldol condensation reaction of MAc with FA.
Figure 7. Catalytic activities of prepared 10Cs/SiO2, Zr(A)-10Cs/SiO2, Zr(B)-10Cs/SiO2, and Zr(N)-10Cs/SiO2.

Table 2. Densities of acid and base sites on prepared 10Cs/SiO2, Zr(A)-10Cs/SiO2, Zr(B)-10Cs/SiO2, and Zr(N)-10Cs/SiO2.
2.2.2. Effect of Cs2O and ZrO2 loading
Since the Zr(A)-10Cs/SiO2 sample showed best catalytic activity, this catalyst was chosen for the optimizing experiments of the loading of the ZrO2 and Cs2O, as shown in . With the enhancement of ZrO2 loading, the conversion of MAc increased accordingly from 21.60% to 37.38%, while the selectivity of MA increased from 62.37% to 88.97% and then declined to 78.16%. As shown previously, the modification using ZrO2 could promote the dispersion of Cs species and increase the specific surface area of Cs/SiO2. In addition, the increase of Zr content would favour the formation of active weak acid and base sites, as shown in Figure S2 and Table S3, thereby improving the conversion of MAc and selectivity of MA. Once excessive loading of the ZrO2 in Cs/SiO2 catalyst, the more acid sites will be introduced in catalyst, resulting in the lower selectivity of MA.
Figure 8. Catalytic performance of Zr(A)-Cs/SiO2 catalysts with various ZrO2 (a) and Cs2O (b) loading.

The catalytic performance of ZrO2 modified Cs/SiO2 catalysts with various Cs2O loading is shown in . As the Cs2O loading increases, the selectivity and yield of MA demonstrated the typical “volcanic curve” reaching its peak at 10 wt.% Cs loading, while the conversion of MAc significantly increased from 25.54% to 60.84%. It was noteworthy that a suitable amount of Cs promoted the activation of MAc and the formation of MA, which attributed to the formation of the Si − O−Cs species. On the other hand, when the loading of Cs2O in SiO2 was excessive, the CsNO3 could not be completely transformed to Si − O−Cs species, causing a reduction in the specific surface area and forming the excessive medium and strong base sites, as shown in Figure S2. This would cause undesired hydrolysis of MAc and reduce the selectivity of MA.
All these results revealed that the content of ZrO2 and Cs2O affected the specific surface area and acid–base balance of the ZrO2 modified Cs/SiO2 simultaneously, and determine the catalytic activity for aldol condensation reaction. The optimal ZrO2 modified Cs/SiO2 catalysts can be achieved with 5% ZrO2 loading and 10% Cs2O loading.
2.2.3. Effect of reaction conditions
The aldol condensation catalytic activity of Zr(A)-Cs/SiO2 was tested at different reaction temperatures from 340 °C to 380 °C, as shown in . As the temperature increased, the conversion of MAc gradually elevated from 26.80% to 53.46% and the selectivity of MA first increased from 77.79% to 88.97% and then decreased to 61.38%. Further increasing the temperature, the pyrolyzation and polymerization of reactants turned to be more competitive than the aldol condensation, which resulting in the formation of precursors of coke, gaseous products (COx) and other byproducts, and reducing the selectivity of MA. Thus, 360 °C was regarded as the suitable reaction temperature for Zr(A)-Cs/SiO2.
Figure 9. Effect of reaction temperatures (a) and LHSV (b) on the aldol condensation over a Zr(A)-10Cs/SiO2 catalyst.

The effect of LHSV on the catalytic performance was explored, as shown in . The LHSV was determined by the feed flow rate and catalyst loading volume. As the LHSV was increased from 0.2 h−1 to 2 h−1, the marked decline of the conversion of MAc was detected from 40.15% to 15.21%, while the selectivity of MA rosed at first and kept in a stable higher level gradually later. Essentially the extending of the residence time, is in favor of conversion of MAc and formation of byproducts. On this basis, A maximum yield of MA was explored at an LHSV of 0.6 h−1, which was suitable for aldol condensation on Zr(A)-Cs/SiO2.
2.2.4. Stability and reusability
The long-term stability of prepared Zr(A)-10Cs/SiO2 samples was investigated by the reaction and regeneration cycle experiments of the aldol condensation. After a reaction cycle, the catalyst was regenerated by calcining in the air atmosphere at 400 °C for 10 h. As shown in , the conversion of MAc decreased from 32.18% to 17.61% after 200 h continuous reaction. After 3 regeneration cycles, the Zr(A)-10Cs/SiO2 was successively employed for 600 h, indicating thar prepared Zr(A)-10Cs/SiO2 catalyst displayed good stability and reusability.
Conclusions
Highly dispersed ZrO2 modification with ligand–assisted strategy was applied to improve the catalytic performance of Cs/SiO2-based catalyst for the aldol condensation of MAc with FA. The effect of ZrO2 modification had quite important influence to the existence state of Cs species, specific surface area, and surface acid–base sites, thus further affected the catalytic performance of aldol condensation. Compared to traditional impregnation methods with using inorganic Zr(NO3)4, ligand–assisted impregnation strategy with using organic Zr(acac)4 and Zr(O-n-Bu)4 possessed better performance in the process of ZrO2 modification. On the one hand, the higher dispersion of ZrO2 on the surface catalyst was beneficial for the formation Cs–O–Si species which could promote the formation of MA. On the other hand, ZrO2 modification could improve pore structure and tune acid–base sites balance of Cs/SiO2. The optimal ZrO2 modified Cs/SiO2 catalyst had 5% ZrO2 loading and 10% Cs2O loading, which could achieve the highest selectivity being 88.97% and the yield being 28.63% of MA under optimal reaction conditions. Besides, the optimal catalyst exhibited good reusability in 600 h recycling experiments. Using ligand–assisted strategy during ZrO2 modification is a very effective approach for enhancing catalytic activity of the Cs/SiO2 catalyst for the aldol condensation, which has a good prospect for industrial application.
Supplemental Material
Download MS Word (588.2 KB)Additional information
Funding
References
- Bao Q, Bu T, Yan J, Zhang C, Ning C, Zhang Y, Hao M, Zhang W, Wang Z. 2017. Synthesis of methyl acrylate by aldol condensation of methyl acetate with formaldehyde over Al2O3-supported barium catalyst. Catal Lett. 147:1540–1550. doi: 10.1007/s10562-017-2014-8.
- Bao Q, Qi H, Zhang C, Ning C, Zhang Y, Jiang Y, Wu Y, Gui W, Wang Z. 2018. Highly catalytic activity of Ba/γ-Ti–Al2O3 catalyst for aldol condensation of methyl acetate with formaldehyde. Catal Lett. 148:3402–3412. doi: 10.1007/s10562-018-2535-9.
- Costa NJ, Rossi LM. 2012. Synthesis of supported metal nanoparticle catalysts using ligand assisted methods. Nanoscale. 4:5826–5834. doi: 10.1039/c2nr31165h.
- Dagle VL, Winkelman AD, Jaegers NR, Saavedra-Lopez J, Hu J, Engelhard MH, Habas SE, Akhade SA, Kovarik L, Glezakou V-A, et al. 2020. Single-step conversion of ethanol to n-butene over Ag-ZrO2/SiO2 catalysts. ACS Catal. 10:10602–10613. doi: 10.1021/acscatal.0c02235.
- Darabi Mahboub MJ, Dubois JL, Cavani F, Rostamizadeh M, Patience GS. 2018. Catalysis for the synthesis of methacrylic acid and methyl methacrylate. Chem Soc Rev. 47:7703–7738. doi: 10.1039/c8cs00117k.
- Deng S, Yan T, Ran R, Li J, Zhang G, Li C. 2022. Influence of Al oxides on Cs-SiO2 catalysts for vapor phase aldol condensation of methyl acetate and formaldehyde. Ind Eng Chem Res. 61:5766–5777. doi: 10.1021/acs.iecr.2c00444.
- Feng X, Sun B, Yao Y, Su Q, Ji W, Au C-T. 2014. Renewable production of acrylic acid and its derivative: new insights into the aldol condensation route over the vanadium phosphorus oxides. J Catal. 314:132–141. doi: 10.1016/j.jcat.2014.04.005.
- Gao M, Jiang H, Zhang M. 2018. The influence of calcination temperatures on the acid-based properties and catalytic activity for the 1,3-butadiene synthesis from ethanol/acetaldehyde mixture. Appl Surf Sci. 439:1072–1078. doi: 10.1016/j.apsusc.2018.01.053.
- Guan Y, Ma H, Chen W, Li M, Qian G, Chen D, Zhou X, Duan X. 2020. Methyl methacrylate synthesis: thermodynamic analysis for oxidative esterification of methacrolein and aldol condensation of methyl acetate. Ind Eng Chem Res. 59:17408–17416. doi: 10.1021/acs.iecr.0c02017.
- Guo X, Yang D, Zuo C, Peng Z, Li C, Zhang S. 2017. Catalysts, process optimization, and kinetics for the production of methyl acrylate over vanadium phosphorus oxide catalysts. Ind Eng Chem Res. 56:5860–5871. doi: 10.1021/acs.iecr.7b01212.
- Hattori H. 2015. Solid base catalysts: fundamentals and their applications in organic reactions. Appl Catal A. 504:103–109. doi: 10.1016/j.apcata.2014.10.060.
- Hu J, Lu Z, Yin H, Xue W, Wang A, Shen L, Liu S. 2016. Aldol condensation of acetic acid with formaldehyde to acrylic acid over SiO2-, SBA-15-, and HZSM-5-supported V-P-O catalysts. J Ind Eng Chem 40:145–151. doi: 10.1016/j.jiec.2016.06.018.
- Ibrahim AA, Al-Fatesh AS, Khan WU, Kasim SO, Abasaeed AE, Fakeeha AH. 2019. Kaolin-supported Ni catalysts for dry methane reforming: effect of Cs and mixed K–Na promoters. J Chem Eng Japan. 52:232–238. doi: 10.1252/jcej.18we125.
- Kim TY, Baek J, Song CK, Yun YS, Park DS, Kim W, Han JW, Yi J. 2015. Gas-phase dehydration of vicinal diols to epoxides: dehydrative epoxidation over a Cs/SiO2 catalyst. J Catal. 323:85–99. doi: 10.1016/j.jcat.2014.12.023.
- La Parola V, Liotta LF, Pantaleo G, Testa ML, Venezia AM. 2022. CO2 reforming of CH4 over Ni supported on SiO2 modified by TiO2 and ZrO2: effect of the support synthesis procedure. Appl Catal A. 642:118704. doi: 10.1016/j.apcata.2022.118704.
- Li B, Qi X, Xie M, Wang G, Wang B, Zhang L, Shen L. 2019. Gaseous catalytic condensation reaction of methyl propionate and formaldehyde in a fluidized bed reactor. Can J Chem Eng. 97:1552–1559. doi: 10.1002/cjce.23398.
- Ma H, Guan Y, Chen W, Sui Z, Qian G, Chen D, Zhou X, Duan X. 2021. Support effects of Cs/Al2O3 catalyzed aldol condensation of methyl acetate with formaldehyde. Catal Today. 365:310–317. doi: 10.1016/j.cattod.2020.06.026.
- Salinier V, Corker JM, Lefebvre F, Bayard F, Dufaud V, Basset J-M. 2009. Silica-supported zirconium complexes and their polyoligosilsesquioxane analogues in the transesterification of acrylates: Part 1. synthesis and characterization. Adv Synth Catal. 351:2155–2167. doi: 10.1002/adsc.200900273.
- Salinier V, Niccolai GP, Dufaud V, Basset J-M. 2009. Silica-supported zirconium complexes and their polyoligosilsesquioxane analogues in the transesterification of acrylates: Part 2. Activity, recycling and regeneration. Adv Synth Catal. 351:2168–2177. doi: 10.1002/adsc.200900274.
- Shen L, Yu Z, Wang A, Yin H. 2019. Reaction between methanol and acetic acid catalyzed by SiO2‐supported V‐P‐O catalyst in oxygen atmosphere. Can J Chem Eng. 97:2699–2707. doi: 10.1002/cjce.23571.
- Sohn JR, Lim JS. 2005. The effect of Al2O3 addition on catalytic activities of NiSO4/Al2O3-ZrO2 for acid catalysis. J Chem Eng Japan. 38:791–800. doi: 10.1252/jcej.38.791.
- Wang B, Deng S, Bian Y, Zhang G, Li C. 2022. Aldol condensation of methyl propionate and formaldehyde: thermodynamics, reaction process, and network. Ind Eng Chem Res. 61:15910–15916. doi: 10.1021/acs.iecr.2c02570.
- Wang G, Cai G. 2020. Synergistic effects between acid and base sites and kinetic for synthesis of methylacrolein on the Cs-P/γ-Al2O3 catalyst. Ind Eng Chem Res. 59:17769–17778. doi: 10.1021/acs.iecr.0c03337.
- Wang G, Li Z, Li C. 2022. Recent progress in one-step synthesis of acrylic acid and methyl acrylate via aldol reaction: catalyst, mechanism, kinetics and separation. Chem Eng Sci. 247:117052. doi: 10.1016/j.ces.2021.117052.
- Wu Z, Sun T, Li Z, Li C. 2022. Si-modified Cs/Al2O3 for aldol condensation of methyl acetate with formaldehyde to methyl acrylate by chemical liquid deposition. Ind Eng Chem Res. 61:17467–17478. doi: 10.1021/acs.iecr.2c03415.
- Yan J, Zhang C, Ning C, Tang Y, Zhang Y, Chen L, Gao S, Wang Z, Zhang W. 2015. Vapor phase condensation of methyl acetate with formaldehyde to preparing methyl acrylate over cesium supported SBA-15 catalyst. J Ind Eng Chem. 25:344–351. doi: 10.1016/j.jiec.2014.11.014.
- Zhang Q, Long K, Wang J, Zhang T, Song Z, Lin Q. 2017. A novel promoting effect of chelating ligand on the dispersion of Ni species over Ni/SBA-15 catalyst for dry reforming of methane. Int J Hydrogen Energy. 42:14103–14114. doi: 10.1016/j.ijhydene.2017.04.090.
- Zhao H, Zuo C, Yang D, Li C, Zhang S. 2016. Effects of support for vanadium phosphorus oxide catalysts on vapor-phase aldol condensation of methyl acetate with formaldehyde. Ind Eng Chem Res. 55:12693–12702. doi: 10.1021/acs.iecr.6b03079.
- Zheng K, Gao Q, Li C, Zhang G, Wu Y, Zhang Q, Wang X, Zhang J, Han Y, Tan Y. 2022. A novel and environmentally friendly NASICON-type material: efficient catalyst for condensation of formaldehyde and acetic acid to acrylic acid and methyl acrylate. Chem Eng J. 446:137324. doi: 10.1016/j.cej.2022.137324.