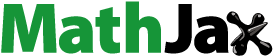
Abstract
TiO2 is known for its strong photocatalytic activity for the degradation of organic pollutants. However, the activity is limited to UV light irradiation. Metal-based nanoparticles have been introduced to enhance the absorbance and activity of TiO2 in the visible light region. The localized surface plasmon resonance (LSPR) effect of the metal improved the degradation efficiency in the visible light region. In this study, Ag was introduced into TiO2 nanoparticulate thin films using a simultaneous one-step plasma-enhanced chemical vapor deposition and physical vapor deposition (PECVD-PVD) method. Metal Ag nanoparticles were prepared using a simple gas-phase PVD method. Adding Ag to TiO2 increases the light absorption for longer wavelengths of light irradiation. The existence of Ag was confirmed by the LSPR absorbance peak originating from the Ag nanoparticles. Further analysis of the Ag nanoparticles was also conducted using X-ray photoelectron spectroscopy, which confirmed the stability of Ag metal upon annealing in N2 atmosphere. The functionality of the nanoparticulate thin films was tested for the degradation of rhodamine 6 G under visible light irradiation, revealing an optimum concentration of 0.24 wt% Ag content exhibited the best activity compared to our fabricated pristine TiO2 nanoparticulate thin film and even commercial TiO2 nanoparticles immobilized on a substrate. This paper presents the potential for producing films composed of nanoparticles through a one-step aerosolized process toward broadening the application scope of photocatalysts in the visible light region.
Introduction
TiO2 is a versatile substance that is widely used in several applications, such as the generation of hydrogen (Gogoi et al. Citation2020), dye-sensitized solar cells, and organic compound decomposition (Santos et al. Citation2015). Its popularity stems from its low cost, nontoxicity, and high chemical, thermal, and photochemical stability (Schneider et al. Citation2014; Falk et al. Citation2018). The pristine crystalline phase of anatase TiO2, with an optical band gap of 3.2 eV, exhibits excellent photodecomposition activity for organic compounds compared to the rutile and brookite phases (Nair et al. Citation2014). However, owing to the wide band gap, the photocatalytic activity of TiO2 is limited to the UV light region (λ < 380 nm), which accounts for only 5% of the solar spectrum (Dong et al. Citation2015; Etacheri et al. Citation2015).
Increasing the light-absorption capability of TiO2 nanoparticles is crucial for their applicability in everyday life. Broadening the light absorption to increase the photocatalytic activity in the visible light region can be achieved through surface modification with the addition of noble metals, such as Ag, Cu, Pt, and Au (Haugen et al. Citation2011; Dong et al. Citation2015). Ag is considered a loaded material owing to its abundance and cost-effectiveness compared with other highly stable noble metals (Elsellami et al. Citation2017). Loaded metals exhibit a unique effect owing to the localized surface plasmon resonance (LSPR). LSPR is described as the oscillation of free electrons in metallic nanoparticles (Gogoi et al. Citation2020). LSPR activates and enhances the visible-light-driven photocatalytic activity (Schneider et al. Citation2014; Fei and Li Citation2015; Méndez-Medrano et al. Citation2016). Moreover, it can increase the light-harvesting capability of TiO2 to longer wavelengths, enhance the scattering of light, and change the electron transfer directly from the metal to the semiconductor, increasing the activity of TiO2 (Etacheri et al. Citation2015).
Seery et al. (Citation2007) described the addition of Ag to TiO2 through Ag-doped TiO2 synthesis using a modified sol–gel process. Furthermore, the photodegradation activity of the nanoparticles was observed under visible light irradiation. The addition of Ag broadened light absorption, enabling higher photodegradation capabilities under visible light irradiation. Zhang et al. (Citation2017a, Citation2017b) confirmed the existence of Ag nanoparticles on the surface of TiO2, which was synthesized through a one-step hydrothermal method. The addition of Ag enhances the visible light absorption. With an appropriate amount of Ag, the photocatalytic activity under visible light irradiation was higher than that of the pristine TiO2 nanoparticles. They further explained that the vibration of Ag electrons makes it possible for them to pass over the Schottky barrier and transfer to TiO2, as shown in . The generated electrons and holes react with oxygen and hydroxyl groups (–OH) or H2O, respectively, to generate hydroxyl radicals (OH•), which are used to degrade organic pollutants. As per previous studies, this mechanism has resulted in the enhanced activity of TiO2 under visible light irradiation with the addition of an appropriate amount of Ag nanoparticles.
Figure 1. TiO2-Ag photocatalytic mechanism for the degradation of organic pollutants under visible light.
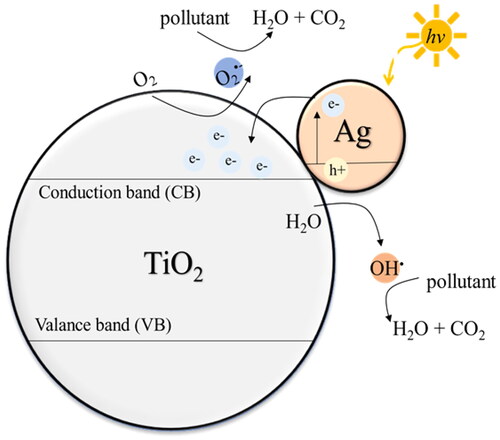
The preparation methods in the aforementioned study are considered as the liquid-phase method, in which Ag nanoparticles are generally prepared by the reduction of Ag ions, involving a series of steps involving purification and drying. The products obtained from these liquid methods often contain Ag+ ions and other intermediates. As reported previously, the LSPR effect of Ag nanoparticles decreases with non-metallic impurities because the LSPR effect is specific to metal nanoparticles (Mogil’naya et al. Citation2021). Therefore, the purity of Ag metal nanoparticles is crucial for LSPR, which subsequently affects their photocatalytic activity.
In our previous study (Jiang et al. Citation2020), a simple one-step gas-phase preparation of nanomaterials by plasma-enhance chemical vapor deposition and physical vapor deposition (PECVD-PVD) was utilized for fabricating photocatalysts and their application under UV light irradiation. In the PVD system, pure Ag metal nanoparticles (less than 10 nm) can be fabricated by the condensation of Ag vapor under a high temperature gradient. Furthermore, PECVD can produce a nanoparticulate thin film of high uniformity and porosity and low agglomeration (Kubo et al. Citation2013). Moreover, the well-dispersed Ag nanoparticles embedded in the porous TiO2 films exhibited an excellent photocatalytic performance under UV light.
To further understand the LSPR effect originating from the pure metal nanoparticles on the photocatalytic activity in the visible region, TiO2-Ag nanoparticulate thin films with different Ag contents were prepared using the PECVD-PVD method. The photodecomposition of liquid organic pollutants induced by TiO2-Ag nanoparticulate thin films under visible light irradiation was investigated.
The characteristics of the nanoparticulate thin film, such as morphology, crystallinity, and light absorbance, were observed using scanning electron microscopy (SEM), X-ray diffraction (XRD), X-ray photoelectron spectroscopy (XPS) and UV–visible diffuse reflectance spectroscopy. The activity of the produced nanoparticulate thin film was studied by measuring the photodecomposition of rhodamine 6 G (R6G) under visible light irradiation. The characteristics and activities of commercially available TiO2 nanoparticles, known as P25, were studied for comparison. This study aims to provide a detailed understanding of the metal–metal oxide characteristics and extend the application of TiO2 to the visible light region induced by the LSPR of Ag metal nanoparticles prepared using a one-step gas-phase method.
1. Experimental Section
1.1 Preparation of Pristine TiO2, TiO2-Ag Nanoparticulate Thin Film, and P25 film
Pristine TiO2 and TiO2-Ag nanoparticulate thin films were produced via one-step PECVD and PECVD-PVD, respectively (). The detailed experimental setup was first proposed in our previous study (Kusdianto et al. Citation2017), and several fabrication parameters were modified in this study. Briefly, for pristine TiO2 nanoparticulate thin film, titanium tetraisopropoxide (TTIP; Kanto Chemical Co., Inc.) was used as the TiO2 precursor. TTIP was placed inside a metal laboratory-made bubbler, maintained at a constant temperature of 40 °C, and Ar gas was flown to vaporize the liquid. Furthermore, the vaporized TTIP was diluted and carried by Ar gas to the radio frequency plasma reactor (100 W; 13.56 MHz; AX-1000IIP, Adtec Plasma Technology Co., Ltd) to be dissociated to form TiO2 nanoparticles. TiO2 nanoparticles were deposited onto a Si substrate at +500 V applied.
Figure 2. Schematic of plasma-enhanced chemical vapor deposition (PECVD) and physical vapor deposition (PVD) system experimental setup.
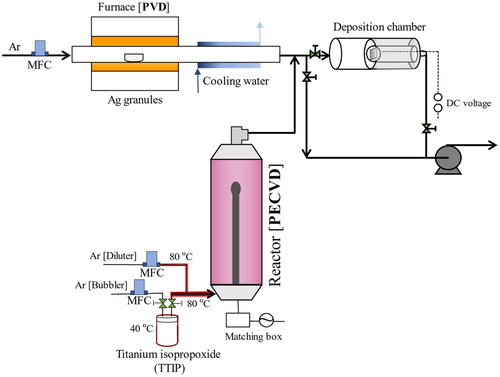
For fabricating TiO2-Ag nanoparticulate thin films, the PECVD and PVD systems were run concurrently. Ag nanoparticles were produced by evaporating Ag granules (Nilaco Corp.) inside a tubular furnace (TMF-300N, AS ONE) in the temperature range of 1020–1220 °C to control the Ag concentration. The generated Ag vapor was rapidly condensed using a cooling water system to form Ag nanoparticles. The Ag and TiO2 nanoparticles from the PVD and PECVD systems were deposited simultaneously onto a voltage applied Si substrate. All pristine TiO2 and TiO2-Ag nanoparticulate thin films prepared in this study were annealed at 500 °C for 3 h under N2 atmosphere.
A commercially available mixture of anatase and rutile TiO2 nanoparticles known as P25 was prepared to form a thin film for comparison purposes. A mixture of P25:HNO3:H2O (2:3:10, weight ratio) was prepared using a mortar and pestle. P25 was added to the mortar, followed by an aqueous solution of HNO3 at a pH of 3 to disperse the P25 nanoparticles. Finally, H2O was added to adjust viscosity. Deposition was achieved by adding the mixture dropwise to a spun Si substrate at a speed of 1500 rpm for 20 s. Subsequently, the film was annealed at 500 °C for 1 h to strengthen the film structure and evaporate the remaining water. To compare their activities, the P25 film was subjected to a photocatalytic test similar to that performed on the prepared pristine TiO2 and TiO2-Ag nanoparticulate thin films.
1.2 Characterization
The morphologies and sizes of the films were observed using SEM (S-5200, Hitachi High Technologies). Detailed observation of the morphology using transmission electron microscopy (TEM, JEOL JEM-2010) was conducted in our previous study (Jiang et al. Citation2020). The crystallinity and crystalline phases of the nanoparticulate thin films were observed using XRD (MiniFlex 600, Rigaku). In addition, XPS (ESCA-3400, Shimadzu) was also performed to understand the chemical state of pristine Ag nanoparticles before and after annealing under N2 atmosphere at 500 °C for 3 h. The light response of the films is an essential factor in photocatalytic activity; this response can be observed from the light-absorption capability of the films, as measured using a UV–Vis spectrophotometer (V-650, Jasco) equipped with an integrated sphere. Furthermore, elemental analysis was performed using inductively coupled plasma optical emission spectroscopy (ICP-OES, SPS 3000; Hitachi High Technologies) to confirm and quantify the amount of Ag in the TiO2-Ag nanoparticulate thin films. The sample preparation for ICP-OES had been previously described (Jiang et al. Citation2020). In brief, samples for ICP were prepared by dissolving the film in HNO3:H2O (1:2 v/v) and subsequently heating the solution. The amount of Ag was measured using ICP-OES, and the weight percentage was calculated from the amount of Ag and the weight of the deposited film.
1.3 Photocatalytic test
The photocatalytic activities of the pristine TiO2, TiO2-Ag nanoparticulate thin films, and the P25 films were evaluated in the liquid phase under visible light irradiation. The photodegradation process was conducted using Rhodamine 6 G (R6G) as a model organic pollutant at a concentration of 5 mg/L. Initially, the sample was left under dark conditions for 30 min to reach adsorption–desorption equilibrium between the dye and the nanoparticles in the film. Subsequently, a photocatalytic test was performed using a solar simulator lamp (HAL-C100, Asahi Spectra) at an intensity of 100 mW/cm2 for 3 h. A UV cut filter was used in the solar simulator to limit light irradiation to visible light region (λ > 380 nm). The photodegradation was monitored between irradiations by measuring the absorption intensity of the dye solution using a UV–Vis spectrophotometer at 30 min intervals. The photocatalytic activity was evaluated by calculating the rate constant (k) from EquationEquation (1)(1)
(1) , where the photodegradation reaction follows a Langmuir–Hinshelwood pseudo-first-order reaction (Kusdianto et al. Citation2018). The rate constant is determined from the slope of the linear curve ln(Ct/C0) vs. (t).
(1)
(1)
where C0 and Ct are the initial and final concentrations of R6G, respectively, and t is the reaction time. The dye concentration was calculated from the absorbance intensity and was proportional to the measured intensity according to Beer–Lambert’s law. In addition, a test was conducted in the dark for 3 h to confirm the dye removal rate by adsorption.
2. Results and Discussion
2.1 Morphological Studies
SEM measurements were conducted to observe the morphology of the produced nanoparticulate films and the P25 film. SEM images of pristine TiO2, TiO2-Ag nanoparticulate thin films, and P25 films are shown in . The film produced by the PECVD-PVD system exhibited a porous structure comprising interconnected spherical nanoparticles. Such a porous morphology is advantageous for film applications because of its higher specific surface area compared to that of denser films, potentially enhancing the photocatalytic activity. The nanoparticles formed in the PECVD reactor formed small, nearly unipolar charged nanoparticles that suppressed the agglomeration process of the nanoparticles, resulting in the formation of these low-agglomeration and porous films (Vollath Citation2008). The non-agglomerated nanoparticles and the effect of the applied bias voltage on the Si substrate can improve the formation of uniform nanoparticulate thin films with good porosity (Kubo et al. Citation2013). The P25 film in appears denser than the films prepared using the PECVD-PVD method.
Figure 3. Scanning electron microscopy (SEM) image of a) pristine TiO2 and TiO2-Ag with b) 0.56 c) 2.97 wt% Ag content prepared by PECVD-PVD method and d) P25 film prepared by spin coating (a–c insets show the size distributions).
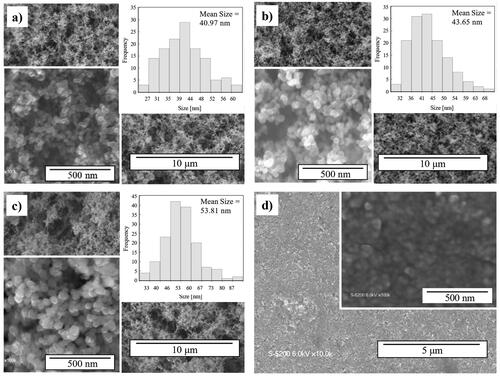
The shape of the TiO2 particle was unaffected by the addition of Ag. The SEM images did not reveal the existence of Ag nanoparticles. However, a slight increase in the size of nanoparticles was observed after adding Ag to TiO2 nanoparticles. On average, the pristine TiO2 film was sized 40.97 nm, whereas the sizes of the TiO2-Ag nanocomposite films with 0.56 and 2.97 wt% Ag contents were 43.65 and 53.81 nm, respectively.
SEM images of the TiO2 and TiO2-Ag nanoparticulate thin films were obtained after annealing. The SEM images suggest that the addition of Ag increases the coalescence of TiO2 nanoparticles during annealing, resulting in larger TiO2 nanoparticles in the TiO2-Ag samples than in the pristine TiO2 nanoparticles. In general, annealing affects the morphology of the film, with factors such as the annealing temperature, time, or even foreign materials influencing the nanoparticle size. In this study, the addition of Ag affected the size of TiO2 nanoparticles.
A more detailed analysis of the size and distribution of Ag nanoparticles by TEM was described in our previous study (Jiang et al. Citation2020). In our previous study, the size of Ag nanoparticles was controlled by differentiating the PVD furnace temperature in the range of 1000–1200 °C. Higher PVD temperatures were proportional to the size of the Ag nanoparticles with sizes ranging from 3 to 10 nm. For Ag nanoparticles in the TiO2-Ag films, round Ag nanoparticles were uniformly dispersed on the surface of the TiO2 nanoparticles. The Ag nanoparticles were clearly visible on the surface of the TiO2 nanoparticles when the Ag content reached 2.2 wt% or greater. Consequently, with an increase in the PVD furnace temperature and Ag content in the film, the size of the TiO2 nanoparticles increased, as observed in this study. Moreover, in this study, the Ag concentration was adjusted by increasing the PVD temperature within the range 1020–1220 °C. An additional observation of the Ag distribution in the TiO2-Ag samples was performed before annealing (data not shown), revealing insignificant differences in the size and distribution of Ag nanoparticles before and after annealing. The size of the Ag nanoparticles was most likely determined during the initial formation in the PVD system. The increase in the PVD furnace temperature was proportional to the amount of Ag vapor, which was the primary factor affecting the size and concentration of Ag nanoparticles formed and deposited in the film.
Controlled growth and size of the nanoparticles are significant for optimum functionality. An increase in the nanoparticle size or agglomeration of nanoparticles is proportional to a decrease in the surface-to-volume ratio. Consequently, the reduction of active surface sites leads to reduced photocatalytic activity. Furthermore, Hanaor & Sorrell Citation2011 discovered that an increased surface-to-volume ratio increases the localized density of states (DOS). The DOS describes the available space for electron occupation. It involves electrons between the conduction and valance bands of semiconductor, representing trapping sites for the excited electrons (Hanaor & Sorrell Citation2011). Conversely, an excess of trapping sites has the disadvantage of increasing the electron–hole recombination. Nam et al. (Citation2018) observed TiO2 nanoparticles and found that larger particles tend to exhibit a lower electron–hole recombination rate; however, the size observed was limited to the range of 1–3 nm. According to these studies, the size of nanoparticles affects not only the active surface area of the photocatalyst and organic compounds, but also their mechanism of electron separation and trapping. Therefore, increasing the size of the nanoparticles can decrease the specific surface area, which is a disadvantage for the photocatalytic activity. However, it can also potentially increase the DOS and lower the electron–hole recombination, which is advantageous for photocatalytic activity. However, this information is based on previous studies, and further research is required.
2.2 Crystallinity and Chemical State of Thin Films
The crystallinity and phase of the nanoparticulate thin films can be understood from the XRD patterns shown in . Annealing is crucial for achieving well-crystallized TiO2 nanoparticles. TiO2 peaks with 2θ = 25.5°, 38.3°, and 48.5°, corresponding to the 101, 004, and 200 planes of crystallized TiO2 in the anatase phase (Kusdianto et al. Citation2017). A peak corresponding to anatase TiO2 was observed in all samples in the XRD pattern. Furthermore, owing to the small size and amount of nanoparticles present in the film, broad peaks were observed in the XRD pattern of the nanoparticulate thin films.
Figure 4. X-ray diffraction (XRD) pattern of nanoparticulate thin films prepared by PECVD-PVD an P25 film prepared by spin coating.
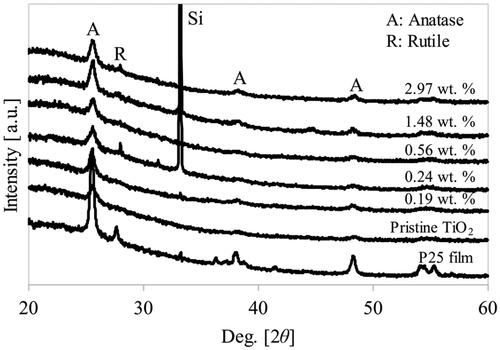
A small diffraction peak at 2θ = 44.5°, corresponding to rutile TiO2, was observed with the addition of Ag (≥ 0.24 wt%). This result is consistent with our previous work that shows TiO2 phase transformation at 500 °C for Ag-loaded TiO2 (Kusdianto et al. Citation2017). The addition of Ag shifts the phase transition from anatase to rutile to a lower annealing temperature. In pristine TiO2, the anatase-to-rutile phase transformation occurred in the range of 400–1200 °C, depending on the fabrication method (Luo et al. Citation2015). Furthermore, it has been reported that factors such as the fabrication method, added materials, initial particle size, and morphology can also influence the transformation temperature of anatase to rutile TiO2 (Etacheri et al. Citation2015). In this study, the TiO2 phase transformation decreased to 500 °C with the one-step PECVD-PVD fabrication method with the addition of Ag metal nanoparticles. The anatase–rutile phase mixture in TiO2 is advantageous for enhancing the photocatalytic activity due to the synergetic effects of the anatase and rutile phases of TiO2, which improves the electron–hole separation process (Haugen et al. Citation2011; Singh & Kumar Citation2021). The anatase–rutile phase mixture was also observed in the P25 film.
The existence of Ag nanoparticles cannot be observed from the peaks corresponding to metal Ag, such as the one at 44.25° on the 200 crystal plane (Ran et al. Citation2018). This may be attributed to the small amount of the well-dispersed Ag nanoparticles within the nanoparticulate films (Okada et al. Citation2001; Santos et al. Citation2015; Gogoi et al. Citation2020). The appearance of the Si peak at 33° was attributed to the Si substrate used for nanoparticle deposition.
Furthermore, XPS measurements were conducted to confirm the stable state or oxidation phase of the pristine Ag nanoparticles before and after annealing under N2 atmosphere at 500 °C for 3 h. The results in show peaks corresponding to 367.2 and 374.2 eV with a spin energy separation of 6.0 eV, confirming that the Ag nanoparticles exist in zero valence (Ag0) or metal Ag (Jiang et al. Citation2012). There is no shift in the XPS peaks after annealing, which confirms that Ag can withstand the oxidation process and is sufficiently stable to maintain its metal form when annealed under N2 atmosphere for 3 h. The metal form of Ag is significant for photocatalytic activity because the LSPR can enhance the activity of TiO2 nanoparticles toward visible light irradiation (Schneider et al. Citation2014).
2.3 UV–Vis Spectrophotometer Absorbance Spectra
The effect of loading different Ag contents on TiO2 and its comparison with pristine TiO2 nanoparticulate thin film and the P25 film were evaluated based on their optical properties and absorbance using a UV–Vis spectrophotometer, as shown in . The characteristics of TiO2 light absorption were evident for wavelengths in the range of 300–400 nm for all samples containing TiO2 nanoparticles. Pristine TiO2 nanoparticles exhibited lower absorbance at longer wavelengths corresponding to visible light, suggesting the low photoactivity of TiO2 at these longer wavelengths. Adding Ag metal nanoparticles increased light absorption at longer wavelengths (λ > 500 nm). Therefore, adding Ag metal nanoparticles to TiO2 nanoparticles increased the light-harvesting ability at longer wavelengths of light. This result was consistent with that of Fei and Li Citation2015 who reported that adding Ag increased visible light absorption of TiO2. A study by Zhang et al. (Citation2017a, Citation2017b) reported a change in absorption for Ag-ZnO nanoparticles, where at lower concentration of Ag the LSPR peak was not visible. However, the effect of Ag metal and its LSPR on the increased visible light absorption in the spectra increased with an increase in Ag concentration, as reflected in our results. Consequently, the LSPR peak of Ag became more prominent.
Figure 6. Absorbance spectra of the PECVD-PVD prepared nanoparticulate film and the P25 film prepared by spin coating.
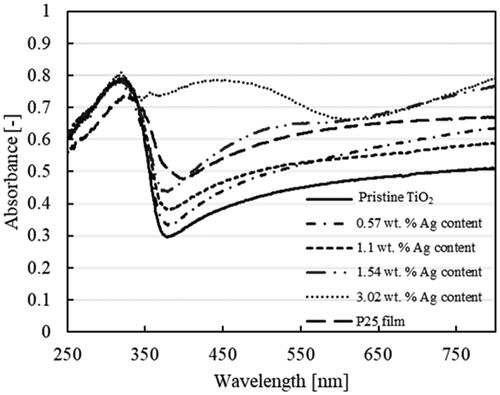
Furthermore, the increase in absorption starting at approximately 450 nm was attributed to the LSPR of loaded Ag metal nanoparticles (Yu et al. Citation2013; Santos et al. Citation2015). The increase in the LSPR peak became noticeable at an Ag content of 3.02 wt%, which can be attributed to the greater amount and size of Ag nanoparticles in our samples. It has been reported that the absorption peak caused by the LSPR intensifies with the increase in the diameter of nanoparticles (Subramanian et al. Citation2004).
2.4 Photocatalytic Degradation of R6G under Visible Light
The photocatalytic activity was evaluated for the degradation of R6G under visible light irradiation. The weight of the fabricated film used for the photocatalytic evaluation was 0.06 mg with a substrate size of 1 × 1 cm2. The experiment was conducted in several scenarios: photolysis of R6G, photocatalytic activity in the presence of pristine TiO2 and TiO2-Ag nanoparticulate films with various Ag contents, and the P25 film. In addition, an adsorption test of the aliquot dye and film was performed in the dark, in the absence of light irradiation.
The degradation process was deduced from the change in the color of R6G, which was quantified by the decrease in absorbance (). The values of the measured absorbances were then used to compare the degradation processes. Based on the results shown in , the adsorption test showed less than 1% dye removal. Furthermore, the presence of a photocatalyst can increase the reaction rate constant of R6G degradation compared to photolysis and adsorption tests. The photocatalytic activity of P25 film was higher than that of the pristine TiO2 nanoparticulate thin film. Upon examination of the SEM image, P25 was observed to have exhibited a denser morphology than the fabricated film, potentially resulting in a reduced surface-to-volume ratio and consequently impacting the photocatalytic activity. However, P25, a commercially available material that contains a mixture of anatase and rutile TiO2 and enhances the photocatalytic activity efficiency. Charge carriers can move from the rutile to the anatase phase, which can further decrease the electron–hole recombination rate of TiO2 (Scanlon et al. Citation2013). In contrast, in our pristine TiO2 nanoparticulate film, only the anatase phase of TiO2 is present, making electron–hole recombination more likely and decreasing the photocatalytic activity (Etacheri et al. Citation2015). Therefore, further investigation of the P25-Ag photocatalytic test was not conducted based on differences in the structure and crystal phase. Nevertheless, the incorporation of Ag is anticipated to enhance the photocatalytic activity of P25, such as listed in potentially increasing the reaction rate of 0.0002 min−1 (0.012 h−1) for P25 to 0.0008 min−1 (0.048 h−1) for P25-Ag.
Figure 7. Absorbance versus wavelength as a function of time corresponding to the photocatalytic degradation of R6G by a) TiO2 and b) TiO2-Ag 0.24 wt%; comparison of c) percent degradation and d) reaction rate constant of the degradation processes.
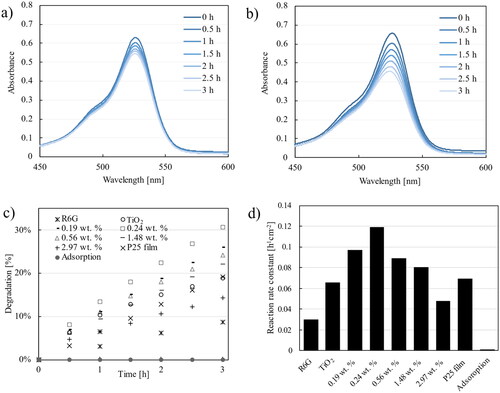
Table 1. Comparison of the photocatalytic activities reported in the present study and the literature.
Loading Ag onto the produced TiO2 increased the photocatalytic activity under visible light irradiation. The lower photocatalytic activity of the P25 film than that of our nanocomposite thin film could be attributed to the general characteristics of P25, including its low surface area, nonporous nature, and low activity in the visible light region (Han et al. Citation2014).
Preliminary tests were conducted to assess the photocatalytic activity of pristine TiO2 annealed in air atmosphere, on samples weighing 0.065 mg (± 20%) to determine the reaction rate constant (data not shown). From these experiments, the coefficient of variation (CV), which indicates the dispersion of values relative to the mean, was calculated. The resulting CV of 0.22 indicates low variability, implying a high likelihood of reproducibility for the fabricated film, which includes the photocatalytic test results in this study.
From this study, we deduced that the addition of Ag to TiO2 can increase its photocatalytic activity under visible light. Furthermore, we investigated the effect of Ag concentration on the photocatalytic activity. The optimum Ag content was observed at 0.24 wt% with a rate constant of 0.119 h−1 cm−2 (). An increase in the Ag content above 0.24 wt% resulted in a decrease in photocatalytic activity. With 2.97 wt% Ag, the photocatalytic activity was lower than that of pristine TiO2.
The photocatalytic activity of TiO2 under visible light is known to be less effective than that under UV light because of the wider band gap (Han et al. Citation2014). With the addition of Ag, the activity initially increased, but then decreased after reaching an optimum Ag content. Several factors affect photocatalytic activity. From our tested products, it is evident that adding Ag increases the size of the nanoparticles and broadens their absorption ability in the visible light region. A possible reason for the decrease in photocatalytic activity is the influence of the increasing size of TiO2 nanoparticles with an increase in Ag content, leading to a decrease in the active surface area and photocatalytic performance. In addition, several studies have suggested that an increase in Ag content above the optimum amount is likely to enhance the electron–hole recombination process in Ag itself (Bumajdad & Madkour Citation2014; Sun et al. Citation2020).
lists the photocatalytic activities of the film fabricated in this study and those reported in the literature. However, the experimental conditions under which the photocatalytic activities were evaluated are different for each study; hence, factors such as the form of the photocatalyst (powder or film), light intensity, and type and concentration of the dye used should be considered. The photocatalytic activity of the TiO2-Ag film prepared herein increased twice compared to that of pristine TiO2 nanoparticulate thin films. Compared to those of other films, the photocatalytic activity of our film demonstrated reasonable results.
The degradation process was deduced from the change in the color of R6G, which was quantified by the decrease in absorbance (). Vinu et al. (Citation2010) reported that the discoloration of the dye is proportional to the mineralization process and the decrease in the total organic compound. In addition, the decomposition pathway for identifying intermediate products using mass spectroscopy has been reported in several previous studies (Vinu et al. Citation2010; Rasheed et al. Citation2017; Vanlalhmingmawia et al. Citation2022). In these studies, the initial R6G exhibited a base peak at m/z = 443. They revealed a possible reaction pathway for the mineralization of R6G. After exposure of the dye and catalyst to light, intermediate peaks can be observed, such as those of N-de-ethylated compounds, before the complete mineralization of the dye, forming CO2 and H2O.
3. Conclusions
TiO2-Ag nanoparticulate thin films were successfully fabricated using a one-step PECVD-PVD method. This one-step process has shown great potential in the formation of films consisting of nanoparticles with low agglomeration. The PVD system enabled the deposition of the Ag metal nanoparticles. The addition of Ag did not affect the spherical linking structure of TiO2. However, the size of the nanoparticles increased slightly. Ag nanoparticles were found to be in a stable metal condition even after annealing under N2 atmosphere. Furthermore, the addition and increase in Ag content broadened the light absorbance capability of TiO2 in the visible light region, which expand its application scope, aside from the usual activity in the UV light region. The photocatalytic test with R6G was conducted under visible light irradiation with an optimum degradation rate of 0.1191 h−1 cm−2 at 0.24 wt% of Ag content. This result demonstrates the effectiveness of loading Ag metal nanoparticles and their LSPR to enhance the photocatalytic activity of TiO2.
Disclosure statement
No potential conflict of interest was reported by the author(s).
Additional information
Funding
Literature Cited
- Bumajdad A, Madkour M. 2014. Understanding the superior photocatalytic activity of noble metals modified titania under UV and visible light irradiation. Phys Chem Chem Phys. 16:7146–7158. doi: 10.1039/c3cp54411g.
- Dermenci KB, Genc B, Ebin B, Olmez-Hanci T, Gürmen S. 2014. Photocatalytic studies of Ag/ZnO nanocomposite particles produced via ultrasonic spray pyrolysis method. J. Alloys Compd. 586:267–273. doi: 10.1016/j.jallcom.2013.10.004.
- Dong H, Zeng G, Tang L, Fan C, Zhang C, He X, He Y. 2015. An overview on limitations of TiO2-based particles for photocatalytic degradation of organic pollutants and the corresponding countermeasures. Water Res. 79:128–146. doi: 10.1016/j.watres.2015.04.038.
- Elsellami L, Dappozze F, Houas A, Guillard C. 2017. Effect of Ag + reduction on the photocatalytic activity of Ag-doped TiO2. Superlattices Microstruct. 109:511–518. doi: 10.1016/j.spmi.2017.05.043.
- Etacheri V, Di Valentin C, Schneider J, Bahnemann D, Pillai SC. 2015. Visible-light activation of TiO2 photocatalysts: Advances in theory and experiments. J. Photochem. Photobiol. C Photochem. Rev. 25:1–29. doi: 10.1016/j.jphotochemrev.2015.08.003.
- Falk GS, Borlaf M, López-Muñoz MJ, Fariñas JC, Rodrigues Neto JB, Moreno R. 2018. Microwave-assisted synthesis of TiO2 nanoparticles: photocatalytic activity of powders and thin films. J. Nanoparticle Res. 20:1–10.
- Fei J, Li J. 2015. Controlled preparation of porous TiO2-Ag nanostructures through Supramolecular assembly for plasmon-enhanced photocatalysis. Adv Mater. 27:314–319. doi: 10.1002/adma.201404007.
- Gogoi D, Namdeo A, Golder AK, Peela NR. 2020. Ag-doped TiO2 photocatalysts with effective charge transfer for highly efficient hydrogen production through water splitting. Int. J. Hydrogen Energy. 45:2729–2744. doi: 10.1016/j.ijhydene.2019.11.127.
- Han C, Likodimos V, Khan JA, Nadagouda MN, Andersen J, Falaras P, Rosales-Lombardi P, Dionysiou DD. 2014. UV–visible light-activated Ag-decorated, monodisperse TiO2 aggregates for treatment of the pharmaceutical oxytetracycline. Environ Sci Pollut Res Int. 21:11781–11793. doi: 10.1007/s11356-013-2233-5.
- Hanaor DAH, Sorrell CC. 2011. Review of the anatase to rutile phase transformation. J Mater Sci. 46:855–874. doi: 10.1007/s10853-010-5113-0.
- Haugen AB, Kumakiri I, Simon C, Einarsrud MA. 2011. TiO2, TiO2/Ag and TiO2/Au photocatalysts prepared by spray pyrolysis. J. Eur. Ceram. Soc. 31:291–298. doi: 10.1016/j.jeurceramsoc.2010.10.006.
- Jiang D, Kusdianto K, Kubo M, Shimada M. 2020. Effect of Ag loading content on morphology and photocatalytic activity of Ag-TiO2 nanoparticulate films prepared via simultaneous plasma-enhanced chemical and physical vapor deposition. Mater Res Express. 7:116406. doi: 10.1088/2053-1591/abc720.
- Jiang L, Zhou G, Mi J, Wu Z. 2012. Fabrication of visible-light-driven one-dimensional anatase TiO2/Ag heterojunction plasmonic photocatalyst. Catal. Commun. 24:48–51. doi: 10.1016/j.catcom.2012.03.017.
- Karimi-Maleh H, Kumar BG, Rajendran S, Qin J, Vadivel S, Durgalakshmi D, Gracia F, Soto-Moscoso M, Orooji Y, Karimi F. 2020. Tuning of metal oxides photocatalytic performance using Ag nanoparticles integration. J. Mol. Liq. 314:113588. doi: 10.1016/j.molliq.2020.113588.
- Kubo M, Ishihara Y, Mantani Y, Shimada M. 2013. Evaluation of the factors that influence the fabrication of porous thin films by deposition of aerosol nanoparticles. Chem. Eng. J. 232:221–227. doi: 10.1016/j.cej.2013.07.097.
- Kusdianto K, Jiang D, Kubo M, Shimada M. 2017. Fabrication of TiO2–Ag nanocomposite thin films via one-step gas-phase deposition. Ceram. Int. 43:5351–5355. doi: 10.1016/j.ceramint.2017.01.009.
- Kusdianto K, Jiang D, Kubo M, Shimada M. 2018. Effect of annealing temperature on the photocatalytic activity of Ag–TiO2 nanocomposite films by one-step gas-phase deposition. Mater. Res. Bull. 97:497–505. doi: 10.1016/j.materresbull.2017.08.062.
- Luo Z, Poyraz AS, Kuo CH, Miao R, Meng Y, Chen SY, Jiang T, Wenos C, Suib SL. 2015. Crystalline mixed phase (anatase/rutile) mesoporous titanium dioxides for visible light photocatalytic activity. Chem Mater. 27:6–17. doi: 10.1021/cm5035112.
- Méndez-Medrano MG, Kowalska E, Lehoux A, Herissan A, Ohtani B, Bahena D, Briois V, Colbeau-Justin C, Rodríguez-López JL, Remita H. 2016. Surface modification of TiO2 with Ag nanoparticles and CuO nanoclusters for application in photocatalysis. J Phys Chem C. 120:5143–5154. doi: 10.1021/acs.jpcc.5b10703.
- Mogil’naya TY, Krit BL, Morozova NV, Kuvshinov VV, Sleptsov VV, Fedotikova MV, Pagava LL, Gorozheev MY. 2021. Evaluation the influence of impurities on the occurrence of a local surface plasmon resonance effect. Surf Engin ApplElectrochem. 57:567–571. doi: 10.3103/S1068375521050094.
- Nair PB, Justinvictor VB, Daniel GP, Joy K, James Raju KC, Devraj Kumar D, Thomas PV. 2014. Optical parameters induced by phase transformation in RF magnetron sputtered TiO2 nanostructured thin films. Prog. Nat. Sci. Mater. Int. 24:218–225. doi: 10.1016/j.pnsc.2014.05.010.
- Nam Y, Li L, Lee JY, Prezhdo OV. 2018. Size and shape effects on charge recombination dynamics of TiO2 nanoclusters. J Phys Chem C. 122:5201–5208. doi: 10.1021/acs.jpcc.8b00691.
- Ng CM, Chen PC, Manickam S. 2012. Hydrothermal crystallization of titania on silver nucleation sites for the synthesis of visible light nano-photocatalysts-enhanced photoactivity using Rhodamine 6G. Appl. Catal. A Gen. 433-434:75–80. doi: 10.1016/j.apcata.2012.05.004.
- Okada K, Yamamoto N, Kameshima Y, Yasumori A, MacKenzie KJD. 2001. Effect of silica additive on the anatase-to-rutile phase transition. J. Am. Ceram. Soc. 84:1591–1596. doi: 10.1111/j.1151-2916.2001.tb00882.x.
- Ran H, Fan J, Zhang X, Mao J, Shao G. 2018. Enhanced performances of dye-sensitized solar cells based on Au-TiO2 and Ag-TiO2 plasmonic hybrid nanocomposites. Appl. Surf. Sci. 430:415–423. doi: 10.1016/j.apsusc.2017.07.107.
- Rasheed T, Bilal M, Iqbal HMN, Hu H, Zhang X. 2017. Reaction mechanism and degradation pathway of rhodamine 6G by photocatalytic treatment. Water. Air. Soil Pollut. 228:291. doi: 10.1007/s11270-017-3458-6.
- Santos LM, Machado WA, França MD, Borges KA, Paniago RM, Patrocinio AOT, Machado AEH. 2015. Structural characterization of Ag-doped TiO2 with enhanced photocatalytic activity. RSC Adv. 5:103752–103759. doi: 10.1039/C5RA22647C.
- Saravanan R, Manoj D, Qin J, Naushad M, Gracia F, Lee AF, Khan MM, Gracia-Pinilla MA. 2018. Mechanothermal synthesis of Ag/TiO2 for photocatalytic methyl orange degradation and hydrogen production. Process Saf. Environ. Prot. 120:339–347. doi: 10.1016/j.psep.2018.09.015.
- Scanlon DO, Dunnill CW, Buckeridge J, Shevlin SA, Logsdail AJ, Woodley SM, Catlow CRA, Powell MJ, Palgrave RG, Parkin IP, et al. 2013. Band alignment of rutile and anatase TiO2. Nat Mater. 12:798–801. doi: 10.1038/nmat3697.
- Schneider J, Matsuoka M, Takeuchi M, Zhang J, Horiuchi Y, Anpo M, Bahnemann DW. 2014. Understanding TiO2 photocatalysis: mechanisms and materials. Chem Rev. 114:9919–9986. doi: 10.1021/cr5001892.
- Seery MK, George R, Floris P, Pillai SC. 2007. Silver doped titanium dioxide nanomaterials for enhanced visible light photocatalysis. J. Photochem. Photobiol. A Chem. 189:258–263. doi: 10.1016/j.jphotochem.2007.02.010.
- Singh A, Kumar S. 2021. Effect of Ag doping on phase-change and photocatalytic performance of rutile–anatase mixed-phase titanium dioxide (TiO2) nanoparticles. Appl Phys A. 127:1–14. doi: 10.1007/s00339-021-04993-w.
- Subramanian V, Wolf EE, Kamat PV. 2004. Catalysis with TiO2/Gold Nanocomposites. Effect of metal particle size on the fermi level equilibration. J Am Chem Soc. 126:4943–4950. doi: 10.1021/ja0315199.
- Sun Y, Gao Y, Zeng J, Guo J, Wang H. 2020. Enhancing visible-light photocatalytic activity of Ag-TiO2 nanowire composites by one-step hydrothermal process. Mater. Lett. 279:128506. doi: 10.1016/j.matlet.2020.128506.
- Vanlalhmingmawia C, Sreenivasa S, Tiwari D, Lee SM. 2022. Novel nanocomposite thin films for efficient degradation of Rhodamine B and Rhodamine 6G under visible light irradiation: reaction mechanism and pathway studies. Environ. Eng. Res. 28:220430–220430. doi: 10.4491/eer.2022.430.
- Vinu R, Akki SU, Madras G. 2010. Investigation of dye functional group on the photocatalytic degradation of dyes by nano-TiO2. J Hazard Mater. 176:765–773. doi: 10.1016/j.jhazmat.2009.11.101.
- Vollath D. 2008. Plasma synthesis of nanopowders. J Nanopart Res. 10:39–57. doi: 10.1007/s11051-008-9427-7.
- Wu MC, Lin TH, Hsu KH, Hsu JF. 2019. Photo-induced disinfection property and photocatalytic activity based on the synergistic catalytic technique of Ag doped TiO2 nanofibers. Appl. Surf. Sci. 484:326–334. doi: 10.1016/j.apsusc.2019.04.028.
- Yu C, Wei L, Li X, Chen J, Fan Q, Yu JC. 2013. Synthesis and characterization of Ag/TiO2-B nanosquares with high photocatalytic activity under visible light irradiation. Mater. Sci. Eng. B Solid-State Mater. Adv. Technol. 178:344–348. doi: 10.1016/j.mseb.2013.01.015.
- Zhang X, Wang Y, Hou F, Li H, Yang Y, Zhang X, Yang Y, Wang Y. 2017a. Effects of Ag loading on structural and photocatalytic properties of flower-like ZnO microspheres. Appl. Surf. Sci. 391:476–483. doi: 10.1016/j.apsusc.2016.06.109.
- Zhang Y, Wang T, Zhou M, Wang Y, Zhang Z. 2017b. Hydrothermal preparation of Ag-TiO2 nanostructures with exposed {001}/{101} facets for enhancing visible light photocatalytic activity. Ceram. Int. 43:3118–3126. doi: 10.1016/j.ceramint.2016.11.127.