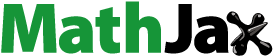
Abstract
Soapstock, mainly composed of organic acid salts, is generated in large quantities during the vegetable oil refining process. However, there are serious environmental and economic problems with conventional treatment, and a new approach for effective utilization is required. Our research explored the application of Kolbe electrolysis, a process typically reserved for water-soluble organic acids with low carbon number, to water-insoluble organic acids. We discovered that the reaction can occur effectively with the water-insoluble organic acids when they are in salt form, producing dimerized hydrocarbons and sodium hydroxide. These produced hydrocarbons can be used as alternative biofuels, such as combustion aids for power generation or as boiler fuel. Simultaneously, the caustic soda can be upcycled. We also examined various operating factors and clarified that Kolbe electrolysis proceeded efficiently at high temperatures, low voltages, and high concentrations. Interestingly, the heating values of the dimerized product increased with the length of the carbon chain of the organic acid, although the product yield in Kolbe electrolysis decreased slightly. Therefore, the Kolbe electrolysis treatment of organic acid salt is an upcycling system that produces more energy than it consumes, thereby achieving a positive energy balance.
1. Introduction
Vegetable oils are an essential part of human life and are used as raw materials for foods and chemical products (Biermann et al. Citation2011). Crude oil obtained directly from oil crops contains components that are undesirable for food and processing applications. Accordingly, refining processes involving several steps are employed to remove these undesirable components (Barbusiński et al. Citation2021). As a result, by-products are generated that account for 10–30% of total vegetable oil production. Among these by-products, soapstock, which is mainly generated during deacidification step, is the most abundant and is generated more than 8.4 million tons annually, which is 6% of vegetable oil production, around the world (Haas Citation2005). It is rich in organic acid salts produced by neutralization of free fatty acids in crude oil with sodium hydroxide, and there is currently no effective way to utilize this product. A similar waste consisting of organic acid salts is generated in the paper industry called black liquor (Spínola et al. Citation2021). Material recycling of alkali from black liquor is already implemented in the paper industry, which uses a large amount of alkali and has a low product price. However, this process is not applicable to the vegetable oil industry, which does not require as much heat as the paper industry.
To date, various studies have been conducted on the treatment of organic acid salt wastes. The simplest method is incineration in a boiler for the purpose of heat recovery. However, there are no actual applications due to boiler damage from alkali metals such as Na and K and clogging of the boiler by non-combustible salts (Sako and Okajima Citation2008). Currently, there is no practical solution for the treatment of organic acid salt wastes, and wastewater treatments, such as the activated sludge method, have to be used. However, because its waste contents 5–50 wt% of organic matter, it requires dilution with a large amount of water and a long processing time to treat it as wastewater. In addition, since a considerable amount of excess sludge is generated, it requires a large amount of energy to reprocess the sludge (Barbusiński et al. Citation2021). Although organic acid salts are generated in large amounts by various industries, an effective treatment technology has not yet been developed, which is a serious problem requiring an immediate solution in the industry (Qiu et al. Citation2021; Yu et al. Citation2022). The difficulty lies in the contradictory properties of organic and inorganic matters, that is, organic acid salt waste has a high organic matter content and contains alkali metals.
This study focused on the Kolbe electrolysis reaction as a new treatment method for organic acid salt waste, with the aim of constructing a technology that can effectively utilize organic and inorganic matters without requiring high temperature. By applying a voltage to an aqueous solution in which an organic acid is dissolved, dimerization of the alkyl group (R-) of the organic acid (R-COOH) proceeds as follows (Meng et al. Citation2022):
(1)
(1)
Previous reports have been limited to water-soluble organic acids, mainly molecules with ≤6 carbon atoms (Zhang et al. Citation2022). Therefore, it was impossible to obtain products worthy of synthesizing electric energy (such as the conversion of acetic acid to ethane and butyric acid to butane), and industrial significance was not found. Recently, this electrolysis has attracted attention as an upgrading technology for biogenic acids, but the reactants are still limited to only water-soluble carboxylic acids (Creusen et al. Citation2018; Qiu et al. Citation2022; Zhang et al. Citation2022). However, the carbon numbers of the organic acid salts that comprise the actual wastes range from 8–20, and their composition varies depending on the derived vegetable oil. For example, rapeseed or soybean-derived wastes mainly contain salts of oleic acid and linoleic acid, while waste derived from coconut and palm kernel contain high amounts of salts of octanoic acid, capric acid, and lauric acid.
Employing organic acids with a higher carbon number results in products with longer carbon chain lengths, thereby enhancing their added value. However, the desired electrolysis reaction will not proceed because the organic acid, which is a reactant, becomes insoluble in water. To resolve this problem, we hypothesized that even a water-insoluble organic acid with higher carbon numbers can become reactants for Kolbe electrolysis if they are in the form of salts, as this would allow them to dissolve in water. Moreover, when an organic acid salt is used, the reaction in EquationEq.(2)(2)
(2)
(2)
(2)
will proceed. It is expected to not only result in the production of longer-chain hydrocarbons with a higher calorific value, but also in the simultaneous regeneration of alkali. In other words, there is a high possibility that organic acid salt waste, which is generated in large quantities in existing industries and is difficult to dispose of, will be recycled into hydrocarbons for biofuel and alkali for neutralization.
In this study, first, an electrolysis reaction experiment on Na salts of water-insoluble carboxylic acids was performed, and whether the desired Kolbe reaction would proceed was investigated. Next, the effects of reaction temperature, voltage, and raw material concentration were investigated to clarify the mechanism of this electrolysis reaction and to identify the conditions for the desired Kolbe reaction to proceed efficiently. Finally, since the carbon number of fatty acid salts generated in the vegetable oil industry is over 8, electrolysis experiments were conducted with raw materials with large carbon numbers to examine the applicability of this method.
2. Experiment
Sodium caprylate (99.0%; Tokyo Chemical Industry CO., Ltd., Tokyo, Japan), which is a fatty acid salt with a carbon chain length of 8, was used as a model raw material. For comparison, caprylic acid (special grade; Fujifilm Wako Pure Chemical Industries, Ltd., Osaka, Japan), which is a fatty acid, was also used. The fatty acid salt sodium decanoate (99.0%; Tokyo Chemical Industry Co., Ltd.) with a carbon chain length of 10 and the fatty acid salt sodium laurate (97.0%; Tokyo Chemical Industry Co., Ltd.) with a carbon chain length of 12 were also used. Ultrapure water was used as a solvent and adjusted to a predetermined fatty acid salt concentration.
shows the experimental setup. A platinum mesh electrode (35 × 25 mm, 80 mesh; BAS CO., Ltd., Tokyo, Japan) commonly used for electrolysis was used for both electrodes. Electrodes were placed in the electrolytic cell with an inter-electrode distance of 2 cm, and 100 cm3 of the reaction solution was added. Thereafter, it was placed in a constant temperature water bath at a predetermined temperature T, and while being sufficiently stirred, an electrolysis experiment was performed by applying a predetermined voltage E with a DC stabilized power supply (AD-8722D, A&D CO., Ltd., Tokyo, Japan). As operating factors, T, E, and C were varied from 10–50 °C, 5–20 V, and 0.06–0.24 mol/dm3, respectively. In the electrolysis experiment, the reaction progressed according to the amount of electricity generated in the system, therefore, the amount of electricity Q that has flowed was an index of the reaction time. Therefore, Q defined by EquationEq.(3)(3)
(3) ,
(3)
(3)
was calculated from the output current, I, of the power supply. It was kept at 1000 C and the operating factors were varied.
In analysis, the pH of the solution was measured with a pH meter (F-51, Horiba Ltd., Kyoto, Japan). In addition, to analyze the reactants and products, the solution after reaction was adjusted to pH 1 using sulfuric acid (95.0%; Fujifilm Wako Pure Chemical Industries, Ltd.) to protonate fatty acid ions. Next, fat-soluble components in the reaction solution were extracted with hexane (special grade; Fujifilm Wako Pure Chemical Industries, Ltd.), and hexanol (special grade; Fujifilm Wako Pure Chemical Industries, Ltd.) was added as an internal standard reagent for sample preparation. Then, the concentration of each component was measured by a gas chromatograph (GC) equipped with a flame ion detector. InertCap WAX-HT (GL-Science, Inc., Tokyo, Japan, film thickness 0.25 µm, i.d. 0.25 mm, length 30 m, polyethyleneglycol) was used as the analytical column. Helium (99.995%; NIPPON HELIUM Inc., Kanagawa, Japan) was used as the carrier gas. The split ratio was 30:1 and the injection volume were 1.0 µL. The injection and detection temperatures were 300 °C, the temperature of the column was initially held at 60 °C for 5 min, then increased to 270 °C at a rate of 10 °C/min, and held for 4 min.
3. Results and Discussion
3.1. Confirmation of progress of Kolbe electrolysis reaction
shows the GC analysis results of the product recovered by hexane extraction after an electrolysis experiment using an aqueous sodium fatty acid solution with a carbon chain length of 8 at an electric charge Q = 1000 C. Peaks for alcohols with 7 carbon atoms (heptanol, R-OH) and aldehydes (heptanal, R'-COH) were observed in addition to the dimerized hydrocarbon (tetradecane, R-R) of the reactant. shows the reaction mechanism of Kolbe electrolysis reported in the previous research (Zhang et al. Citation2022). A carboxylic acid dissolved in water becomes an alkyl radical (R) via decarboxylation by an electrode reaction. Dimerization of these alkyl radicals in the bulk liquid phase produces hydrocarbon. Simultaneously, alkyl radicals become carbocations (R+) through electrode reactions, and alcohols are produced through electrode reactions with hydroxide ions (OH-) in water. Furthermore, the electrode reaction and the reaction with hydroxide ions produce aldehydes. Therefore, dimerized hydrocarbons, 1-alcohols, and 1-aldehydes were observed when sodium salts of fatty acids were used, suggesting that Kolbe electrolysis proceeds by the same mechanism as that in the case of using water-soluble carboxylic acids.
Figure 2. Chromatograph of hexane solution extracting liposoluble components in electrolysis with R-COONa (T = 20 °C, E = 20 V, Q = 1000 C).
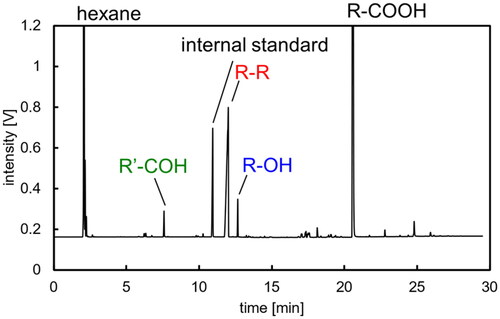
Next, based on the analysis results described above, the conversion, X, and yield of each component, Y, was calculated by EquationEqs. (4)(4)
(4) and Equation(5)
(5)
(5) .
(4)
(4)
(5)
(5)
where CR-COONa,0 is the initial R-COONa concentration and Ci is the concentration of the component i. shows the results in comparison with the results of electrolysis experiments conducted in the same manner using fatty acids. Because fatty acids are not dissolved in water, electrolysis experiments were conducted up to Q = 1000 C with the reactant floating on the water surface. In the samples collected via hexane extraction, only very small peaks of the products were observed, indicating that Kolbe electrolysis hardly progressed. In both experiments, the conversion and yield were almost in agreement, confirming that no gas products were generated due to excessive decomposition. Therefore, even if the carbon chain length was long, the fatty acid salt dissolved in water and the Kolbe electrolysis reaction proceeded as expected.
Figure 4. Conversion and yields of R-COOH and R-COONa in electrolysis (T = 20 °C, E = 20 V, Q = 1000 C).
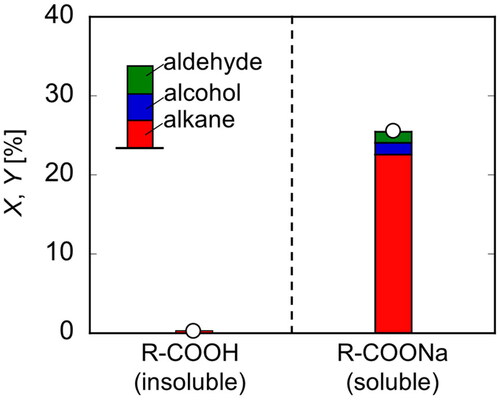
Next, using sodium fatty acid with a carbon chain length of 8, electrolysis experiments were performed by changing the reaction time, that is, the amount of electricity, and the time course of each component concentration and solution pH was observed. The results are shown in . Until approximately 60 min (2000 C) after the reaction started, the fatty acid ion concentration (diamond), which is the raw material, decreased. Accordingly, the concentration of hydrocarbons (circle) increased rapidly, and the concentrations of alcohols (square) and aldehydes (triangle) increased gradually. In addition, the pH (cross) of the reaction solution rapidly increased from 7.7 after the start of the reaction to 9.6 after 30 minutes (1000 C), suggesting formation of caustic soda as expected. However, despite the presence of fatty acid ions, neither product concentration nor pH changed after 60 min (2000 C). Hence, it may be possible that the flowing electrons were consumed in reactions other than Kolbe electrolysis.
Figure 5. Concentration profiles of reactant and product and pH in electrolysis of R-COONa (T = 20 °C, E = 20 V).
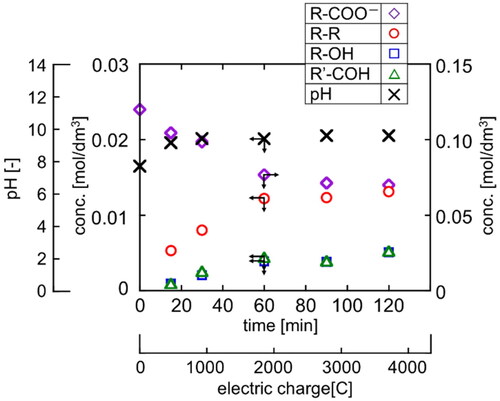
Consequently, the selectivity of electrons used for the desired Kolbe electrolysis among those transferred during the reaction is discussed. The current efficiency (CE) is defined as the ratio of the total amount of electrons, Q, that were transferred through the system to the amounts of electrons consumed by the desired Kolbe electrolysis reaction. It was calculated as follows:
(6)
(6)
Here, Qr was defined by EquationEq.(7)(7)
(7) , considering the production amount ni of the Kolbe electrolysis product i, the number of electrons Ne,i required for its production, and the Faraday constant F.
(7)
(7)
shows the time course of CE during electrolysis reaction. At the initial stage of the reaction, the CE was approximately 35%, however, it gradually decreased to approximately 15%. The operating voltage in this system was 20 V, which was sufficiently higher than the standard electrode potential of 1.23 V (Bratsch Citation1989) for water electrolysis. Therefore, the oxygen evolution reaction of EquationEq.(8)(8)
(8) is considered to have occurred simultaneously with Kolbe electrolysis at the anode.
(8)
(8)
As the Kolbe electrolysis progressed within this system, an increase in pH was observed. In addition, since the overvoltage of the oxygen evolution reaction decreases under basic conditions, The progression of oxygen evolution reaction is promoted (Teschke and Zwanziger Citation1982). In this system, the increase of pH in the reaction solution due to the progression of the Kolbe electrolysis is considered to have promoted the undesired oxygen evolution reaction. Therefore, it leads to a reduction in the selectivity for the Kolbe electrolysis.
3.2. Effects of operating factors on electrolysis
To determine the optimal conditions for efficiently obtaining the desired hydrocarbons, the effect of operating factors on reaction selectivity at 1000 C where the Kolbe electrolysis proceeds adequately was examined. shows the conversion and yield in each experiment. In addition, CE was also shown in diamond symbol. In both cases, the conversion and yield were almost the equivalent. As shown in (a), the yield increases with reaction temperature. However, as shown in (b), the yield tends to decrease as the voltage increases. As shown in (c), the yield reaches a maximum at a certain reactant concentration, however, the CE increases with the initial reactant concentration. Under high concentration conditions, the increase in current efficiency indicates that the amount of reacted fatty acid salts has increased. However, the larger effect of the increased amount of reactant present in the system is considered to be the reason for the yield showing a maximum value. Based on the above, it is considered that Kolbe electrolysis proceeds preferentially at high temperatures, low voltages, and high concentrations.
Figure 7. Conversion, yield and faradaic efficiency in electrolysis under various conditions at Q = 1000 C (Basic condition: T = 20 °C, E = 20 V, C = 0.12 mol/dm3).
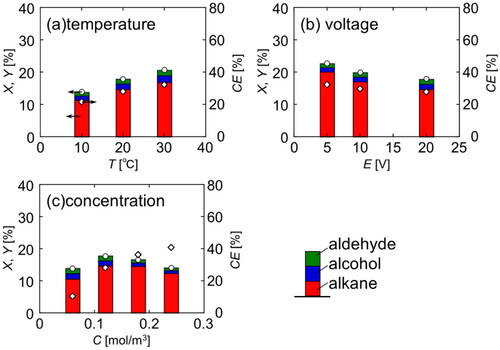
Based on the above results, the effect of operating factors is discussed. shows a schematic of the electrolysis mechanism proceeding on the anode. Kolbe electrolysis comprises two stages: the mass transfer of reactants from the liquid phase onto the anode and the electrolytic reaction on the anode. On the other hand, because there are enough amounts of hydroxide ions in the solution and their diffusion rate is large reaction (Agmon Citation2000), mass transfer can be neglected. Therefore oxygen-evolving reaction proceeds in only one step, the electrolytic reaction. The above-mentioned result that Kolbe electrolysis proceeded preferentially at high temperatures and concentrations is expected to be due to the promotion of the mass transfer of fatty acid ions, and this step is considered to be the rate-determining step of Kolbe electrolysis. Furthermore, when the voltage increased, the amount of electron transfer per unit time increased, and the amount of reaction on the electrode (total of Kolbe electrolysis and oxygen generation) increased. In this system, the progress of the Kolbe electrolysis reaction is restricted by the mass transfer rate control of the reactants. Therefore, oxygen evolution reaction proceeded preferentially without the effect of mass transfer.
3.3. Effect of raw material types on electrolysis
Next, an electrolysis experiment was conducted by varying the carbon chain length of the fatty acid salt. shows a photograph of the reaction solution when the experiment was performed at 20 °C, which is the same basic condition as described above. When using a fatty acid salt with a carbon chain length of 8 in (a), the reactant dissolved completely to form a transparent aqueous solution. After the reaction, the solution is kept in a transparent aqueous state, although hydrophobic products are suspended on the surface of the water. On the other hand, when the fatty acid salt with a carbon chain length of 12 (b) was used, the reaction solution was clouded with white. After a few minutes of reaction, the reaction solution became a gel without fluidity, did not conduct electricity. This is because the network formation between the oil droplets generated by the reaction and the reactants, leading to the gelling of the emulsion. This gelation occurs because hydrogen bonds form a cross-linked structure, which is more likely to occur at low temperature and become a sol at high temperature (Xian and Webber Citation2020).
Based on this knowledge, the reaction conditions were set to 50 °C, 5 V and 0.12 mol/dm3 and the electrolysis experiment as described above was performed. Under this temperature condition, even with a carbon chain length of 12, the reaction proceeded while maintaining the state of a transparent aqueous solution, as in the case of the carbon chain length of 8 in . It was reported that Kolbe electrolysis is preferred at low temperatures below 27 °C when water-soluble organic acids are used as raw materials (Klüh et al. Citation2021). However, for long-chain fatty acid salts, a high temperature was found to be preferable, which prevents gelation of the reaction solution. shows the experimental results of electrolysis. When C8 was used as the raw material, the conversion and yield increased compared to the results shown in (b), 5 V. As the carbon chain length used in the Kolbe electrolysis reaction increased, the conversion decreased. In addition, total product yield also decreased, and the alcohol and aldehyde yields tended to increase. At 50 °C, the yield was lower than the conversion, unlike at 20 °C. This difference became smaller as the carbon number increased in length, indicating that this was due to volatilization of the product.
Figure 10. Conversion, yield and faradaic efficiency of various fatty acid salt in electrolysis (T = 50 °C, E = 5 V, Q = 1000 C).
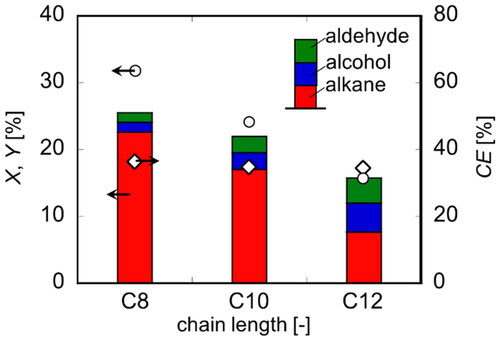
On the other hand, the CE was constant at approximately 40% regardless of the carbon chain length, indicating that the total amount of electricity used for Kolbe electrolysis did not change. As shown in above, the number of electrons required to produce a dimer, an alcohol, and an aldehyde per molecule of fatty acid ion are 1, 2, and 4, respectively. This means that even if the CE remains constant, the total yield decreases as the alcohol or aldehyde selectivity increases. To improve the energy efficiency in this system, it is effective to increase the selectivity of dimers with fewer number of electrons required for generation. Therefore, it is important to promote the mass transfer of R on the anode surface into the bulk liquid phase.
This study envisions the product hydrocarbons and other products to be used as biofuels. As mentioned above, an increase in the carbon chain length of the raw fatty acid salts resulted in a decrease in yield. On the other hand, the carbon chain length increase contributes to the increase in calorific value per mole of products. Therefore, the energy balance was evaluated using the lower heating value, assuming that the products in each experiment were used as fuels for power generation. The lower heating value was calculated using EquationEq.(9)(9)
(9) .
(9)
(9)
Here, power generation efficiency value of 44.9% was used (Song et al. Citation2018). Furthermore, the amount of input energy was calculated using EquationEq.(10)(10)
(10) .
(10)
(10)
shows the lower heating values of the products. For comparison, the input energy is shown as a dashed line. When fatty acid salts with carbon chain lengths of 8 and 10 are used, the same level of formation energy is obtained. In the case of the carbon chain length of 12, the amount of energy was approximately 90% of the carbon chain lengths of 8, although it decreased slightly. For any of the carbon chain lengths, the energy obtained was more than the input energy. The increase in carbon chain length leads to the effect of decreasing total yield. However, this is offset by an increase in energy per mole of product. Therefore, the application of Kolbe electrolysis suggests the possibility of establishing a method to process organic acid salts independently without any external energy input.
4. Conclusion
With the aim of developing an effective utilization method of soapstock discharged in large quantities in vegetable oil industry, the electrolysis mechanism and applicability of Kolbe electrolysis were examined. As a result, although the reaction proceeded even with a water-insoluble organic acid if it was a salt, the progress of electrolysis reached a plateau in the latter stage of the reaction due to the generated alkali. In the initial stage of the reaction, when electrolysis proceeded adequately, the effect of operating factors was investigated. And, it was found that the desired Kolbe electrolysis reaction proceeded preferentially at high temperatures, low voltages, and high concentrations. In addition, when the effect of the carbon chain length of the organic acid salt was examined, it was found that the longer the chain length, the reaction selectivity changed, and the sequential reactions on the electrode tended to proceed. Increasing the chain length of the organic acid slightly decreased the yield. but it contributes increasing the calorific value of the resulting product. Consequently, it was clarified that the amount of heat of the obtained products could be kept at almost the same level, and in any case, it was larger than the energy input for electrolysis. By introducing a separating system for the generated alkali, recycling of the alkali can be expected at the same time as the electrolytic reaction. Furthermore, the separation of the generated alkali is expected to prevent an increase of the pH in the reaction solution and promote Kolbe electrolysis. By establishing these reaction-separation systems, it will become a sustainable and effective utilization process that independently treat soapstock without energy input from the outside.
Disclosure statement
No potential conflict of interest was reported by the author(s).
Additional information
Funding
References
- Agmon N. 2000. Mechanism of hydroxide mobility. Chem Phys Lett. 319:247–252. doi: 10.1016/S0009-2614(00)00136-6.
- Barbusiński K, Fajkis S, Szeląg B. 2021. Optimization of soapstock splitting process to reduce the concentration of impurities in wastewater. J Cleaner Prod. 280:124459. doi: 10.1016/j.jclepro.2020.124459.
- Biermann U, Bornscheuer U, Meier MAR, Metzger JO, Schäfer HJ. 2011. Oils and fats as renewable raw materials in chemistry. Angew Chem Int Ed Engl. 50:3854–3871. doi: 10.1002/anie.201002767.
- Bratsch SG. 1989. Standard electrode potentials and temperature coefficients in water at 298.15 K. J Phys Chem Ref Data. 18:1–21. doi: 10.1063/1.555839.
- Creusen G, Holzhäuser FJ, Artz J, Palkovits S, Palkovits R. 2018. Producing widespread monomers from biomass using economical carbon and ruthenium–titanium dioxide electrocatalysts. ACS Sustainable Chem Eng. 6:17108–17113. doi: 10.1021/acssuschemeng.8b04488.
- Haas MJ. 2005. Improving the economics of biodiesel production through the use of low value lipids as feedstocks: vegetable oil soapstock. Fuel Process Technol. 86:1087–1096. doi: 10.1016/j.fuproc.2004.11.004.
- Klüh D, Waldmüller W, Gaderer M. 2021. Kolbe electrolysis for the conversion of carboxylic acids to valuable products—a process design study. Clean Technol. 3:1–18. doi: 10.3390/cleantechnol3010001.
- Meng X, Xu H, Zheng Y, Luo J, Huang S. 2022. Electrochemical decarboxylative oxygenation of carboxylic acids. ACS Sustainable Chem Eng. 10:5067–5071. doi: 10.1021/acssuschemeng.2c01495.
- Qiu Y, Lopez-Ruiz JA, Zhu G, Engelhard MH, Gutiérrez OY, Holladay JD. 2022. Electrocatalytic decarboxylation of carboxylic acids over RuO2 and Pt nanoparticles. Appl Catal, B. 305:121060. doi: 10.1016/j.apcatb.2021.121060.
- Qiu Y, Lv Y, Tang C, Liao J, Ruan H, Sotto A, Shen J. 2021. Sustainable recovery of high-saline papermaking wastewater: optimized separation for salts and organics via membrane-hybrid process. Desalination. 507:114938. doi: 10.1016/j.desal.2021.114938.
- Sako T, Okajima I. 2008. Soapstock treatment equipment, soapstock treatment method, and production method for fertilizer derived from soapstock. Patent WO 2008/105035 A1. https://patentimages.storage.googleapis.com/95/c0/aa/27699409d26def/WO2008105035A1.pdf.
- Song Q, Wang Z, Li J, Duan H, Yu D, Liu G. 2018. Comparative life cycle GHG emissions from local electricity generation using heavy oil, natural gas, and MSW incineration in Macau. Renewable Sustainable Energy Rev. 81:2450–2459. doi: 10.1016/j.rser.2017.06.051.
- Spínola AC, Pinheiro CT, Ferreira AGM, Gando-Ferreira LM. 2021. Mineral carbonation of a pulp and paper industry waste for CO2 sequestration. Process Safety Environ Protect. 148:968–979. doi: 10.1016/j.psep.2021.02.019.
- Teschke O, Zwanziger M. 1982. Operation of a steady-state pH-differential water electrolysis cell. Int J Hydrogen Energy. 7:933–937. doi: 10.1016/0360-3199(82)90161-6.
- Xian S, Webber MJ. 2020. Temperature-responsive supramolecular hydrogels. J Mater Chem B. 8:9197–9211. doi: 10.1039/D0TB01814G.
- Yu M, Zhang C, Li X, Liu Y, Li X, Qu J, Dai J, Zhou C, Yuan Y, Jin Y, et al. 2022. Pyrolysis of vegetable oil soapstock in fluidized bed: characteristics of thermal decomposition and analysis of pyrolysis products. Sci Total Environ. 838:155412. doi: 10.1016/j.scitotenv.2022.155412.
- Zhang B, Gao Y, Hioki Y, Oderinde MS, Qiao JX, Rodriguez KX, Zhang H-J, Kawamata Y, Baran PS. 2022. Ni-electrocatalytic Csp3–Csp3 doubly decarboxylative coupling. Nature. 606:313–318. doi: 10.1038/s41586-022-04691-4.