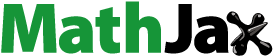
Abstract
The evaluation of the structural integrity of a vertical cask that is used for spent nuclear fuel dry storage is reported. The cask diameter and height are 3.566 m (140 in.) and 5.28 m (207.75 in.), respectively. The analysis focuses on such a cask being impacted by a commercial airplane. The dry storage container standards, which are under evaluation and approval by the U.S. Nuclear Regulatory Commission, are considered. The storage container inner basket is made of a stainless steel plate cylinder. It is located within an outer shell. The last one is manufactured with concrete and has internal and external steel liners. The commercial airplane considered in this analysis has a length of 40.39 m (132 ft, 6 in.). Its wingspan and height are 35.23 m (115 ft, 7 in.) and 11.98 m (39 ft, 4 in.), respectively. Its take-off weight is 81 090 kg (178 773 lb).
An explicit analysis with the finite element method is carried out. The impact angles were 0, 30, 45, and 60 deg with respect to the horizontal. The mesh of the domain has 1 104 229 hexahedral elements and 1 516 156 nodes. Initially, all the structures are considered without restrictions and free of stresses. The vertical container for dry storage is at rest on a rigid concrete base. The aircraft velocity is 234 m/s or 842 km/h (523 mph). The impact event is simulated in an interval of 0.03 s. The maximum principal stress fields show that there are points at the lid of the container that are above the elastic limit and the ultimate strength. Under these conditions, brittle failure is expected.
I. INTRODUCTION
The Electric Power Research Institute[Citation1] has mentioned that due to concerns about nuclear nonproliferation and for political reasons, the reprocessing of spent fuel stopped in the mid-1970s. The spent nuclear fuel (SNF) cooling pools of nuclear power plants were not designed to store the entire inventory of nuclear fuel. Their capacity has been exceeded.
The Electric Power Research Institute[Citation1] has stated that the independent spent fuel storage installation is an alternative, which is in accordance with the Law on Nuclear Waste Policy of 1982. The U.S. Nuclear Regulatory Commission[Citation2] has developed an independent regulatory framework for the storage of SNF outside the cooling pools and the reactor (10 CFR 72). These dry storage containers (DSCs) have been designed to provide physical protection, to contain radiation, and to passively remove heat during storage.
The SNF dry storage casks have been designed against earthquakes, tornadoes, free fall, penetration, extreme temperatures, and immersion.[Citation3,Citation4] Hanifehzadeh et al.[Citation5] tested the containers by producing a tipping event at an angular impact velocity during a seismic event. Tung-Yueh et al.[Citation6] analyzed on a small scale the straight (0 deg) and the oblique (5 deg) impacts on a dry storage barrel. The numerical analysis was carried out with the nonlinear finite element code LS-DYNA.
Results have been reported on issues related to a missile and an aircraft impacting nuclear installations. Siefert et al.[Citation7] made a model of an aircraft to analyze the impact on the wall of a building in a nuclear power plant. Ornai et al.[Citation8] analyzed the combined effect of bomb fragmentation and blast loading over the structure and the main reactor components. A cylindrical fragmentation model and the blast model of Kingery-Bulmash were considered, assuming a hemispherical charge. Zhu et al.[Citation9] analyzed a steel plate–concrete containment against a commercial aircraft impact. The nonlinear dynamic analysis was made with the force time–history method. The numerical model was made with the ANSYS/LS DYNA code. Impact displacements, plastic deformation, concrete damage, internal energy, and energy absorbed in the containment were evaluated.
Almomani and coworkers[Citation10] evaluated the risk to the structure of a metal storage container for spent fuel in an aircraft crash with the LS-DYNA code. The container materials were considered elastic plastic.
Bangash[Citation11] proposed a methodology for the impact evaluation of an aircraft on nuclear structures. He suggested some guidelines for a finite element method evaluation and the ultimate limit state analysis.
Previous results of this paper have been reported in Hernández-Palafox et al.[Citation12] An analysis of the impact of a light aircraft on a DSC for SNF was carried out. The main issue was the generation of the meshes for the container and the aircraft. Based on this, the considerations for their discretization and the selection of the parameters for the dynamic analysis, such as time step, were established.
II. STATEMENT OF THE PROBLEM
The structural integrity of a SNF container was evaluated when it was impacted by a commercial aircraft. Dynamic loading conditions were developed in this postulated case. The impact was totally inelastic, and the material failed. This evaluation was carried out with the Explicit Dynamics (ED) module of ANSYS®. The nonlinear dynamic analysis can be done.[Citation13]
The Electric Power Research Institute[Citation1] has mentioned that the inner basket is made of a stainless steel plate cylinder (). Woodward and Springman[Citation14] mentioned that the inner basket is located within an outer shell. It is manufactured with steel-concrete-steel. The concrete thickness varies between 0.6 to 0.7 m (23.62 to 27.56 in.). It has interior and exterior steel liners. Each one is 0.0254 m (1 in.) thick. The diameter, height, and mass of the storage system are 3.566 m (140 in.), 5.28 m (207.75 in.), and 193 094 tonnes (426 700 lb), respectively.[Citation15]
A commercial airplane is a large aircraft. It is used to transport people or merchandise and complies with the regulations of an international airport. The narrow-body aircraft is common.[Citation16] Its average length, wingspan, and height are 40.39 m (132 ft, 6 in.), 35.23 m (115 ft, 7 in.), and 11.98 m (39 ft, 4 in.), respectively. The take-off weight is 81 090 kg (178 773 lb), and the cruising velocity is 942 km/h (523 mph).
The coupling of two different geometries was necessary to carry out in the impact event. All the structural components of the container were modeled as solid bodies, and the aircraft was modeled as a shell or thin-walled body. The generation of adequate elements was essential in the discretization of the domain to avoid the nonphysical deformation modes of zero energy, which do not generate stresses.[Citation17]
III. METHODOLOGY
The following steps were followed:
The models of a container and a commercial aircraft were made with the CATIA V5® code.
The coupling of the solid geometries and surfaces was carried out in a single graphics window by means of the SpaceClaim code. In this way, it was possible to locate the commercial aircraft at different impact angles.
The initial loading and boundary conditions were determined. The NUREG-1864,[Citation18] NUREG-1536,[Citation19] NUREG-2125,[Citation20] and the American Society of Mechanical Engineers’ ASME Boiler and Pressure Vessel Code, Sec. III, Division 3[Citation21] were considered.
Impact simulations were carried out with 0-, 30-, 45-, and 60-deg impact angles by means of ED.
IV. THEORETICAL BACKGROUND
The partial differential equations that relate the conservation of momentum with the acceleration and the stress tensor σij are the following:
The energy conservation is evaluated with the following equation:
Becker et al.[Citation22] stated that the numerical temporal integration of the equations can be done explicitly or implicitly. These equations were solved explicitly for each element of the model in the problem of interest. The main advantage of this integration method is the possibility of solving each time step locally. It is not required to solve a system of equations where all the degrees of freedom are involved. Belytschko et al., cited by O’Sullivan and Bray,[Citation23] argue that explicit time integration is well suited to dynamic contact/impact problems because the time steps are small. The accuracy of the results is conditioned by the steps of time.
The basic formulation of an explicit integration allows certain advantages in the time integration of linear and nonlinear problems, such as the decoupling of the equations. It can be solved directly, and convergence evaluations are not required. The convergence is guaranteed unless there are distorted elements or energy errors in the model.[Citation13] Islam and Singh[Citation24] stated that hourglass modes are nonphysical distortion modes of zero energy. They affect the accuracy of the solution.
The size of the time step in an explicit time integration is limited by the Courant-Friedrichs-Lewy (CFL) condition.[Citation25] It is proportional to the smallest dimension of the element in the model and inversely proportional to the velocity of sound in the material. In this way, a stress wave cannot travel beyond the length of the smallest element in a single time step.[Citation13,Citation25] The time step is obtained with the following equation:
where h is the length of the element, c is the speed of sound in the material, and f is the safety factor. Under these conditions, the stability and accuracy of the solution are guaranteed.
Time increments can be set to 1 μs. However, several computational cycles are required to obtain the solution. The time step can be set by the user or controlled by the program itself. The program-controlled option automatically detects the best default options for the evaluation.[Citation13,Citation25] For this case, the time step was controlled by the solver.
The explicit solver uses the central difference time integration scheme (Leapfrog method). Once the forces have been calculated at the nodes, the nodal accelerations are calculated by dividing the force by the mass,
where are the components of the nodal acceleration (i = 1, 2, 3), Fi are the forces acting on the nodes, bi are the components of the acceleration of the body, and m is the mass of the node.[Citation13] With accelerations at time n − 1/2 determined, the velocities at time n + 1/2 are found from
The solution of a dynamic explicit analysis requires that the partial differential equations of mass conservation, momentum, and energy are expressed in Lagrange coordinates. The mesh moves and distorts with the material of the model, so the conservation of mass is automatically satisfied. The density at any time can be determined from the current volume and its initial mass,
V. NUMERICAL ANALYSIS
The models of the vertical container for dry storage of SNF and the commercial aircraft were developed with a workstation with two 3.2-GHz processors, 64 GB RAM, and 32 cores.
V.A. Model of a Vertical Container for Dry Storage of SNF
The vertical container for dry storage of SNF is manufactured with different components, as shown in . Woodward and Springman[Citation14] state that the DSC has been designed to provide physical protection to the SNF.
The finite element meshes of the main components were developed with the Part Design tool in Catia V5. The representative details of the inner basket, the fuel elements, the lid, and the outer shell of the container with its two metal liners were considered. They were assumed to be independent bodies. The explicit integration of the ANSYS ED module allows for the equations to be decoupled. They can be solved independently (explicitly).[Citation13] Inversion of the stiffness matrix is not required. Contact nonlinearities are included in the internal force vector. Bolts and welds were not considered as their dimensions are small compared to the elements mentioned previously.
The small geometries would cause errors in the evaluation of the energy and the use of erroneous time steps. The physical and mechanical properties of the materials of these components are summarized in .
TABLE I Mechanical and Physical Properties of the Materials of the Main Components of the Nuclear Spent Fuel DSC
V.B. Model of a Commercial Aircraft
The characteristics of the commercial aircraft considered are summarized in .
TABLE II Characteristics of a Commercial Airplane[Citation16]
The commercial aircraft model was developed with the CATIA V5 code. It was done with the Generative Shape Design module, which generates a wireframe geometry. This is the guide or reference of lines and curves to create surfaces. The model configuration was made with surface-type elements. Its thickness was 0.03988 m (1.57 in.). The main interest was to evaluate the structural integrity of the DSC, so the relevant aspects of the airplane were only considered. Its mass, which was 82 417 kg (181 698.4 lb), was simulated with this mesh. shows a complete view of the aircraft model just before the impact with the container and the boundary conditions. The vessel was fixed to the ground.
The material of the model of the commercial aircraft was aluminum alloy 5083-H116. It is used in air transport due to its good resistance to corrosion. Its mechanical and physical properties are summarized in .
TABLE III Mechanical and Physical Properties of Aluminum Alloy 5083-H116[Citation29]
V.C. Main Characteristics of the Finite Element Mesh
Regarding the rollover analyses of the DSC, Hanifehzadeh and coworkers[Citation5] and the NUREG 1864[Citation18] have considered that containers are impacted on a rigid base. In some cases, this situation takes place in friction-free conditions. Based on these assumptions, the vertical container was placed on a rigid concrete base. shows the discretization of the domain; 1 104 229 hexahedral elements and 1 516 156 nodes were required. The Appendix reports the types of mesh drivers for each one of the components involved in the analysis, as well as the number of nodes and elements for such components.
V.D. Initial Conditions
All the structures were unstressed. The velocity of the aircraft was 234 m/s or 842 km/h (523 mph). The impact event was simulated in 0.03 s. In accordance with our experience,[Citation12] this time interval was considered adequate.
V.E. Evaluation of the Impact
A nuclear spent fuel dry storage cask has several components. However, the structural elements that directly maintain the confinement of the spent fuel were considered; in this case, the outer casing of the container, the lid, and the inner basket. Regarding the airplane impact, four attack angles with respect to the floor level were considered: 0, 30, 45, and 60 deg. The evaluations were carried out with the ANSYS ED code.
V.E.1. Analysis of the Drastic Drop of the Commercial Aircraft Velocity
The approach velocity of the aircraft was 234 m/s (523 mph) at the beginning of impact. However, its velocity decreased drastically in 1.50E-03 s after the impact at different angles of attack. The results are summarized in .
Fig. 4. Drastic drops in the velocity of the commercial aircraft when it impacted a vertical container at different angles of attack.

An energy evaluation was carried out when a horizontal impact has taken place (). A total of 21 points were evaluated in a time interval of 9.86E-02 s after impact. The kinetic energy of the aircraft before impact was 2.25E+09 J and it was 1.16E+09 J at the end of the period. The kinetic energy was reduced as the impact on the container developed. Hibbeler[Citation26] indicated that energy was stored internally as the impacted body deformed. The blue curve in shows the increment of the internal energy.
Hourglass modes can be controlled with a mesh refinement. In this case, a mesh was generated with elements of constant dimensions and volumes. The CFL criterion and the smallest thickness of the vessel components were considered. These conditions assured that the hourglass energy was less than 10% of the total internal energy of the system (). The hourglass control forces artificially dissipate energy from the system when the hourglass energy is greater than 10% of the internal energy.[Citation17,Citation24]
V.E.2. Distribution of the Dirac Delta
The resistance to the movement of the DSC on a surface, which has friction R, is proportional to the velocity. The movement is under the action of an external force F(t). It can be represented by the following ordinary differential equation[Citation30]:
A first-order differential equation has the following form y’ + ay = 0. Its solution is in the interval (-∞, ∞).[Citation30] EquationEquation (9)
(9)
(9) can be rewritten in the following way:
The relationship is constant,
Its solution, in a short time interval, is
A is important in the domain of the Dirac Delta δ(t – t0) because it indicates the effect of the impact on the container.[Citation31] Integrating EquationEq. (9)(9)
(9) ,
The mass was multiplied by the velocity in a defined time interval. This is equal to the impulse minus the force of friction. The velocity of the container was not high. The friction force R was smaller than F(t), and it was not considered during ∆t ().
For the case of interest, two instants were considered. The first was before the impact, and the second was when the impact force acted on the container:
, before the impact, where
:
(14)
(14)
If the solution of EquationEq. (12)
(12)
(12) is considered, the following relationship is obtained:
(15)
(15)
If A = I (impulse), the impact velocity is defined with EquationEq. (16)(16)
(16) :
where v(t) = I/m is the variation of the velocity of the container when it is impacted by an aircraft (). It is based on the arguments mentioned previously and the results obtained in the numerical evaluation of the impact. The maximum velocity of the container after impact was 1.01E+02 m/s. Then it decayed.
The impulse force I is defined as in Ref. [Citation32]. It depends on the velocity v(t). The resultant force ΣF on the container is constant in an interval of time Δt,
The magnitude of the impact force changed through time. It is an exponential function. The maximum force was F = 1.29E+10 N and took place in the time interval between t1 = 0 s and t2 = 1.50E-03 s in a horizontal impact (). Then it decayed. In the first phase, greater stresses developed, which can lead to brittle failure. When the force decayed, an energy dissipation took place.
VI. ANALYSIS OF THE RESULTS
shows the maximum principal stress field in a horizontal impact. A time interval of 3.00E-02 s was considered. The maximum principal stress was1.6E+09 Pa. It was developed at the cap shell. The yield and ultimate strengths were lower than the maximum principal stresses. Dynamic loading and high strain rates developed before the peak stresses took place. Under this condition, the materials tend to be fragile because their capacity to absorb energy is reduced. Brittle fracture is expected on the impact spot. The generation of missiles can also take place. The structural integrity of the container was not kept. The stresses varied during the interval of evaluation on those areas that were unbroken during the unloading. A similar situation has been observed in other cases of interest.
The maximum principal stress field, generated at 1.50E-03 s after a 30-deg impact, is shown in . The maximum principal stress was 1.97E+09 Pa. Bending conditions took place, and the resistant moments were at the base of the DSC.
The maximum principal stress fields of the 45- and 60-deg impacts are shown in and , respectively. Similar conditions developed. In the first case, the maximum principal stress was 2.05E+09 Pa. In the second case, it was 2.03E+09 Pa.
compares the maximum principal stresses that were obtained in all the cases of interest. The results are in the same range. Two phases can be observed. Dynamic loading takes place in the first phase, the energy dissipates in a longer period during the second phase.
Fig. 13. Maximum principal stresses at the impact spot on the DSC when the initial impact velocity was 842 km/h (523 mph).

Concrete is confined between the external and internal steel liners of the wall of the container. The best strength was obtained when there was a good adherence between both materials. This situation was considered in the damage analysis. Voids between both materials reduced the strength of the DSC.
Abdel-Kader[Citation33] stated that the Riedel-Hiermaier-Thoma (RHT) material model is widely used for concrete. It is capable of describing the behavior of concrete under impact loads. Explicit dynamic analyses use models such as the RHT to estimate the damage that occurs in concrete. The damage range (DAMAGEALL) for the ANSYS ED is between 0.0 and 1.0. The first case means that the material is completely intact, while 1.0 means that the material is completely damaged.[Citation34]
Concrete is a brittle material. illustrates the damage to the concrete wall of the container when the impact took place with a 45-deg attack angle. This evaluation was carried out when peak stresses took place. The damage developed during the dynamic loading.
Fig. 14. Damage developed on the concrete wall of the container after an impact with a 45-deg angle.

As can be seen in , the impact area was destroyed (red zone). Besides, there are some points at the base that could be in a similar situation. The impact generated bending moments that were resisted at this location. However, there are some areas in the bottom of the container that were not destroyed.
The vessel was manufactured with two materials. The internal and external layers of the container are made with steel, which is ductile. It is expected that this material absorbs part of the impact energy and improves the total resistance.
Finally, it was considered that an aircraft reduces its velocity considerable from its cruising speed as it approaches the ground. A final evaluation was carried out. In this case, a horizontal impact, in which the velocity was 67.056 m/s (150 mph), was considered. shows the maximum principal stress field. It is similar to the cases discussed perviously. The maximum principal stress was 1.533E+09 Pa.
Fig. 15. Maximum principal stress field after a horizontal impact. The aircraft velocity was 67.056 m/s (150 mph).

illustrates the damage to the concrete wall at the impact point. Severe conditions took place in the neighborhood of this area. Besides, there was stress concentration at the bottom of the vessel. The structural integrity was not kept.
VII. CONCLUSIONS
A spent fuel dry storage cask is a concrete vessel that has external and internal steel liners. The interaction of both materials improves its strength, and it can keep its structural integrity at different loading conditions. Regarding the postulated cases, the contact surfaces of both materials were considered without any sliding.
The dynamic load took place in a short interval of time. An explicit evaluation with the finite element method showed the damage that developed on a nuclear spent fuel dry storage cask. The balance of energy showed that the hourglass energy was at an acceptable level. It can be considered that the numerical evaluations were appropriate.
Brittle fracture was expected, as a high level of energy was released. Missiles can be generated after impact. Extreme damage can be anticipated at the point of collision and on the base of the vessel.
Acknowledgments
The authors kindly acknowledge the grant for the development of Project 211704, which was awarded by the National Council of Science and Technology (CONACyT). All the authors recognize the contributions of Dr. Pablo Ruiz-López to this research.
Disclosure Statement
No potential conflict of interest was reported by the authors.
Correction Statement
This article has been corrected with minor changes. These changes do not impact the academic content of the article.
Additional information
Funding
References
- “Impacts Associated with Transfer of Spent Nuclear Fuel from Spent Fuel Storage Pools to Dry Storage After Five Years of Cooling, Revision 1,” EPRI 1025206 Technical Report, Electric Power Research Institute (2012).
- “Dry Cask Storage of Spent Nuclear Fuel,” Backgrounder, Office of Public Affairs, U.S. Nuclear Regulatory Commission; https://www.nrc.gov/docs/ML0622/ML062200058.pdf (accessed Mar. 21, 2018).
- L. M. RUIZ and H. G. OROZCO, “Containers for Temporary Storage and Transportation of Spent Fuel,” Nuclear Safety and Radiological Protection Magazine (2015); https://www.csn.es/documents/10182/13557/Alfa+27 (accessed May 20, 2018).
- “Safety of Spent Fuel Transportation,” NUREG/BR-0292, Rev. 2, U.S. Nuclear Regulatory Commission (2017); https://www.nrc.gov/docs/ML1703/ML17038A460.pdf (accessed May 28, 2018).
- M. HANIFEHZADEH, B. GENCTURK, and K. WILLIAM, “Dynamic Structural Response of Reinforced Concrete Dry Storage Casks Subjected to Impact Considering Material Degradation,” Nucl. Eng. Des., 325, 192 (Dec. 2017); http://dx.doi.org/10.1016/j.nucengdes.2017.10.001.
- W. TUNG-YUEH, L. HSUEH-YUAN, and K. LONG-CHYUAN, “Dynamic Response Analysis of a Spent-Fuel Dry Storage Cask Under Vertical Drop Accident,” Ann. Nucl. Energy, 42, 18 (2012); http://dx.doi.org/10.1016/j.anucene.2011.12.016.
- A. SIEFERT, F.-O. HENKEL, and E. ALDOGHAIM, “Modelling of Aircraft Cessna 210 for Impact Analysis on Nuclear Building,” presented at the 23rd Conf. Structural Mechanical Reactor Technology (2015); https://repository.lib.ncsu.edu/bitstream/handle/1840.20/33987/SMiRT-23_Paper_580.pdf?sequence=1&isAllowed=y (accessed Aug. 29, 2018).
- D. ORNAI et al., “A Methodology of Risk Assessment, Management, and Coping Actions for Nuclear Power Plant (NPP) Hit by High-Explosive Warheads,” Adv. Eng. Inf., 46, 101192 (Oct. 2020); http://dx.doi.org/10.1016/j.aei.2020.101192.
- X. ZHU et al., “Sensitivity Analysis of Steel-Plate Concrete Containment Against a Large Commercial Aircraft,” Energies, 14, 2829 (2021); http://dx.doi.org/10.3390/en14102829.
- B. ALMOMANI, S. LEE, and H. GOOK KANG, “Structural Analysis of a Metal Spent-Fuel Storage Cask in an Aircraft Crash for Risk Assessment,” Nucl. Eng. Des., 308, 60 (2016); http://dx.doi.org/10.1016/j.nucengdes.2016.07.014.
- M. Y. H. BANGASH, Structures for Nuclear Facilities: Analysis, Design, and Construction, Springer-Verlag Berlin Heidelberg, London (2011); https://books.google.com.mx/books?hl=es&lr=&id=SOF6ZQfxulUC&oi=fnd&pg=PR3&dq=Structures+for+Nuclear+Facilities:+Analysis,+Design,+and+Construction&ots=7rJasJISJX&sig=-nQbX8eQXlz8MEmaBVsXqMJ9d40#v=onepage&q=Structures%20for%20Nuclear%20Facilities%3A%20Analysis%2C%20Design%2C%20and%20Construction&f=false (accessed July 5, 2020).
- E. HERNÁNDEZ-PALAFOX et al., “Analysis of an Aircraft Impact on a Dry Storage Cask of Spent Nuclear Fuel,” Engineering Design Application III: Structures, Materials and Processes, A. ÖCHSNER and H. ALTENBACH, Eds., pp. 91–102, Springer International Publishing (2020); http://dx.doi.org/10.1007/978-3-030-39062-4_9.
- “Module 02: Introduction to Explicit Dynamics—Introduction to ANSYS Explicit Dynamics,” Customer Training Material, pp. 1–62, ANSYS, Inc. (2019).
- S. W. WOODWARD and M. R. SPRINGMAN, “The Evolution of Spent Nuclear Fuel Storage. Policy and Technology in the US,” World Nuclear & Radiation, Information of Holtec International, Seoul, Korea (June 2017).
- “ Task Order 16: Generic Design Alternatives for Dry Storage of Spent Nuclear Fuel,” U.S. Department of Energy (2015); https://www.energy.gov/sites/prod/files/2016/10/f33/to16-final-report_0.pdf (accessed Oct. 30, 2018).
- “Aircraft Specifications,” Delta Cargo (2018); https://www.deltacargo.com/Cargo/catalog/aircraft-specification?from=home (accessed Oct. 29, 2019).
- S. HALE, “Why Worry about Hourglassing in Explicit Dynamics? Part 1 2015,” Forbrukslånsiden.com (2015); https://caeai.com/blog/why-worry-about-hourglassing-explicit-dynamics-part-i (accessed Oct. 10, 2018).
- “A Pilot Probabilistic Risk Assessment of a Dry Cask Storage System at a Nuclear Power Plant,” NUREG-1864, U.S. Nuclear Regulatory Commission (2007); https://www.nrc.gov/docs/ML0713/ML071340012.pdf.
- “Standard Review Plan for Spent Fuel Dry Storage Systems at a General License Facility: Final Report,” NUREG-1536 Rev. 1, U.S. Nuclear Regulatory Commission (2010); https://www.nrc.gov/docs/ML1010/ML101040620.pdf.
- “Spent Fuel Transportation Risk Assessment: Final Report,” NUREG-2125, U.S. Nuclear Regulatory Commission (2014); https://www.nrc.gov/docs/ML1403/ML14031A323.pdf.
- “Boiler and Pressure Vessel Code, Division 3: Containments for Transportation and Storage of Spent Nuclear Fuel and High Level Radioactive Material and Waste—Rules for Construction of Nuclear Facility Components,” American Society of Mechanical Engineers (2010).
- P. BECKER, N. NIGRO, and S. IDELSOHN, “Explicit Time Integration with Large Time Steps for the Heat Flow Equations,” Int. J. Numer. Methods Calculation Des. Eng., 28, 187 (2012); http://dx.doi.org/10.1016/j.rimni.2012.07.001.
- C. O’SULLIVAN and J. D. BRAY, “Selecting a Suitable Time Step for Discrete Element Simulations that Use the Central Difference Time Integration Scheme,” Eng. Comput., 21, 2/3/4, 278 (2004); http://dx.doi.org/10.1108/02644400410519794.
- M. T. ISLAM and E. A. SINGH, “Study of Behavior of RCC Beam Under Impact Loading and Effect of Hourglass Energy by Finite Element Analysis Using ANSYS,” IJERT, 8, 7, 2278 (2019); http://dx.doi.org/10.17577/IJERTV8IS070027.
- ANSYS Explicit Dynamics Analysis Guide, pp. 131–140, ANSYS, Inc. (2019).
- R. C. HIBBELER, Mechanics of Materials, 10th ed., Pearson Education, Inc. (2017).
- “304 Stainless Steel,” MatWeb (2019); https://www.matweb.com/search/DataSheet.aspx?MatGUID=abc4415b0f8b490387e3c922237098da.
- “Zircaloy-2 Zirconium Alloy,” MatWeb (2019); http://www.matweb.com/search/DataSheet.aspx?MatGUID=eb1dad5ce1ad4a1f9e92f86d5b44740d.
- “Aluminum 5083-H116; 5083-H321,” MatWeb (2019); http://www.matweb.com/search/DataSheet.aspx?MatGUID=1efe7441a72f4a22a53c0dc1bd9c87ec.
- E. BUTKOV, Mathematical Physics, Addison-Wesley Publishing (1973).
- D. G. ZILL, Advanced Engineering Mathematics, 7th ed., Jones & Bartlett Learning (2020).
- H. D. YOUNG and R. A. FREEDMAN, University Physics with Modern Physics, 15th ed., Pearson (2020).
- M. ABDEL-KADER, “Modified Settings of Concrete Parameters in RHT Model for Predicting the Response of Concrete Panels to Impact,” Int. J. Impact Eng., 132, 103312 (2019); http://dx.doi.org/10.1016/j.ijimpeng.2019.06.001.
- “Workshop 05.3: Drop Test on Reinforced Concrete—Introduction to ANSYS Explicit Dynamics,” Customer Training Material, pp. 1–24, ANSYS, Inc. (2016).
APPENDIX
MAIN CHARACTERISTICS OF THE FINITE ELEMENT MESHES OF THE DSC AND THE AIRCRAFT
specifies the number of nodes and elements for each component and the mesh drivers that were used for each case.
TABLE A.I Characteristics of the Mesh Drivers for Each Component of the System