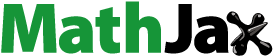
Abstract
Long-lived high-level waste from commercial nuclear power reactors is a problem that concerns stakeholders and scientists working in the back end of the nuclear fuel cycle. Nuclear waste transmutation is under investigation to tackle this problem, transforming nuclides that represent a long-term source of radioactivity, radiotoxicity, and heat into short-lived or stable nuclides. However, the transmutation process will require that several long-lived isotopes be separated from the spent nuclear fuel, which raises proliferation concerns.
In this paper, we perform an investigation of the attractiveness characteristics related to the material used in a lead-cooled fast reactor system concept designed to burn minor actinides before and after irradiation. The materials evaluated are separated uranium, neptunium, plutonium, americium, and curium. We also evaluated grouped product materials, neptunium + americium and neptunium + plutonium. Additionally, we present potential safeguards and physical protection implications for the proposed materials. The main conclusion of this paper is that the separated neptunium and plutonium generated by the fast reactor are materials that deserve attention mainly related to physical protection measures.
I. INTRODUCTION
Nuclear waste minimization is a step toward a sustainable future nuclear fuel cycle. The decay heat released, the long-term radiotoxicity, and the proliferation risk of plutonium (Pu) and minor actinides (MAs), such as americium (Am), neptunium (Np), and curium (Cm), are concerns related to the waste management of current light water reactor (LWR) systems.[Citation1]
Over the years, different options have been investigated to decrease the quantities of MAs in the final nuclear waste. Actinide recycling via partitioning followed by transmutation (P&T) via fission is considered the most effective technology.[Citation2] Partitioning refers to the separation of MAs and long-lived fission products from the spent nuclear fuel (SNF) through recycling, and transmutation refers to the fissioning or the process of neutron capture in actinides, resulting in the creation of short-lived or stable fission products.
Partitioning followed by transmutation requires access to facilities and processes that enable the separation of MAs and the fissioning of waste products. In practice, the separation step is envisaged to take place in fuel reprocessing or recycling facilities, and the transmutation step is foreseen to take place in a nuclear reactor. Because of the low fission-to-capture ratio of MAs in thermal nuclear reactors, international initiatives, such as INPRO (International Project on Innovative Nuclear Reactors and Fuel Cycles) and GNEP (Global Nuclear Energy Partnership), have demonstrated interest in fast reactor concepts.[Citation3]
Wallenius[Citation4] proposes a lead-cooled fast reactor concept that uses MA fuel for waste transmutation. The concept reactor makes use of Np and Am in a nitride matrix as nuclear fuel, (Np,Am)N. This in turn assumes the recycling of SNF from LWRs to get material streams for Np and Am. Although Ref. [Citation4] recognizes that separated Np may be useful for weapons production, it affirms that the spent fuel generated by the reactor concept would be proliferation resistant.
Since any advanced fuel cycle proposal should aim to use materials that present barriers against their use in the production of nuclear explosive devices (NEDs) or weapon material at all the considered steps,[Citation5] the present work is motivated by nonproliferation concerns. The objective of this work is to evaluate the attractivity of the materials involved in the proposed reactor system and its related nuclear fuel cycle. To do so, we make use of so-called figures of merit (FOMs) that relate material attractivity to intrinsic barriers of the material against diversion and its use in a NED. Furthermore, we discuss external measures to ensure material safeguardability and physical protection based on the material’s attractivity level results.
II. SELECTED NUCLEAR ENERGY SYSTEM
In this work, we consider the nuclear energy system shown in . The starting point is the operation of LWRs that produce SNF, which after cooling, is sent to a reprocessing facility for MA recovery using a commercially available reprocessing technique. The facility is assumed to recover U, Pu, Np, and Am. The safeguards and security aspects related to the U and Pu recovered from the LWR SNF have been thoroughly studied before and will not be considered here. The Np and Am are then sent to a fuel fabrication facility that chemically processes the materials and fabricates (Np,Am)N fuel destined for the lead-cooled fast reactor designed to transmute nuclear waste products.
Fig. 1. Flowchart of the fuel cycle steps considered in this work. The red dotted line indicates the facility of interest in this work, and hence, also the origin of the material in the attractiveness evaluation.
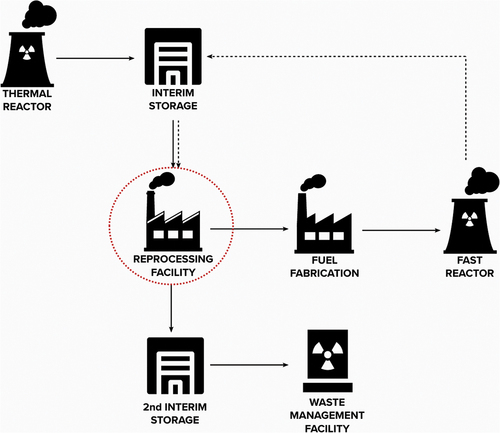
The reactor is designed to have a lifetime of 24 years without refueling.[Citation4] After 24 years, the fuel will be discharged from the reactor and temporarily stored before being sent back to the reprocessing facility. After being reprocessed (again), the separated nuclear material can either be recovered and used to fabricate new fuel for the lead-cooled fast reactor or sent to a waste management facility.
We consider all facilities to be part of a civilian fuel cycle and assume that the state in question has ratified a comprehensive safeguards agreement. Furthermore, we do not consider any material losses in any of the processes. To limit the scope of this work, we evaluate only materials in the reprocessing facility (highlighted with a red dotted line in ) due to their sensitive nature from a proliferation point of view, as separated materials are available in a pure form.
The material parts of the P&T fuel cycle that will be investigated are (1) separated Np and Am recycled originating in the SNF from the thermal nuclear reactors and (2) separated streams of Pu, U, and MAs recovered in the reprocessing of SNF from the lead-cooled fast reactor. While U and Pu are not present in the fresh fuel, they are produced as the reactor is operating. Since U and Pu may vary significantly from LWR SNF, these materials will be investigated.
II.A. Fast Lead-Cooled Reactor and its Fuel Properties
Wallenius[Citation4] presents a compact lead-cooled fast reactor designed for MA transmutation using (Np,Am)N fuel. The 4-MW(thermal) reactor is claimed to have a specific rate for transmuting actinides that is 40 times higher than the rate in large sodium-cooled reactors.[Citation4] The reactor is composed of
seven fuel assemblies, each containing 61 (Np,Am)N fuel rods
six control assemblies, each with 19 B4C rods
six shutdown assemblies composed of (W,Re)10B2
18 reflector assemblies composed of yttria-stabilized zirconia.
The fuel rods are composed of fuel pellets with a 13.4-mm diameter piled in 54-cm-tall and 0.5-mm-thick cladding tubes made of alumina-scale material.[Citation6,Citation7] The detailed information on the other assemblies can be found in Ref. [Citation4].
The composition of the fresh fuel is 33% 237Np and 67% Am, where the Am composition is 241Am and 243Am in a weight fraction of 85/15. [According to Ref. [Citation4], this fraction can be obtained from LWR spent fuel with 20 years cooling time (CT).] According to Ref. [Citation4], the total mass of fresh fuel necessary to start the reactor is around 373 kg (120 kg 237Np, 215 kg 241Am, and 38 kg 243Am). Although manufacturing of this fuel is outside the scope of the analyses in this work, one can note that the world inventory of 237Np and Am was estimated to be over 140 tonnes in 2003 (with an expected increase rate of 7 tonnes per year), but the great majority of this is contained in irradiated fuel and high-level nuclear waste.[Citation8]
The average discharge burnup of the lead-cooled fast reactor fuel is assumed to be 120 MWd/kg, assuming a reactor availability of 90% and a calculated power density of 15 kW/kg for the actinides.
III. MATERIAL ATTRACTIVENESS
The attractiveness of nuclear material for use in a NED is related to its intrinsic properties. A material’s isotopic composition and its physical form are explicitly used by the International Atomic Energy Agency (IAEA)[Citation9] to classify whether the material is directly useful in the manufacture of a NED. Materials such as Pu, 233U, and U with a 235U enrichment of >20% [highly enriched uranium (HEU)] are considered direct-use materials, while U with <20%235U and Th are considered to be indirect-use materials. Materials in metal form are considered more attractive than oxide materials, which are less attractive since they require conversion to metal form before use in a NED.[Citation5] This conversion process requires knowledge, technology, resources, and time.
In a NED, enough material is needed to have a self-sustaining chain reaction. This amount is called the critical mass. The so-called bare critical mass (BCM) is the minimum mass required to generate a self-sustaining fission reaction in the material surrounded by air. However, by surrounding the bare mass with a neutron-reflecting material, the critical mass can be reduced. As an example, the BCM of 239Pu is around 15 kg, but can be lowered to 8 kg using reflective material.[Citation9]
While some properties of nuclear materials make them attractive for use in NEDs, others make them less attractive for the same purpose. The latter are typically described as barriers, and such properties are, for instance, a high decay heat rate, dose rate, and spontaneous fission rate. A high decay heat rate would impose difficulties on the weapon design since it would require a heat dissipation device to keep the weapon functional. High dose rates would require shielding or regulated exposure time while handling the material, making acquisition and utilization challenging. The spontaneous fission rate can affect the nuclear yield produced by the NED since neutrons coming from spontaneous fission may initiate a fission reaction too early, causing a “fizzle” before achieving maximum compression, which also makes the weapon yield unreliable.
III.A. Figures of Merit
To evaluate the material attractiveness, one needs a measure of how strong the barriers are. Different approaches to evaluate the attractiveness of nuclear materials have been studied and developed by others. Approaches specifically related to Pu have been studied by Pellaud,[Citation10] Sagara et al.,[Citation11] Kessler,[Citation12] and Saito et al.[Citation13] A well-accepted methodology to evaluate the attractiveness of nuclear material in advanced fuel cycles, incorporating the already mentioned barriers (critical mass, decay heat content, dose rate, and spontaneous fission rate), was developed by Bathke et al.[Citation14] The authors proposed two different expressions to quantify the nuclear material attractiveness. These are referred to as FOMs and are shown in EquationEqs. (1)(1)
(1) and (Equation2
(2)
(2) ),
where
M | = | = BCM of the metal (kg), |
h | = | = decay heat content (W/kg), |
D | = | = radiation dose rate evaluated at 1 m from the surface (rad/h) |
S | = | = spontaneous fission neutron rate (n·(s·kg)−1). |
The difference between the two equations lies in the yield of the NED (the third term in FOM2), which is in turn connected to the potential proliferator. EquationEquation (1)(1)
(1) applies to a subnational group or technically advanced proliferating states, where the spontaneous fission rate is not considered a barrier. A subnational group may be satisfied with any yield, and it is assumed that a technically advanced State possesses the necessary technology to design a nuclear device, minimizing the impact of pre-initiation. EquationEquation (2)
(2)
(2) applies to less technically advanced proliferating states, where pre-initiation by spontaneous fission would be an issue. This would in turn require the use of nuclear material with a low spontaneous fission rate.
Both equations assume that the materials to be evaluated are in alloy or metal form when the metrics are assessed and that the necessary material transformations and conversions have been performed. The results of applying the analyses are numerical values of the FOMs, which Bathke et al.[Citation14] suggest should be interpreted in the following way:
FOM < 1: These materials are unattractive for use in a NED.
1 ≤ FOM ≤ 2: These materials are attractive for use in a NED.
FOM > 2: These materials are preferred for use in a NED.
III.B. FOM Uncertainty
Simplistically, the uncertainty in the FOM equations could arise from (1) the model itself, as any model will inevitably be a simplification of the reality it is designed to represent and (2) the uncertainty in the parameters used to feed the FOM equations. Since it is a mathematical model, the uncertainties in M, h, D, and S affect the FOM results. Unfortunately, the uncertainty in the FOM model is not presented in its original reference.[Citation14] The statistical uncertainty in each Monte Carlo calculation can be neglected, and a full uncertainty quantification is complex and beyond the scope of this paper. Instead, we here investigated the impact of an assumed 3% uncertainty in each of the parameters (M, h, D, and S). We then propagated the parameter uncertainty to the FOM results.
III.C. Material Selection
In this work, we evaluate the material attractiveness of materials in the selected nuclear energy system using the formulas for FOM1 and FOM2. We point out that although the (Np,Am)N fuel foreseen for the chosen reactor is unique and very different from most other reactor concepts, the material attractiveness results may nonetheless be valuable for other reactor systems using MA fuel and targets that require separated Np or Am. The materials to be evaluated are expected to be available in the reprocessing facility either following reprocessing of LWR SNF intended to be used to fabricate (Np,Am)N fuel or after reprocessing of the irradiated (Np, Am)N fuel.
For the reprocessed LWR fuel, we studied
separated metallic 237Np
separated metallic Am (241Am +243Am)
Am + Np alloy.Footnotea
For the reprocessed (Np, Am)N, we studied
separated metallic U
separated metallic Np
separated metallic Cm
separated metallic Am
separated metallic Pu
Pu + Np alloy.a
For the materials in the reprocessing facility, the total mass of each element (or in the separated streams of alloy form) in the lead-cooled fast reactor core was considered before and after irradiation.
Within the scope of this work, we do not evaluate what reprocessing techniques may be used in the reprocessing facility. Readers can refer to Refs. [Citation15,Citation16] for comprehensive information on available options. We also do not consider aspects related to the availability of nuclides intended for the proposed fuel fabrication or safety aspects related to handling it.
IV. MONTE CARLO CALCULATIONS
To evaluate the relevant parameters in the attractiveness analysis, a sequence of computational simulations was done according to . In total, three different Monte Carlo simulations were performed: depletion calculations for estimating the composition of the SNF, criticality simulations to estimate the BCM, and radiation transport to estimate radiation dose.
The Serpent2[Citation17] model of the lead-cooled fast reactor core was granted by the author of Ref. [Citation4]. The depletion simulations were performed up to a discharge burnup of 120 MWd/kg in steps of 1 year, followed by decay calculations up to 60 years to model the cooling of the fuel. The Pu content was estimated at CTs of 0 years, 2 years, 5 years, 10 years, 30 years, and 60 years, considering that the material could be reprocessed at a later stage. Along with the composition of the SNF, Serpent2 also provided estimates of total mass, decay heat per unit mass, and spontaneous fission rate for the content of each isotope.
A second Serpent2 simulation was then performed to estimate the BCM as a first indication of how much material (mass) would be required in a NED. The geometrical shape used in the calculations was a sphere, and simulations were performed to estimate the minimum radius that generates (through linear interpolation) a neutron multiplication parameter value, keff ≥ 1. The nuclear data library used in the calculations was JEFF3.1.1,[Citation18] and the material temperature was 300 K.
Third, radiation transport calculations were done with MCNP6.2[Citation19] to calculate the dose emitted by a sphere composed of 0.2 BCM at a 1-m distance in agreement with Ref. [Citation14]. The dose was calculated separately for photons and neutrons via tally F2, using the photon dose function from the International Commission on Radiological Protection document ICRP-21[Citation20] and the effective-dose conversion function for energy deposition from ICRP-60.[Citation21]
V. DENSITY CALCULATIONS
Because the density of the materials under consideration affects the probability of neutron interaction, it is essential to consider what value to use in the calculation of the critical mass. Since the isotopes that compose a specific grouped product can possess different miscibility behaviors, as well as crystallographic lattice preferences, we performed an analysis of the pseudo-binary phase diagrams of the alloys of interest.
The grouped products were categorized into two groups. The first is one where the elements have no solubility, i.e., each actinide forms its own phase. In this case, the density of the compound was estimated based on the average density weighted by the concentration of the actinide, determined by the level rule, as follows:
where Dn = density of actinide n (kg/cm3) and mn = mass of actinide n (kg).
The second group is comprised of elements that are miscible, i.e., the same lattice is shared by different elements. The calculation of the lattice parameters of the crystal structure of the composite was necessary to achieve the expected density of the compound. According to solid-state physics, the average volume per atom in a solid is related to the Wigner-Seitz radius rs, which can be expressed in terms of the molar mass M, the number of atoms in a mole N), the density ρ, and Avogadro’s number (NA),
and it can be related to the lattice parameter a of a certain crystal structure by the equality of its volumes W (Wigner-Seitz volume) and V (crystal structure volume): W(rs) = V(a). For faced-centered cubic and body-centered cubic (bcc) structures, the volume is the cubic volume a3, and the relation between the density and the lattice parameter can be expressed as
To calculate the lattice parameter a of a compound αβ with a bcc crystal structure (a = 4 r/), Vegard’s Law can be used to find an approximation of the atomic radius of the compound,
where the atomic radius of the compound αβ is represented by rαβ and the radii of the elements α and β by rα and rβ, respectively, and is the molar fraction of element α.
VI. RESULTS
The results of this work are presented in the following four subsections. The first contains simulation results from the depletion calculations, the second the density calculation results, the third includes results from the criticality and dose calculations, and the fourth contains the results of the FOM1 and FOM2 calculations and evaluations.
VI.A. Depletion Calculation
As described in the section about Monte Carlo calculations, the depletion calculation with the Serpent2 code provided the total mass (in kg), decay heat (in W), and spontaneous fission neutron rate (in n/s) of each selected nuclide. The decay heat and the spontaneous neutron fission rate were divided by the total mass to acquire the parameters that fed the FOM EquationEqs. (1)(1)
(1) and Equation(2)
(2)
(2) . The results for these two parameters are presented in .
TABLE I Parameters and FOM Calculated Values Related to Material Attractiveness for Materials in Different Stages of the Nuclear Fuel Cycle
In this section, we furthermore describe how the composition of the fuel changes during irradiation and CT, and more specifically, the buildup of Pu and U and the change in the composition of the MAs. shows how the fuel content changes as a function of time spent in the lead-cooled fast reactor (corresponding to the expected 24-year lifetime of the reactor, assuming a 90% availability). shows the composition of the SNF after up to 60 years of CT.
Fig. 4. Actinide mass as a function of time. The fuel is in storage for the next 60 years after discharge.
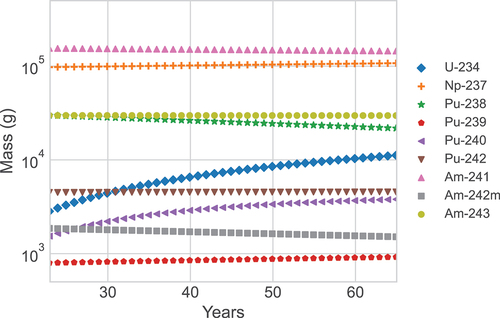
As can be seen in , fresh fuel consists only of 237Np, 241Am, and 243Am, while U, Pu, and Am isotopes build up during irradiation. More specifically, 238Pu is predominately created by neutron captures on 237Np and subsequent beta decays, although some alpha decay of 243Am also contributes. Additional neutron captures in 238Pu cause the heavier Pu isotopes to build up. One can also see that 243Am is continuously created during irradiation and does not present any considerable change in its mass.
After 8000 days of irradiation in the lead-cooled fast reactor, the four most abundant radionuclides in the SNF are 241Am, 237Np, 243Am, and 238Pu, where the first three were present already in the fresh fuel. As well, 234U also builds up, as a consequence of alpha decays in 238Pu. Concerning more detailed information about the SNF at discharge, the total masses of the nuclides of interest for this work are U = 2.6 kg, Np = 100.0 kg, Pu = 36.4 kg, Am = 186.8 kg, and Cm = 3.7 kg. The isotopic composition of each nuclide at discharge is presented in .
During cooling of the SNF, the major change in the SNF isotopic composition is the increase of 234U from 2.6 kg at CT = 0 to 10.0 kg at CT = 60 years, due to alpha decays of 238Pu (see ).
VI.B. Density Results
According to the phase diagram of an alloy consisting of the actinides Am and Np,[Citation22] it is clear that the two isotopes do not mix in the alloy. Thus, EquationEq. (3)(3)
(3) was used for the density calculation of the material Am + Np in the fresh nuclear fuel.
For the material Pu + Np, the molar fractions of Pu and Np in the spent fuel were calculated ( = 0.27 and
= 0.73). According to the phase diagram of the Pu + Np alloy calculated by Kurata,[Citation23] Pu is highly miscible with Np. Thus, EquationEq. (5)
(5)
(5) was used. The values for the atomic radius of Np and Pu were extracted from Ref. [Citation24].
The results related to the density calculation for the compounds Am + Np and Pu + Np are presented in .
VI.C. Criticality and Dose Calculations
As described in the Monte Carlo calculation section, the direct result from the criticality calculations in Serpent2 was the radius of the sphere corresponding to 1 BCM of the material studied. We calculated the mass of material using the radius R information and the densities ρ presented in , mass = 3πR3/4ρ. The calculation dose rate results for neutrons and photons were summed up and are presented in rad/h. The BCM results along with the dose rate calculation results are presented in .
VI.D. FOM Calculations and Evaluation
After having obtained values for the BCM, decay heat, dose rate, and spontaneous fission rate from the Monte Carlo simulations, the estimates were used to calculate the FOMs presented in . The upper part of the table summarizes the properties of materials intended for the fabrication of fuel for the fast lead-cooled reactor (237Np and Am, i.e., 241Am + 243Am, as well as the Am + Np alloy). The lower part of the table summarizes the results of materials built up during irradiation in the lead-cooled fast reactor and foreseen to be separated in the reprocessing facility (U, Np, Am, Cm, Pu + Np, and Pu at discharge and after five selected CTs).
VI.D.1. FOM Uncertainty Results
We observed that an uncertainty of 3% in each of the input parameters (BCM, decay heat, dose rate, and spontaneous fission rate) propagated as 0.015% on average in the FOM results. The larger uncertainties were found for the materials containing Pu (0.018%), while the small uncertainty was for the U material (0.012%). The majority of the materials would not have their classification changed in the limits of the FOM’s calculated uncertainties. The only material that would have its classification changed, if the maximum limit of its uncertainty was applied to the FOM numerical result, would be Am + Np. This material mixture had a FOM very near the classification border between unattractive (FOM < 1) and attractive (FOM = 1), with values of 0.99 and 0.98 for FOM1 and FOM2, respectively. These results show the robustness of the FOM model and gave us confidence in the conclusions we drew about the classification of the materials studied.
VI.D.2. Fresh Lead-Cooled Fast Reactor Fuel Material
The FOM evaluations of 237Np yielded values above 2, indicating this to be a preferred material for use in a NED. The explanation for this is mainly that the decay heat and dose rate are relatively low. Since 237Np also has a very low spontaneous fission rate (the branching ratio for spontaneous fission rate is <10−12),[Citation24] this results in FOM1 and FOM2 being identical. Furthermore, the BCM is lower than that of Am and about six times larger than that of 239Pu. Given the large mass of 237Np in the core, the required mass for one or two (1.8×) NEDs would be available.
The other MA in the fresh fuel is Am in a composition including both fissile isotopes 241Am and 243Am. Although the total mass of Am in the core corresponds to a few BCMs (3.5×), the generated decay heat is high (around 100 W/kg). The major contributor to the high decay heat rate is the 241Am isotope, around 141 W/kg.[Citation14] Furthermore, the dose rate is four orders of magnitude larger than for 237Np. These properties result in FOMs below 1.
The two FOMs for Am are almost the same, since both 241Am and 243Am have very low spontaneous fission rates. With respect to the Am + Np alloy product intended for fresh fuel fabrication, the properties of Am result in the FOM values being similar to, but slightly higher than, those of Am alone. In fact, the two FOM values for Am + Np are very similar and just below 1, meaning that the materials are on the border of being considered attractive for use in a NED.
VI.D.3. Irradiated Lead-Cooled Fast Reactor Fuel Materials
Of particular interest in this work is the FOM evaluations of U, Np, Am, Cm, and Pu + Np after irradiation in the fast reactor, as well as of separated Pu at different CTs.
The FOM evaluation shows that separated U results in values of FOM1 and FOM2 at the high end of the interval of attractive materials. However, the U quantity produced at discharge burnup (120 MWd/kg) is around 2.8 kg in the full core. Considering that the BCM for U with this isotopic composition is 104.5 kg, more than 35 cores would be required (assuming no material losses) to reach a BCM and have a self-sustainable fission reaction. Furthermore, FOM1 and FOM2 for U appear to be the same because of the small spontaneous fission rate. This is due mainly to the majority of U being 234U, which has a branching ratio for spontaneous fission on the order of 10−9.
The FOM evaluations for Np and Am are similar to the results shown for the fresh fuel material, although there is some variation in BCM, decay heat, dose rate, and particularly, the spontaneous fission rate (for Am). To conclude, Np remains a preferred material for use in a NED after irradiation, while Am is considered an unattractive material.
Curium is the only material that yields FOM values on the negative side. The reason for this is the very large decay heat generated in the material in combination with an extremely high spontaneous fission rate. The dose rate is also the highest among the evaluated materials, and the BCM is just about three times as large as that of 239Pu. Thus, Cm provides significant barriers for use in a NED.
The FOM calculations of Pu + Np result in FOM1 having a value of 0.89, while the value corresponding to FOM2 is 0.11. These results are reduced compared to the FOM values of Np and Pu alone. Compared with separated Np, one can note that the grouped product Pu + Np has a higher decay heat (four orders of magnitude higher compared to Np) and an increased spontaneous fission rate for FOM2. Compared with separated Pu, one can observe that although Pu + Np has a decay heat, dose rate, and spontaneous fission rate lower than the ones for separated Pu, it still has lower FOM values.
This divergency is due to the different weights that each parameter has in the FOM equation calculation. The BCM of Pu is very small compared to the BCM of Pu + Np, and it is a parameter with a higher impact on the calculation since it influences all the other terms. Furthermore, it seems that keeping Np together with other actinides, such as Pu and Am, is a way to reduce the attractivity of Np-containing materials.
An analysis of separated Pu (alone) reveals FOM values at discharge of 0.94 (for FOM1) and around 0.30 (for FOM2). The material is clearly more attractive for technically advanced proliferators. With increasing CTs, the FOM values change only moderately as the fraction of 238Pu decreases from around 80% at discharge to about 68% after 60 years of CT. After 60 years of CT, FOM1 increases to 0.95, while FOM2 is reduced to 0.29. The reason for FOM1 and FOM2 being different is due to the high spontaneous fission rate from 238Pu.
VII. NUCLEAR SAFEGUARDS AND PHYSICAL PROTECTION CONSIDERATIONS
In this section, we present a discussion on how the results on attractiveness defined by FOM1 of the studied material relates to the IAEA requirements associated with nuclear safeguards[Citation9,Citation25] and the recommendations from the IAEA on physical protections.[Citation26]
VII.A. Nuclear Safeguards Implications
A general objective of international safeguards is to deter and detect any diversion of declared nuclear material.[Citation9] Of primary concern in the safeguards community are significant quantities (SQs) and timeliness requirements because these properties determine the frequencies of inspections as well as the detection goals for nuclear material. Concerning the material irradiation status and its suitability for conversion into components of a NED, Ref. [Citation9] presents two distinct categories of nuclear material: direct-use nuclear material and indirect-use nuclear material.
Direct-use material is defined in the reference as “… nuclear material that can be used for the manufacture of nuclear explosive devices without transmutation or further enrichment.”[Citation9] This category includes HEU, 233U, Pu containing less than 80% 238Pu, and mixtures of these materials, such as mixed oxides (MOXs) and Pu in SNF.
Indirect-use material is U with enrichment levels below 20% (including low-enriched uranium, natural uranium, and depleted uranium) and thorium. The IAEA does not define Np and Am as source material or special fissionable material but as alternative material. Nevertheless, separated Np and Am within relevant states can be monitored by the IAEA under the Np and Am monitoring scheme.[Citation9] In this section, we look into international safeguards requirements applied to the materials used as fresh fuel for the lead-cooled fast reactor and materials built up during the reactor operation in the irradiated fuel.
VII.A.1. Irradiated Unseparated Fuel Material from the Lead-Cooled Fast Reactor
Taking into consideration the quantities of produced 235U, 237Np, and Pu, the SNF generated by the proposed lead-cooled fast reactor would be considered indirect-use material under safeguards categorization[Citation9] and the material conversion timeliness used to define inspection goals would be between 1 to 3 months.
VII.A.2. Fresh and Irradiated Separated Fuel Material from the Fast Lead-Cooled Reactor
According to Ref. [Citation9], the separated U would be considered as indirect-use material because of its isotopic composition (235U < 20%), but it would still be under international safeguards. Although this finding is consistent with the FOM calculations indicating U to be an attractive material for use in a NED, the produced quantity of U in the entire core is very small (around 2.8 kg at discharge and around 10 kg after CT = 60 years) compared to the defined SQ of 235U in Ref. [Citation9] of 75 kg.
The Pu produced during irradiation is evaluated to be on the border of being attractive for use in a NED according to FOM1 irrespective of CT. As already mentioned, the Pu composition, however, does change with CT, and at CT = 5 years, the fraction of 238Pu drops below 80%. According to existing safeguards classifications, all Pu except that with a 238Pu content above 80% is under nuclear safeguards (under paragraph 36 of INFCIRC/153).[Citation27] This means that the produced Pu cooled for less than 5 years would not need to be placed under safeguards. However, with longer CTs, the Pu would be considered as direct-use nuclear material (238Pu < 80%) according to Ref. [Citation9], and it would need to be subject to nuclear safeguards.
The assessment done by Ref. [Citation9] to establish inspection regimes, quantities, and timeliness detection goals presents Pu with a SQ of 8 kg, and the estimated material conversion time for finished Pu metal components would be in the order of (1 to 3) months if the conversion takes part from irradiated nuclear fuel and in the order of (7 to 10) days if the conversion takes part from Pu metal.
Separated Cm is not a material subject to nuclear safeguards, which is consistent with the attractiveness evaluation in this study, which shows negative FOM values.
As mentioned earlier, separated Np and Am are not defined under the IAEA statute[Citation25] as source material or special fissionable material and are only monitored by the IAEA under voluntary arrangements with states. The voluntary monitoring scheme of Am and Np was introduced by the IAEA via flowsheet verification of inventories, exports, and separation of the material.[Citation9] By 2014, the IAEA had received information regarding the monitoring of separated Np and Am from the European Commission and eight states: Japan, United Kingdom, United States, Pakistan, Norway, Republic of Korea, France, and the Czech Republic.[Citation28]
Although separated Am FOMs classify the material as unattractive as fresh and spent fuel, Np is the material with the highest FOMs (2.08 as fresh fuel and 2.09 as spent fuel) in this study. Despite the existence of a monitoring scheme for Am and Np, no details for SQ or timeless detection are publicly available. Since separated Np is classified as a preferred material for use in a NED, we suggest defining SQ values and timeless detection values for Np. Albright[Citation8] argues that 237Np and 235U have similar critical masses, and that the SQ quantity and timeliness detection goals defined for 235U could be applied also to 237Np if it were to be placed under safeguards. That would mean that the SQ for 237Np would be 25 kg and that the estimated material conversion time for 237Np in metal, oxide, and contained in irradiated fuel would be 7 to 10 days, 1 to 3 weeks, and 1 to 3 months, respectively, according to Ref. [Citation9]. It can be noted that the U.S. Department of Energy (DOE)[Citation29] also places separated 237Np in the same list of safeguarded materials as 235U.
Since the grouped product Am + Np is classified on the border of being attractive material, we would suggest that the same safeguards considerations assumed for separated Np would apply to the Am + Np product.
VII.B. Physical Protection Implications
The IAEA describes the attractiveness of nuclear material available for potential theft in the INFCIRC/225[Citation26] guidelines on the physical protection of nuclear material. Three categories are introduced in Ref. [Citation26] based on the irradiation status of the material, its quantity, and its suitability for conversion into components of a NED. The categorization is the basis for a graded approach for protection against the unauthorized removal of nuclear material, and each category is related to a certain level of required physical protection and different measures of control and accountability.
Category I materials require a high physical protection level, while Category II and Category III materials require a lower level of physical protection. According to Ref. [Citation26], Category I nuclear material should be used and stored in a vital (inner) area, providing an additional layer for detection, access control, and delay against unauthorized removal. Additional recommendations are also presented for the transport of Category I nuclear material, such as the presence of armed guards.
Although in a lower category, the recommendations from Ref. [Citation26] for Category III nuclear materials include that they should be stored and handled inside limited access areas, which must include prudent and necessary security measures such as keys and a computerized access list. The listed materials under the proposed categorization are irradiated fuel, Pu, 233U, and 234U. Even though the DOE[Citation29], in a document from 2019, defines separated 237Np and Am (241Am and 243Am) as other accountable nuclear materials (with reportable quantities of grams), the document does not present an attractiveness level for these materials.
VII.B.1. Irradiated Unseparated Fuel from the Lead-Cooled Fast Reactor
The categorization of SNF presented in Ref. [Citation26] is based on international transport considerations, placing irradiated fuel with less than 10% fissile content in Category II. Irradiated fuel with more than 10% fissile content is placed in Category I. The results of the analyses in this work showed that the irradiated fuel from the lead-cooled fast reactor contains around 20% 237Np, 40% 241Am, and 7% 243Am. The 237Np, 241Am, and 243Am can sustain a neutron chain reaction on fast neutrons, and together they correspond to a total content of about 67%. Considering this property alone, the SNF from the lead-cooled fast reactor could be placed in physical protection Category I.
VII.B.2. Fresh and Irradiated Separated Fuel Material from the Lead-Cooled Fast Reactor
Considered materials in this category are the already discussed materials of interest in the spent lead-cooled fast reactor fuel, i.e., U, Np, Am, Cm, Pu, and Pu + Np.
The amount of U produced in the entire reactor core at discharge is about 2.8 kg, and the enrichment level (of 235U) is around 1.7%.
The low enrichment level and the small quantity produced in the core place the U outside the physical protection Categories I, II, and III described in Ref. [Citation26], but still in need of placement in a limited-access area with safety measures, especially if it is highly radiotoxic. However, the FOM evaluation performed in this work classifies U to be attractive for use in a NED.
The FOM calculations evaluated separated Np as attractive material, but this material is not included in Ref. [Citation26]. Nevertheless, as discussed in the previous subsection, 237Np is comparable to 235U not only in terms of critical mass but also in terms of decay heat, radiation dose rate, and spontaneous fission rate. Based on the results of this work, we suggest that the same physical protection measures recommended for 235U in Ref. [Citation26] should be applied to separated 237Np, and potentially also to any separated Np grouped products with a major contribution of 237Np.
Neither materials Am or Cm are mentioned under the IAEA recommendation document.[Citation26] And since the FOM values calculated classify both separated materials as unattractive, with the major proliferation barrier being decay heat, we do not foresee the necessity of a different approach to physical protection measures related to these materials.
For the product Am + Np, despite the majority of the material being composed of Am (67%), we here propose the application of the same physical protection measures previously suggested for Np materials since its attractivity classification according to the FOM calculations places the material close to the limit of attractive material.
The Pu produced by the lead-cooled fast reactor amounts to around 36 kg at discharge, and its composition changes with CT, mainly as 238Pu decays (half-life of 87.7 years). The FOM1 calculations evaluated Pu as unattractive but on the border of attractive material for all CT cases considered. In the evaluations, the fraction of 238Pu in Pu is around 68% to 81%. According to Ref. [Citation26], Pu material with more than 80% of 238Pu is outside the available physical protection categories, meaning that it does not require special physical protection measures. However, it should still be stored and handled in a limited-access area with safety measures. Nevertheless, as the fraction of 238Pu becomes lower than 80% (after 5 to 10 years CT), Ref. [Citation26] would place the Pu produced by the lead-cooled reactor under Category I as the quantity is larger than 2 kg.
The physical protection classification for the product Pu + Np should be based on the Pu content, following Ref. [Citation26]. However, since the material composition consists in its majority of Np (73%) and its classification according to the FOM calculations places it on the borderline of attractive material, we propose that the physical protection recommendations should follow the previously proposed recommendations for Np material instead.
VIII. CONCLUSION
In this work, we evaluated the attractiveness of materials used in a nuclear energy system containing a lead-cooled fast reactor fueled with (Am,Np)N fuel, designed to transmute MAs.[Citation4] The fresh fuel was foreseen to originate from reprocessing of SNF from LWRs. The material attractiveness of the fresh and irradiated nuclear fuel was evaluated using two different FOMs, considering two different types of proliferators. Monte Carlo simulations were performed to obtain the estimated BCM, decay heat, dose rate, and spontaneous fission rate, and these estimations were used as input to the FOM calculations. We then discussed international safeguards and physical protection issues related to the materials involved in the proposed energy system (U, Np, Pu, Am, and Cm) using the material attractivity results from FOM1.
Despite being classified as attractive by the FOMs, the U material produced by the lead-cooled fast reactor is in a quantity way below the SQ established by Ref. [Citation9] for nuclear safeguards verification applications and also outside the categories established by Ref. [Citation26] for physical protection recommendations.
The results revealed that Np, both in fresh and irradiated nuclear fuel, was classified as a preferred and attractive material, respectively, for use in a NED by the FOMs. While the IAEA could refer to the Np and Am monitoring scheme to apply international safeguards to separated Np in relevant states, the extent of the current physical protection measures warranted for this material does not align with its attractivity level, nor does it correspond to the quantities outlined by Ref. [Citation4]. If states engage in research and demonstrate advanced fuel reprocessing technologies, facilitating the large-scale separation of Np (connected to the quantities proposed by Ref. [Citation4]), then there may be a requisite for the formal recommendation of enhanced physical protection measures for 237Np. In this study, we recommend that the same physical protection measures applied to 235U could be considered for 237Np based on similarities found between the physical properties of both nuclides.
Although Ref. [Citation4] declares that the Pu isotopic composition in the spent fuel would classify it as proliferation resistant, the FOM1 calculations showed Pu material was on the borderline of being attractive for a technically advanced proliferating state or a subnational group. We also highlight that the isotopic composition of the Pu material produced in the lead-cooled fast reactor changes depending on CT. This in turn changes its classification under international nuclear safeguards requirements and categorization under physical protection recommendations with time. According to the results here presented, we do not find arguments to justify a lower level of international safeguards or physical protection measures for the spent fuel generated by the lead-cooled fast reactor.
The materials Am, and particularly Cm, were classified as unattractive for use in a NED, primarily because of the high decay heat content and the high spontaneous fission rate. We do not find pieces of evidence to recommend a change in the physical protection measures or international safeguards applied to Am and Cm with the isotopic composition strictly related to the energy system under research.
The grouped products Am + Np and Pu + Np were classified on the borderline of being attractive to a subnational group or a technically advanced proliferating state. Although the attractivity of products from the co-extraction of Np with Am or Pu has been shown to be reduced compared to pure Np, we would not classify these products as unattractive for use in a NED without further chemical separation.
One important note is that the FOM evaluations did not consider the material availability, and as pointed out by Ref. [Citation14], the quantity of material is as relevant as its properties. We stress here that a holistic physical protection and nuclear safeguards approach needs to consider both material properties and material quantities, as there is a finite amount of resources to be allocated to either area.
Acknowledgments
The authors acknowledge Janne Wallenius from the Royal Institute of Technology for sharing the code of his lead-cooled fast reactor core model and for fruitful discussions.
Disclosure Statement
No potential conflict of interest was reported by the authors.
Additional information
Funding
Notes
a Since we expected a high attractivity of Np, we evaluated the co-extraction of Np with Am or Pu to check for changes in the attractivity of the rendered grouped products. These grouped product materials were evaluated in the form of alloy.
References
- “Programme for Encapsulation, Deep Geological Disposal and Research,” RD&D—Programme—Svensk Kärnbränslehantering AB (1995).
- “Implications of Partitioning and Transmutation in Radioactive Waste Management,” Vol. 435 of Technical Reports Series, International Atomic Energy Agency (2004).
- “Status of Minor Actinide Fuel Development,” NF-T-4.6, IAEA Nuclear Energy Series, International Atomic Energy Agency (2009).
- J. WALLENIUS, “Maximum Efficiency Nuclear Waste Transmutation,” Ann. Nucl. Energy, 125, 74 (2019); http://dx.doi.org/10.1016/j.anucene.2018.10.034.
- “Technical Features to Enhance Proliferation Resistance of Nuclear Energy Systems,” NF- T-4.5, IAEA Nuclear Energy Series, International Atomic Energy Agency (2010).
- J. EJENSTAM et al., “Oxidation Studies of Fe10CrAl-RE Alloys Exposed to Pb at 550°C for 10000 h,” J. Nucl. Mater., 443, 161 (2013); http://dx.doi.org/10.1016/j.jnucmat.2013.07.023.
- J. EJESTAM and P. SZAKALOS, “Long Term Corrosion Resistance of Alumina Forming Austenitic Stainless Steels in Liquid Lead,” J. Nucl. Mater., 461, 164 (2015); http://dx.doi.org/10.1016/j.jnucmat.2015.03.011.
- D. ALBRIGHT and K. KRAMER, “Neptunium 237 and Americium: World Inventories and Proliferation Concerns,” Institute for Science and International Security (2005).
- IAEA Safeguards Glossary, International Atomic Energy Agency (2002).
- B. PELLAUD, “Proliferation Aspects of Plutonium Recycling,” J. Nucl. Mater. Manage., 3, 7–8, 1067 (2002).
- H. SAGARA et al., “Denaturing of Plutonium by Transmutation of Minor-Actinides for Enhancement of Proliferation Resistance,” J. Nucl. Sci. Technol., 42, 161 (2005); http://dx.doi.org/10.1080/18811248.2005.9726376.
- G. KESSLER, “Plutonium Denaturing by Pu-238,” Nucl. Sci. Eng., 155, 53 (2007); http://dx.doi.org/10.13182/NSE07-A2644.
- M. SAITO et al., “Development of Methodology for Plutonium Categorization (II)—Improvement of Evaluation Function ‘Attractiveness’,” Am. Nucl. Soc. Trans., 98, 145 (2008).
- C. BATHKE et al., “The Attractiveness of Materials in Advanced Nuclear Fuel Cycles for Various Proliferation and Theft Scenarios,” Nucl. Technol., 19, 51 (2012).
- “Assessment of Partitioning Processes for Transmutation of Actinides,” IAEA TECDOC 1648, International Atomic Energy Agency (2010).
- P. BARON et al., “A Review of Separation Processes Proposed for Advanced Fuel Cycles Based on Technology Readiness Level Assessments,” INL/JOU-18-44641-Rev.-0, Idaho National Laboratory (2019).
- J. LEPPÄNEN et al., “The Serpent Monte Carlo Code: Status, Development and Applications in 2013,” Ann. Nucl. Energy, 82, 142 (2015); http://dx.doi.org/10.1016/j.anucene.2014.08.024.
- “The JEFF-3.1.1 Nuclear Data Library,” OECD Publishing (2009).
- “MCNP® User’s Manual Code Version 6.2,” LA-UR-17-29981, Los Alamos National Laboratory (2002).
- “Data for Protection Against Ionizing Radiation from External Sources: Supplement to ICRP Publication 15,” ICRP Publication 21, International Commission on Radiological Protection, Pergamon Press (1973).
- “Recommendations of the International Commission on Radiological Protection,” ICRP Publication 60, International Commission on Radiological Protection, Pergamon Press (1991).
- N. GREENWOOD and A. EARNSHAW, Chemistry of the Elements, Butterworth- Heinemann (1997).
- M. KURATA, Phase Diagrams of Actinide Alloys, Elsevier Ltd (2012).
- A. L. NICHOLS, D. L. ALDAMA, and M. VERPELLI, Handbook of Nuclear Data for Safeguards, International Atomic Energy Agency (2007).
- Statute of the International Atomic Energy Agency, International Atomic Energy Agency (1956).
- “Nuclear Security Recommendations on Physical Protection of Nuclear Material and Nuclear Facilities,” INFCIRC/225/Rev.5, NSS 13, International Atomic Energy Agency (2011).
- “The Structure and Content of Agreements Between the Agency and States Required in Connection with the Treaty on the Non-Proliferation of Nuclear Weapons,” INFCIRC/153/Corrected, International Atomic Energy Agency (1972).
- “The Safeguards Implementation Report for 2014,” GOV/2015/30, Board of Governors, International Atomic Energy Agency (2015).
- “Nuclear Material Control and Accountability,” DOE -STD-1194, U.S. Department of Energy (2019).