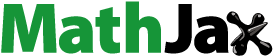
Abstract
In the present study, we investigated the potential immunomodulatory effects of heat-killed (hLR) and live Limosilactobacillus reuteri PSC102 (LR; formerly Lactobacillus reuteri PSC102) in RAW264.7 macrophage cells and Sprague–Dawley rats. RAW264.7 murine macrophage cells were stimulated with hLR and LR for 24 h. Cyclophosphamide (CTX)-induced immunosuppressed Sprague–Dawley rats were orally administered with three doses of hLR (L-Low, M-Medium, and H-High) and LR for 3 weeks. The phagocytic capacity, production of nitric oxide (NO), and expression of cytokines in RAW264.7 cells were measured, and the different parameters of immunity in rats were determined. hLR and LR treatments promoted phagocytic activity and induced the production of NO and the expression of iNOS, TNF-α, IL-1β, IL-6, and Cox-2 in macrophage cells. In the in vivo experiment, hLR and LR treatments significantly increased the immune organ indices, alleviated the spleen injury, and ameliorated the number of white blood cells, granulocytes, lymphocytes, and mid-range absolute counts in immunosuppressive rats. hLR and LR increased neutrophil migration and phagocytosis, splenocyte proliferation, and T lymphocyte subsets (CD4+, CD8+, CD45RA+, and CD28+). The levels of immune factors (IL-2, IL-4, IL-6, IL-10, IL-12A, TNF-α, and IFN-γ) in the hLR and LR groups were upregulated compared with those in the CTX-treatment group. hLR and LR treatments could also modulate the gut microbiota composition, thereby increasing the relative abundance of Bacteroidetes and Firmicutes but decreasing the level of Proteobacteria. hLR and LR protected against CTX-induced adverse reactions by modulating the immune response and gut microbiota composition. Therefore, they could be used as potential immunomodulatory agents.
1. Introduction
The immune system, comprising the spleen, bone marrow, and thymus, is a rigorous defense mechanism against microbes and foreign antigens (Yatim and Lakkis Citation2015). This highly sophisticated system involves various immune cells, macrophages, and lymphocytes. Lymphocytes secrete cytokines and antibodies that are associated with cell-mediated and humoral immunity. Any disorder in the immune system can result in inflammatory diseases, autoimmune diseases, obesity, diabetes, and even cancer (Rohm et al. Citation2022). The immune functions of the body are essential for the inhibition of and recovery from these immune-mediated disorders. Therefore, developing a new, effective, and safer immunomodulating agent may be an efficient and effective strategy for the treatment of immunosuppressive disorders.
The microbiota is a diverse and intricate ecosystem comprising trillions of bacteria, including thousands of species in mammals; as such, it is gradually becoming recognized as an integral part of the host immune regulation (Colella et al. Citation2023). Intestinal bacteria play an important role in maintaining and developing host immunity by triggering the immune system and supporting the barrier functions of the epithelial layer (Håkansson et al. Citation2015). However, when microbial dysbiosis occurs, it triggers the change in the intestinal epithelial mucosa and stimulates inflammation by external stimuli (Weiss and Hennet Citation2017).
Researchers have been developing effective ways of using probiotics to treat or prevent immunosuppressive diseases and gut microbiota dysbiosis for years. Several bioactive components of probiotics, such as metabolites, organic acids, short-chain fatty acids, peptides, enzymes, proteins, and exopolysaccharides (EPSs), contribute to comprehensive health benefits, including maintenance of intestinal microbial balance and immunomodulatory effects (Indira et al. Citation2019; Bernardeau and Cretenet Citation2019). Moreover, certain active cellular constituents of probiotics, such as peptidoglycans, teichoic acid, lipoteichoic acid, and lipopolysaccharides, have a role in regulating the immune system by promoting both innate and adaptive immune responses (Yeşilyurt et al. Citation2021). Nevertheless, there is ongoing debate regarding the safety of using live probiotics. Systemic infections are brought on by translocation in pediatrics and susceptible individuals (Kothari et al. Citation2019). Furthermore, the administration of probiotics may increase the likelihood of gaining antibiotic-resistant genes and disrupt the gut microbiota composition in newborns (Imperial and Ibana Citation2016). The consumption of live probiotics in specific risk factors, such as diabetes, mitral regurgitation, and short-gut syndrome, has the potential to result in various sepsis, endocarditis, and bacteremia (Boumis et al. Citation2018; Castro-González et al. Citation2019; Katkowska et al. Citation2021). In order to overcome the potential hazards, probiotics are typically used as non-antibiotic-resistant or non-infectious agents, mainly in heat-killed forms. Investigations have suggested that heat-killed probiotics can modulate the immune response. Heat-inactivated Lactobacillus rhamnosus ATCC7469 induces the synthesis of tumor necrosis factor (TNF)-α, interleukin (IL)-6, IL-1β, IL-4, IL-10, and IL-12 in RAW264.7 cells (Jorjão et al. Citation2015). Lee et al. (Citation2019) showed that heat-killed L. plantarum LM1004 can elicit immunomodulatory effects by modulating the expression of serum TNF-α, inducible nitric oxide synthase (iNOS), and toll-like receptor (TLR)-4. It was investigated that heat-inactivated L. gasseri can boost immune activity by enhancing CD8+ lymphocyte levels (Miyazawa et al. Citation2015). Moreover, heat-killed L. plantarum L-137 stimulates immune functions by modulating intestinal microbiota composition (Nakai et al. Citation2021).
L. reuteri is an important probiotic that has been shown to possess various health-beneficial activities, including immunomodulatory effects (Jørgensen et al. Citation2017; De Marco et al. Citation2018; M.S. Ali et al. Citation2022). Additionally, it has the capability to modulate the composition of gut microbiota (Li et al. Citation2020). Since L. reuteri has been described to show beneficial activity in numerous models, we postulate that L. reuteri PSC102 possesses immunomodulatory activities. Therefore, we investigated the immunomodulatory effects of heat-killed L. reuteri PSC102 (hLR) and live LR in cyclophosphamide (CTX)-induced immunosuppressed Sprague–Dawley rats. Cyclophosphamide (CTX) is widely used as a myelotoxic alkylating agent in creating an immunosuppressive model to examine immunomodulatory activities (Meng et al. Citation2018; Noh et al. Citation2019; Zhang et al. Citation2020).
2. Materials and methods
2.1. Preparation of sample
L. reuteri PSC102 (preserved at the Laboratory of Veterinary Pharmacokinetics and Pharmacodynamics, College of Veterinary Medicine, Kyungpook National University) was cultured in de Man Rogosa Sharpe (MRS) media at 37 °C for 24 h. Biomasses were obtained by centrifugation (6000 × g for 10 min) and washed with phosphate-buffered saline. Whole lysates were dried in a vacuum freeze dryer (Operon Co., Ltd., Gyeonggi, South Korea) and kept at −70 °C for the experiment. The colony forming units (CFU) per gram of the dried sample was measured by incubating the sample on an MRS agar plate. The sample was heat-inactivated for 15 min at 80 °C.
Four different temperatures (37 °C, 60 °C, 80 °C, and 100 °C) and time points (0, 15, 30, 45, and 60 min) were set to examine and determine the heat inactivation of L. reuteri PSC102. After 24 h of culturing at 37 °C and subsequent centrifugation (6000 × g, 10 min), the cell biomasses were washed thrice with PBS. Approximately 1 × 109 CFU/mL of pellets was suspended in PBS and heated in a water bath at different time points and temperatures. After being heated, the bacteria were quickly chilled to 4 °C. A small portion of the heated bacterial suspension was serially diluted and incubated by spreading on MRS agar plates at 37 °C for 24 h. The viable colonies of each sample were obtained and expressed in CFU per milliliter.
2.2. Morphological analysis via scanning electron microscopy (SEM)
The morphological features of hLR and LR were analyzed through SEM as described previously (Ali et al. Citation2023). hLR and control bacterial cells were fixed in 2.5% glutaraldehyde in PBS (pH 7.0) at 4 °C for 2 h and washed thrice with PBS. The cells were washed and dehydrated using a series of ethanol concentrations (30%, 50%, 70%, 80%, 90%, and 100%). The samples were frozen overnight by storing at −70 °C and freeze-dried for 24 h. Then, the samples were mounted on SEM tubes, successively sputter-coated with gold–palladium, and analyzed under a SEM (model S-4300; Hitachi, Japan) at 5.0 kV and magnification of ×35000.
2.3. RAW264.7 cell culture and in vitro study
RAW264.7 cells were collected from Korean Cell Line Bank and cultured in Dulbecco’s modified Eagle medium (DMEM, Welgene, Gyeongsangbuk, South Korea) supplemented with 10% fetal bovine serum (FBS) and 1% penicillin and streptomycin (P/S). The cell culture was maintained in an incubator at 37 °C and 5% humidified conditions.
2.3.1. Cell viability assay
For cell viability evaluation, RAW264.7 cells were cultured in a 24-well plate (SPL Life Science Co., South Korea). Then, 1 × 105 cells/well were seeded in a culture plate and incubated at 37 °C in a 5% CO2-supplied incubator for 12 h. After the medium was changed, the cells were treated with hLR and LR and incubated for 24 h. The viability of RAW264.7 cells upon sample treatment was measured using a 3-(4,5-dimethylthiazol-2-yl)-2,5-diphenyl tetrazolium bromide (MTT) assay (Quah et al. Citation2020).
2.3.2. Phagocytic activity
The phagocytic activity of RAW 264.7 cells was assessed using the neutral red uptake method (Zhang et al. Citation2019). Initially, cells were seeded in a 96-well plate at a density of 1 × 105 cells/mL and subsequently incubated overnight at 37 °C in a humidified atmosphere containing 5% CO2. Following the seeding period, cells were subjected to various treatments for a duration of 24 h. Upon completion of the treatment, the supernatant was discarded, and 200 μL of medium containing 20 µL of neutral red was added to each well, followed by a 2-h incubation period. Subsequently, the cells within the 96-well plate were washed with PBS to eliminate any precipitated neutral red. Cell lysis was achieved by the addition of 200 μL of cell lysate (composed of ethanol and 0.01% acetic acid at a 1:1 ratio), and the mixture was maintained at room temperature on a slow shaking plate for 10 min. The optical density at 540 nm was then measured using a microplate reader (BioTek, Winooski, VT, USA).
2.3.3. Production of NO
The level of NO production was assayed using Griess reagent. The cultured RAW264.7 cells (1 × 105 cells/well) were seeded into a 24-well plate for 12 h and treated with hLR and LR for 24 h. The supernatant (100 μL) was collected and mixed with 100 μL of Griess reagent (0.1% N-(1-naphthyl)ethylenediamine dihydrochloride and 1% sulphanilamide in 5% phosphoric acid) in a 96-well plate. The plate was then shaken in an orbital shaker at room temperature for 1 min. Absorbance was read at 540 nm in a multi-mode microplate reader (BioTek, Winooski, VT, USA). NO production was quantified as nitrite (NO2) based on a standard curve established from sodium nitrite (NaNO2; (Ali et al. Citation2022).
2.3.4. Quantitative real-time polymerase chain reaction (qRT-PCR)
RAW264.7 cells were treated with samples for 24 h, and their total RNA was extracted using TRIzol reagent (Life Technologies, CA, USA). Then, 5 µg of RNA from each sample was transcribed into cDNA by using AccuPower® RT premix (Bioneer, Daejeon, South Korea). The relative mRNA expression levels of different cytokines such as iNOS, TNF-α, IL-6, IL-1β, and cyclooxygenase (Cox)-2 were assessed using a cycler CFX96 TouchTM PCR detection system (Bio-rad, Singapore). The following particular primers were utilized in PCR amplification, with β-actin as the internal control: iNOS forward (F): TGTGGCTACCACATTGAAGAA and reverse (R): TCATGATAACGTTTCTGGCTCTT; TNF-α F: CTGTAGCCCACGTCGTAGC and R: GGTTGTCTTTGAGATCCATGC; IL-1β F: TGAGCACCTTCTTTTCCTTCA and R: TTGTCTAATGGGAACGTCACAC; IL-6 F: TAATTCATATCTTCAACCAAGAGG and R: TGGTCCTTAGCCACTCCTTC; Cox-2 F: CACTACATCCTGACCCACTT and R: ATGCTCCTGCTTGAGTATGT; and β-actin F: GTCATCACTATTGGCAACGAG and R: TTGGCATAGAGGTCTTTACGG. For real-time qPCR, the following cycling conditions were used: initial denaturation and enzyme activation at 95 °C for 5 min; 40 cycles of amplification at 95 °C for 10 s; and annealing at 60 °C (iNOS and IL-1β) and 56 °C (TNF-α, IL-6, and Cox-2) for 20 s.
2.4. Animals and in vivo study
The experimental protocols used for this animal study were approved by the Committee on Care and Use of Laboratory Animals of Kyungpook National University (KNU-2021-50). Four-week-old specific pathogen-free Sprague–Dawley rats were purchased from Orient Bio Inc. (Gyeonggi-do, Republic of Korea). They were acclimatized in a laboratory environment for 7 days under the following conditions: room temperature, 22 °C–26 °C; relative humidity, 55%–70%; and 12 h/12 h light and dark cycle. They were supplied with ad libitum filtered water and a standard pellet diet throughout the experiment. They were randomly distributed into eight groups with eight rats each (). Except for the normal control and levamisole groups, all the groups were intraperitoneally (i.p.) injected with cyclophosphamide (80 mg/kg/day) dissolved in sterile isotonic saline for the first 3 consecutive days to induce immunosuppression. Then, they were administered a daily oral dose of 1 mL of levamisole, LhLR, MhLR, HhLR, and LR dissolved in distilled water for 3 weeks through gavage feeding (Figure S1). Physical examination and body weight measurement were performed regularly for dosage adjustment. On day 21, the rats were terminated, and blood was collected and centrifuged (1000 × g, 10 min) to separate serum for enzyme-linked immunosorbent assay (ELISA); their organs were processed accordingly.
Table 1. Description of treatment groups.
2.4.1. Analysis of body weight and immune organ index
The weight of the rats was measured regularly throughout the experiment to adjust the dose. Before they were sacrificed, their body weight was taken, and their immune organs were excised and accurately weighed. The following formula was used to calculate the immune organ index:
(Fei et al. Citation2022).
2.4.2. Spleen histological analysis
Spleen tissues were fixed with 10% of buffered formalin solution. Then, the organs were processed and embedded in paraffin. The samples were cut (4–5 μm thick) using a frozen section machine and stained with hematoxylin and eosin (H&E). The histological characteristics of the tissues were assessed under a microscope.
2.4.3. Complete blood count (CBC) analysis
After final drug administration, blood was collected from the sacrificed rats and placed in a heparinized tube. CBC analysis was performed following previously described procedures (Lee et al. Citation2018). The number of immune blood cells such as WBCs, granulocytes, lymphocytes, and mid-range absolute, which generally comprises basophils, eosinophils, and monocytes, were counted using URIT-300 Vet Plus (URIT Medical Electronic Co., Ltd., Guangxi, China).
2.4.4. Neutrophil isolation and counting
Histopaque® (Sigma-Aldrich, USA) was used to separate neutrophils from the whole blood. Briefly, a 15 mL conical tube was filled with equal parts of whole blood and Histopque-1077; then, the specimen was centrifuged (400 × g, 30 min) at room temperature. After the upper layer was carefully aspirated within 0.5 cm of the opaque interface, the interface was collected, washed with 10 mL of PBS, and centrifuged at 250 × g for 10 min. The cells were counted using a hemocytometer by mixing with trypan blue after they were reconstituted in DMEM.
2.4.5. Neutrophil migration assay
A neutrophil migration assay was conducted using a CytoselectTM 24-well migratory test kit (3 μm, fluorometric format) in accordance with the manufacturer’s instructions (Cell Biolabs, INC. CA, USA). Briefly, after neutrophils were isolated as previously described, 100 mL of 5 × 106 cells/mL in serum-free DMEM was added to the insert, and 500 μL of the media containing 10% FBS was added to the lower well of the migratory plate. The cells were then incubated for 24 h, and the media were aspirated from the inside insert. They were further incubated at 37 °C for 30 min after the insert was transferred to a clean well containing 200 μL of a cell detachment solution. The cells were fully removed from the membrane’s underside by gently tilting the insert into the detachment solution that was then mixed with 400 μL of the media containing migrating cells. The mixture and 60 μL of a 4 × lysis buffer/CyQuant® dye solution (Thermo Fischer Scientific, Waltham, Massachusetts, USA) were poured into a 96-well plate and left to stand at room temperature for 20 min. The mixture (200 μL) was then placed in a 96-well plate to read the fluorescence at 480 nm and expressed as relative fluorescence unit (RFU).
2.4.6. Neutrophil phagocytosis assay
CytoselectTM 96-well phagocytosis assay (zymosan substrate-based; Cell Biolabs, Inc., CA, USA) was performed in accordance with the manufacturer’s instructions to determine the phagocytic activity of neutrophils. Briefly, neutrophils (100 μL of 106 cells/mL) was seeded in a 96-well plate, and 10 μL of zymosan was added to each well. The resulting sample was incubated at 37 °C for 2 h. After centrifugation, the culture medium was removed by aspiration and washed with cold PBS. The fixation solution (100 μL) was supplied to each well, and the wells were left to stand at room temperature for 10 min. Then, the fixation solution was eliminated by centrifugation and washed with PBS. The prepared blocking solution (100 μL) was added to each well and incubated at room temperature for 60 min. After the solution was centrifuged and washed, the prepared permeabilizing solution (100 μL) was added, and the mixture was left to stand at room temperature for 5 min. After the mixture was washed, a detection antibody solution (100 μL) was added to each well. After the solution was washed again with PBS, 50 μL of detection buffer was added to each well and incubated at room temperature for 10 min. A substrate solution (100 μL) was added to each well to initiate the reaction by incubating at 37 °C for 20 min. After 50 μL of stop reagent was added, absorbance was measured at 405 nm by using a microplate reader (BioTek Instruments, Inc., Winooski, USA).
2.4.7. Isolation of PBMCs
Whole blood was collected into anticoagulant-coated tubes and mixed well. An equal volume of whole blood (3 mL) and Histopaque-1077 (Sigma-Aldrich, St. Louis, MO, USA) was mixed in a 15 mL falcon tube to create a density gradient. The upper layer above the opaque interface containing the mononuclear cells was carefully removed after centrifugation at 400 × g and room temperature for 30 min. The opaque layer was collected and transferred into a conical tube. The cells were washed with 10 mL of PBS and then centrifuged at 250 × g for 10 min. After the supernatant was discarded, the cells were resuspended in 1 mL of FACS buffer. Finally, the cells were counted using a hemocytometer.
2.4.8. Preparation of splenocytes and thymocytes
Splenocytes and thymocytes were prepared in accordance with previously described methods (Ahmad et al. Citation2015). Briefly, after the rats were terminated, the spleen and thymus were removed aseptically and kept in Hank’s balanced salt solution (HBSS; Gibco Life Technologies, New York, NY, USA). The organs were sieved through a 70 μm nylon cell strainer (BD Bioscience, CA, USA) by using a 3 mL syringe plunger. Then, the cells were centrifuged at 1800 rpm for 5 min, suspended in red blood cell (RBC) lysing buffer to lyse RBCs, and centrifuged again (1800 rpm, 5 min) to remove the lysed RBCs. The supernatant was discarded and washed thrice with PBS. The cell pellets were suspended in Roswell Park Memorial Institute (RPMI)-1640 media (Sigma-Aldrich, St. Louis, MO, USA), and the number of cells was counted using a hemocytometer via a trypan blue dye exclusion method.
2.4.9. Splenic lymphocyte proliferation assay
A splenocyte proliferation assay was performed using mitogen concanavalin A (Con A) and lipopolysaccharide (LPS). In this procedure, 100 μL of 1 × 106 cells/mL from different groups was seeded in a 96-well plate containing RPMI-1640 media supplemented with FBS (10%) and P/S (1%). The total volume was adjusted to 200 µL by adding mitogen Con A or LPS (5 μg/mL), and the cells were incubated at 37 °C for 72 h. The MTT reagents (20 μL) of 5 mg/mL were mixed in each well and incubated for 4 h. Then, 200 μL of dimethyl sulfoxide was added to each well, and absorbance was read at 570 nm.
2.4.10. Fluorescence-activated cell sorting (FACS) analysis of T lymphocyte subpopulations
The T lymphocyte phenotyping of the thymus, PBMCs, and spleen was performed at a concentration of 1 × 106 cells/mL by harvesting them into a FACS tube. T cells were stained with the following anti-rat antibodies: anti-CD3+ (APC-conjugated), anti-CD4+ (PE-Cy7-conjugated), anti-CD8+ (FITC-conjugated), anti-CD45RA+ (PE-conjugated) and anti-CD28+ (BV711-conjugated). Then, 10 μL of 106 cells/mL was mixed and incubated in a dark environment at 4 °C for 30 min. The cells were centrifuged (300× g, 5 min) with FACS buffer to remove unbound antibodies. For FACS analysis, the cells were suspended in 400 μL of FACS buffer. PBMCs, thymocytes, and splenocytes were analyzed in terms of CD3 T cells, CD4 Th, CD8 CTL, CD45RA, and CD28 regulatory T cells (Treg). Multicolor FACS with FACSDiva version 6.1.3 on BD FACSAriaTM III (BD Biosciences, San Diego, CA, USA) was used to acquire and analyze the data.
2.4.11. Determination of cytokines in the serum
Cytokine concentrations were measured via ELISA in accordance with the manufacturer’s protocol (ELK Biotechnology, Wuhan, China). Briefly, 100 μL of the sample or standard was transferred to each well and incubated at 37 °C for 2 h. The wells were washed thrice with the wash buffer. Then, 100 μL of pre-diluted biotinylated antibody was mixed into each well and incubated at 37 °C for 1 h. The wells were washed three more times with the wash buffer before 100 μL of pre-diluted streptavidin-HRP was added to each well and incubated at 37 °C for 1 h. After three rounds of washing, 90 μL of TMB substrate was added to each well and incubated at 37 °C for 20 min. Lastly, 50 μL of the stop reagent was added to each well, and absorbance was read at 450 nm. Protein concentrations were determined by generating a standard curve, and results were expressed in picograms per milliliter.
2.4.12. Gut microbiota analysis
Five groups (normal control, CTX, positive control, HhLR, and LR) were prepared to analyze the microbiota composition. The HhLR group was selected to represent the hLR treatment groups. Fecal microbial genomic DNA was extracted using a QIAamp DNA stool kit (Qiagen Inc., Hilden, Germany) following the manufacturer’s protocols. The rats’ fecal samples were microbially profiled on the basis of the 16S rRNA gene sequencing amplified by Pacific Biosciences performed in Chun Lab Inc. (Seoul, South Korea). The EzBioCloud Microbiome Taxonomic Profile (MTP) and EZBioCloud 16S database PKSSU4.0 were used to create the MTP from next-generation sequencing (Yoon et al. Citation2017). UCLUST was used to perform high-quality sequence clustering based on operational taxonomic units (OTUs) with a 97% similarity cutoff. The alpha-diversity (Chao 1, ACE, Jackknife, Shannon, and Simpson) and taxonomic profile were screened using EzBiocloud 16S-based MTP software (Yoon et al. Citation2017).
2.5. Statistical analysis
Data were statistically analyzed using GraphPad Prism 8 (GraphPad Software Inc., San Diego, CA, USA), including one-way ANOVA and Tukey’s multiple comparison test. Results were presented as mean ± standard error of the mean (SEM), with p < 0.05 denoting statistical significance.
3. Results
3.1. Inactivation of L. reuteri PSC102
To obtain hLR, we heated L. reuteri PSC102 at different temperatures and time points. The results showed that the loss of viability occurred when the bacteria were subjected to heat treatment at 80 °C for 15 min (). After heat inactivating L. reuteri PSC102, we determined its morphological characteristics via SEM. As shown in , heat treatment (80 °C for 15 min) killed the bacteria, leaving it with slightly unsmooth surfaces. We also obtained the inactivated L. reuteri PSC102 ghost (LRG) by treating it with the minimum bactericidal concentration (MBC) of NaOH (Table S1), which created pores in its structure (Figure S2).
Figure 1. (A) L. reuteri PSC102 was killed at different temperatures (37 °C, 60 °C, 80 °C, and 100 °C) and time points (0, 15, 30, 45, and 60 min). Inactivation was measured by determining the colony forming units per milliliter at 15 min intervals after heat treatment. SEM image of (B) viable and (C) heat-inactivated L. reuteri PSC102.
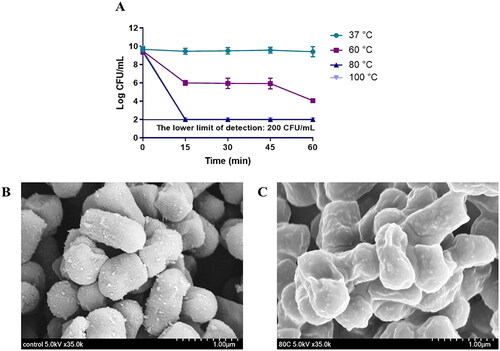
3.2. RAW264.7 cell activation by hLR and LR treatments
We determined whether the hLR, LRG, and LR treatments could affect the activity of RAW264.7 cells. Upon treatment, the phagocytic activity and the expression of iNOS, IL-1β, TNF-α, IL-6, and Cox-2 significantly increased in RAW264.7 cells (p < 0.05; ). The production of NO was also significantly induced (p < 0.05; ). Moreover, hLR, LRG, and LR treatment did not exert any cytotoxic effect on RAW264.7 murine macrophage cells ().
Figure 2. Effects of LR, LRG, and hLR on phagocytic activity, nitric oxide (NO) production, and cytokine expression. RAW264.7 macrophage cells were treated with LR, LRG, and hLR for 24 h. Phagocytic activity was determined by neutral red uptake assay (a). NO production was measured using griess reagent (B), and cytokines were measured via qRT-PCR: (C) iNOS, (D) TNF-α, (E) IL-1β, (F) IL-6, and (G) Cox-2. (H) Cell viability. PBS (vehicle), negative control; LPS (100 ng/mL), positive control; LR, live L. reuteri PSC102 (109 CFU/mL); LRG, L. reuteri PSC102 ghost (109 CFU/mL) and hLR, heat-killed L. reuteri PSC102 (109 CFU/mL). Data are presented as mean ± SEM (n = 3). Different letters (a–d) above the bars indicate significant differences (p < 0.05) between groups.
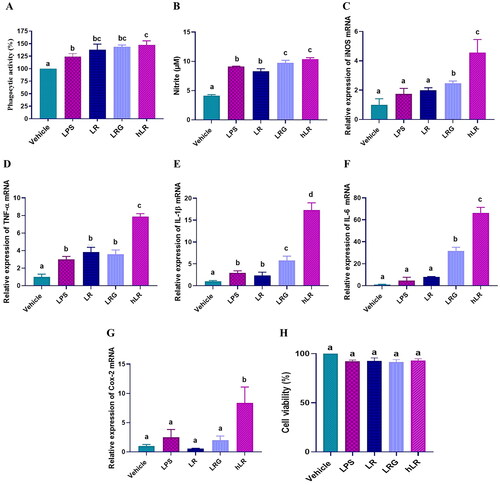
3.3. In vivo immunomodulatory effects in rats
For the animal experiments, hLR and LR were selected on the basis of in vitro results. The samples were orally administered to Sprague–Dawley rats for 3 weeks and assessed whether the immunostimulatory activities could be replicated in vivo.
3.3.1. Body weight analysis
Throughout the experiment, the body weight of the rats in all eight groups was measured regularly (). No significant changes in the initial body weight of the rats were observed during the acclimatization period. After the rats were i.p. injected with CTX, the body weight of the six immunosuppressed rat groups was significantly lower than that of the control group (p < 0.05). However, throughout the remaining study period, the body weights of the hLR (L, M, and H), LR, and positive control groups increased compared with those of the CTX group.
Figure 3. Effects of hLR and LR on body weight (a), thymus and spleen indices (B), and spleen tissues in CTX-treated rats (C). Spleen tissues were stained with H&E for histomorphology images (observed at 200×; scale bar = 100 μm). The white arrows indicate necrotic lesions. Data are expressed as mean ± SEM (n = 8). Significant differences (p < 0.05) between the groups are indicated with different letters (a–b) in superscript.
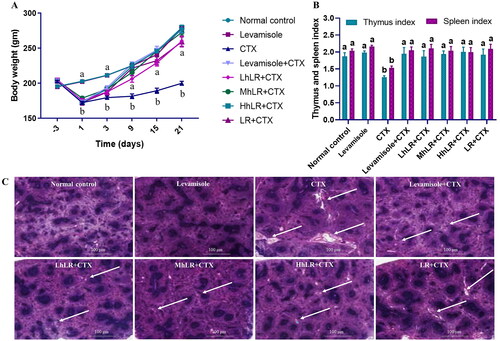
3.3.2. Immune organ indices
The immune organ (thymus and spleen) indices were effectively reduced in the CTX-treated group compared with those in the control group (p < 0.05; ). However, the thymus and spleen indices were considerably higher in the hLR and LR treatment groups than in the CTX group (p < 0.05).
3.3.3. Histological observation of the spleen
Immune system destruction is accompanied by damage to the immune organs. In the present study, the ultrastructure of the spleen was examined by H&E staining to further examine its histomorphological characteristics. The spleen of the normal control group displayed tight and closely arranged spleen cells with no lesions (). In the CTX treatment group, larger necrotic areas were observed in the H&E-stained histopathological images of the spleen cells. However, in the hLR and LR treatment groups, the ultrastructure of the spleen cells showed no lesions, and this finding was comparable with that of the control group. Therefore, hLR and LR treatments could prevent CTX-induced spleen damage in rats.
3.3.4. Effects of hLR and LR on immune cell indices in immunosuppressive rats
To evaluate the effect of hLR and LR on blood immune cells, we determined the number of immune cells in CTX-treated immunosuppressive rats. The WBC, lymphocyte, granulocyte, and MID counts in the CTX group were significantly lower than those in the control group (p < 0.05). However, hLR and LR treatments significantly recovered the CTX-induced decrease in WBC, lymphocyte, granulocyte, and MID counts (p < 0.05; ).
Table 2. Effects of hLR and LR on blood cell counts in CTX-induced immunosuppressive rats.
3.3.5. Effects of hLR and LR on neutrophil migration and phagocytosis
To determine the primary functions of immunity, we assessed the neutrophil migration and phagocytic activities, because neutrophils are potent effector cells that are usually transferred to the site of infection to counteract foreign invaders (Rosales et al. Citation2017). As shown in , the hLR- and LR-treated rats showed a significantly higher neutrophil count than the CTX-treated rats did. Additionally, CTX treatment decreased the migration () and phagocytosis () of neutrophils (p < 0.05). However, hLR (L, M, and H) and LR overturned the impaired migration activity of neutrophils (p < 0.05; ). We also examined the neutrophil phagocytosis activity by using zymosan as a substrate and found that the phagocytic activity of the neutrophils of the hLR- and LR-treated rats significantly increased (p < 0.05; ).
Figure 4. Effects of hLR and LR on neutrophil migration and phagocytosis, and splenocyte proliferation in CTX-induced immunosuppressive rats. A) Total neutrophils count in different groups. B) Neutrophil migration and C) phagocytosis. D) Total splenocyte count in different groups. Ex vivo splenocyte proliferation induced by Con A (E) and LPS (F). Data are expressed as mean ± SEM (n = 4). Different letters (a–f) above the bars indicate significant differences among the groups (p < 0.05).
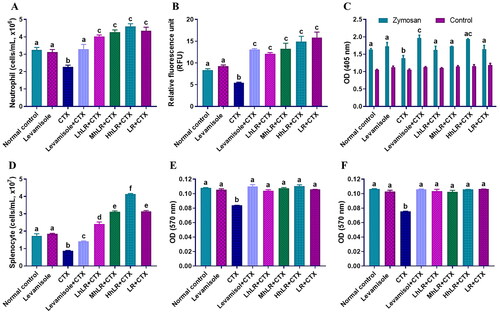
3.3.6. Effects of hLR and LR on primary splenocyte proliferation
Splenocyte proliferation can be used to measure cellular immunity since immunological activation is characterized by immune cell proliferation (Heinzel et al. Citation2018). Splenocytes typically contain various cell types, including macrophages, T lymphocytes, and B lymphocytes. In the present study, the hLR- and LR-treated rats displayed significantly increased spleen cells compared with those in the CTX-treated rats (). Moreover, the proliferation of spleen lymphocytes to mitogen Con A () and LPS () stimuli was significantly suppressed in the CTX-treated rats compared with that in the normal control group. However, hLR and LR treatments significantly increased the splenocyte proliferation in the CTX-treated rats (p < 0.05).
3.3.7. Effects of hLR and LR on the expression of PBMC T cells
We used flow cytometry to analyze the effects of hLR and LR on the expression of T cells in PBMCs (). hLR and LR treatments did not significantly affect the expression of CD4+ and CD8+ T lymphocytes compared with that of the CTX treatment. The CD4+ and CD8+ levels in all the groups were 70%–80% and 20%–30%, respectively (). In CD45RA+ and CD28+ differentiation, HhLR and LR treatments increased the CD45RA+ level but not the CD28+ level ().
Figure 5. Effects of hLR and LR on PBMC T lymphocyte subsets in CTX-treated rats. A) Percentage of CD4+ (R1 region) and CD8+ (R3 region) T cell population. B) Percentage of CD45RA+ (R1 region) and CD28+ (R3 region) T cell population. Data are expressed as mean ± SEM (n = 4). Different letters (a–c) above the bars indicate significant differences (p < 0.05) between groups.
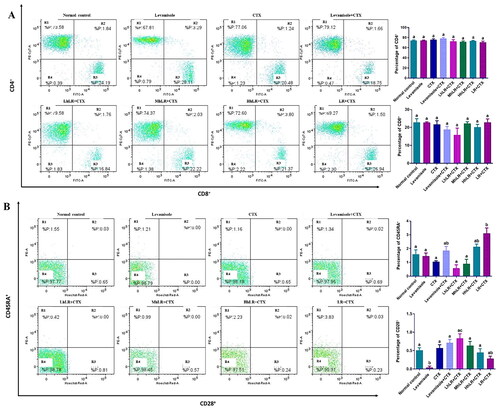
3.3.8. Effects of hLR and LR on the expression of thymic T cells
The effects of hLR and LR on the expression levels of thymic T cells are shown in . The results revealed that CD4+ and CD8+ T cell expression was not significantly suppressed by CTX treatment. The high population of lymphocytes was double positive. However, hLR and LR treatments did not significantly change the expression levels of CD4+ or CD8+ T cells.
3.3.9. Effects of hLR and LR on the expression of splenic T cells
The effects of hLR and LR on the expression of T cells in the spleen are presented in . The percentage of CD4+ in the splenocytes of hLR- and LR-treated groups was significantly higher than that of the CTX-treated group (p < 0.05; ). hLR treatment might favor the differentiation of lymphocytes into CD4+ helper T cells (more than 75%) compared with that of their differentiation into CD8+ cytotoxic T cells (12%–15%; ). In CD45RA+ and CD28+ differentiation, the CD45RA+ expression significantly decreased in the hLR and LR treatment groups, but the CD28+ expression was not statistically significant ().
Figure 7. Effects of hLR and LR on splenic T lymphocyte subsets in CTX-treated rats. A) Percentage of CD4+ (R1 region) and CD8+ (R3 region) T cell population. B) Percentage of CD45RA+ (R1 region) and CD28+ (R3 region) T cell population. Data are expressed as mean ± SEM (n = 4). Different letters (a–b) above the bars indicate significant differences (p < 0.05) between groups.
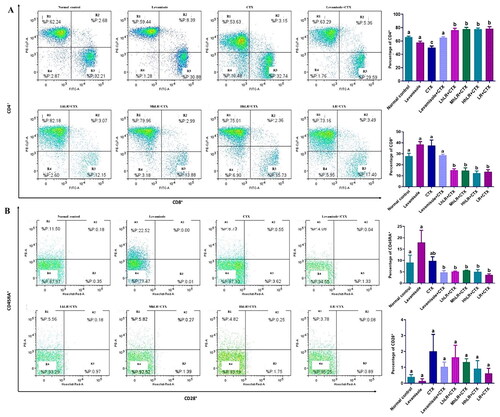
3.3.10. Effects of hLR and LR on cytokine expression
The concentrations of the cytokines IL-2, IL-4, IL-6, interferon (IFN)-γ, TNF-α, IL-10, and IL-12A in the serum of the rats were analyzed to assess the effects of hLR and LR on cytokine production. As shown in , the CTX treatment significantly reduced the levels of cytokines compared with those in the control group (p < 0.05). However, our findings demonstrated that all cytokine levels in the hLR (L, M, and H) and LR treatment groups were significantly higher than those in the CTX group (p < 0.05). Interestingly, hLR could more effectively reverse the CTX-induced attenuation of cytokine expression than LR.
Table 3. Effects of hLR and LR on cytokine levels in serum obtained from CTX-treated rats.
3.3.11. Effects of hLR and LR on gut microbiota modulation
In our study, 449,248 high-quality bacterial gene sequences were obtained from five groups through high-throughput 16S rRNA gene sequencing analysis. The alpha-diversity of the gut microbiota was determined in terms of Chao 1, ACE, Jackknife, Shannon, and Simpson indices (). The community richness can be evaluated using Chao 1, ACE, and Jackknife, while the community diversity in the samples can be assessed using Shannon and Simpson indices. hLR and LR treatments significantly reversed the decrease in Chao 1, ACE, and Jackknife indices in the CTX-treated rats (p < 0.05). The Shannon and Simpson indices increased in the treatment groups, but this increase was not significantly different from that in the CTX group. Taxonomic analysis revealed that Bacteroidetes and Firmicutes were the most abundant gut microbiota, and Prevotella, Fusobacterium, Bacteroides, and Lactobacillus were the most abundant genera in all the groups (). hLR treatment significantly increased the abundance of Bacteroidetes, while LR treatment significantly increased the level of Firmicutes compared with that in the CTX-treated groups. However, the levels of Proteobacteria in all treatment groups were decreased compared to CTX-treated groups (). The taxonomic profiles indicated that the compositions of Prevotella, Fusobacterium, Lactobacillus, and Oscillibacter were modulated by hLR and LR treatments ().
Figure 8. Effects of hLR and LR administration on gut microbiota. (A) Alpha-diversity assessed by Chao 1, ACE, Jackknife, Shannon, and Simpson indices. (B) Composition of gut microbiota at the phylum level. (C) The relative abundance of Bacteroidetes, firmicutes, and proteobacteria. Data are presented as mean ± SEM (n = 3). Different letters (a–c) above the bars indicate significant differences (p < 0.05) between groups.
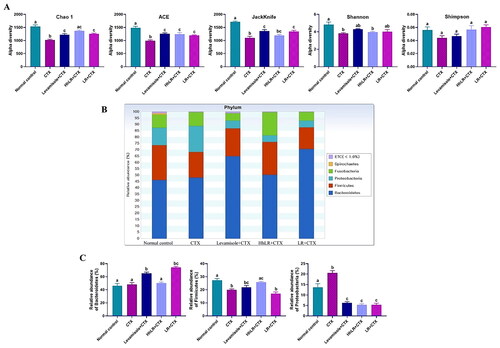
Figure 9. Effects of hLR and LR on the intestinal microbiota taxonomic composition. (A) Composition of gut microbiota at the genus level. (B) The relative abundance of Prevotella, Fusobacterium, Bacteroides, Lactobacillus, and Oscillibacter. Different letters (a–d) above the bars indicate significant differences (p < 0.05) between groups.
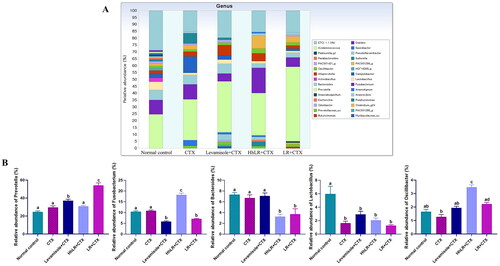
4. Discussion
Probiotics offer a wide range of positive health effects on humans and other animals. Although evidence supporting the use of live probiotics for clinical practices in many countries is widely available, recent studies have suggested that non-viable probiotics containing bioactive compounds may have many advantages over viable ones (Piqué et al. Citation2019). While live probiotics may encounter challenges in adhering to intestinal epithelial cells, which is primarily attributed to the presence of the mucous layer, which acts as a barrier preventing direct contact between bacteria and epithelial cells. However, it is worth noting that non-viable bacteria and their products have the ability to traverse the mucus layer in a more direct manner (Taverniti and Guglielmetti Citation2011). Moreover, the inactivated bacteria do not provide infection and have less possibility of transferring antibiotic resistance. The supernatant of cell-free culture and various soluble components present in probiotic culture media, including metabolites and other substances that are produced, cross the mucous barrier and engage with the mucosal immune cells within the intestinal monolayer (Bermudez-Brito et al. Citation2013). The metabolites produced by Lactobacillus exhibit antioxidant and anti-inflammatory characteristics, exerting their effects primarily on the cells lining of the intestinal mucosa and, subsequently, the immune system (De Marco et al. Citation2018). In relation to immunity, it is commonly observed that most bacteriocin-producing strains possess an immunity mechanism that entails the presence of this immunity protein (Pérez-Ramos et al. Citation2021). Probiotic Lactobacillus can produce a variety of short-chain fatty acids (SCFAs), such as lactic, formic, butyric, and propionic acids, which have been shown to have immunomodulatory properties (Parada Venegas et al. Citation2019). The bioactive peptides synthesized by Lactobacillus rhamnosus 1.0320 can stimulate lymphocyte proliferation, enhance phagocytosis and macrophage maturation, and promote splenic lymphocyte proliferation (Gao et al. Citation2021). The immunomodulatory effects of probiotic lactobacilli have garnered significant attention from researchers, mostly due to their ability to produce EPSs. The evidence indicates that the EPSs released by L. acidophilus DSMZ 20079 have the potential to enhance immune activities through the modulation of cytokines such as IL-2, IL-8, and TNF-α levels (El-Deeb et al. Citation2018). A prior investigation demonstrated that the lipoteichoic acids derived from L. plantarum have the capability to elicit the production of IL-12, consequently promoting the activation of innate immunity in the culture of splenic dendritic cells in mice (Hirose et al. Citation2010). It was revealed that the peptidoglycan obtained from L. rhamnosus exhibited the ability to enhance the innate immune response in immunocompromised mice infected with Streptococcus pneumoniae (Barbieri et al. Citation2017). Surface-layer (S-layer) proteins are composed of proteins and glycoproteins that envelop the cellular surface in different strains of probiotic bacteria. The capacity of the S-layer protein found in L. acidophilus to bind to dendritic cells results in the development of an immunoregulatory phenotype known as Treg. This interaction also contributes to the maintenance of mucosal homeostasis, which has been associated with the ability of probiotic bacteria to induce an immunological response through their binding to dendritic cells (Klotz et al. Citation2020). However, consuming live probiotics may create problems for neonates (Navarro-Tapia et al. Citation2020) and vulnerable patients (Goldenberg et al. Citation2017) mainly because bacteria can move from the gastrointestinal tract to systemic circulation, causing infections and creating antibiotic resistance (Imperial and Ibana Citation2016). Conversely, consuming heat-inactivated probiotics is safer than taking live probiotics (Chen et al. Citation2017); the former also offers a lower risk of antibiotic resistance, exerts biological responses, and restores normal intestinal homeostasis (Piqué et al. Citation2019). Heat-killed probiotics can also provide other benefits, such as extending shelf-life and reducing reactions with other materials (Bernardeau and Cretenet Citation2019). Therefore, the proper selection and subsequent inactivation of probiotics can contribute to the development of new functional foods.
To produce hLR, we first treated the bacteria at different temperatures, and their viability was assessed by counting their CFU per milliliter in MRS media. After confirming the inactivation, we performed SEM analysis and found that the heat inactivation of L. reuteri PSC102 at 80 °C for 15 min left the bacteria with a slightly unsmooth surface. A previous study showed that applying optimum temperature to kill bacteria may produce a slightly uneven surface that can still produce immunomodulatory effects (Ou et al. Citation2011). Then, we used the heat-killed and live L. reuteri PSC102 to investigate the immunomodulatory effects on RAW264.7 cells and Sprague–Dawley rats.
The stimulation of RAW264.7 cells with hLR significantly increased the phagocytic capability and the expression levels of iNOS, IL-6, TNF-α, IL-1β, and Cox-2. The significance of macrophage phagocytic activity in host defense against pathogens is underscored, as it serves as a pivotal component of immunity. Macrophages possess the ability to engulf senescent cells, aberrant cells, and invasive pathogens, thereby augmenting the body’s capacity to combat infections in vivo. Hence, phagocytosis stands as a critical function executed by macrophages (Sueiro-Benavides et al. Citation2021). The upregulation of the cytokine expression is an indicator of immunostimulatory effects (Azad et al. Citation2018). Similarly, hLR increases NO production, one of the most versatile participants in the immune system, which can also cause macrophages to inhibit pathogen replication (Luo et al. Citation2023). Interestingly, hLR displayed higher stimulatory effects on the expression of cytokines and the production of NO than live LR and LRG did. In short, hLR stimulation enhanced RAW264.7 phagocytic activity, likely correlated with elevated nitric oxide (NO) levels and upregulated cytokine expression. In this study, we used heat-killed whole cell lysates to determine the bioactivity of hLR, which could potentially be used as a functional food/feed supplement. Therefore, considering the practical use of hLR in the food sector, we selected whole cell lysates. L. reuteri PSC102 has various structural components, such as teichoic acid, lipoteichoic acids, and peptidoglycans, which may contribute to immunomodulatory effects. Previous studies showed that the cytoplasmic portion and cell wall components of probiotics play immunomodulatory roles (Taverniti and Guglielmetti Citation2011).
CTX is a well-known cytotoxic drug used in cancer treatment. However, CTX as an anticancer agent produces many side effects, including immune organ atrophy and weight loss, bone marrow suppression, and an imbalance in immune blood cells, ultimately inhibiting immune functions (Wang et al. Citation2011). Moreover, administration of CTX can interfere with the proliferation and differentiation of T and B cells as a result of the disruption of the T helper (Th)1/Th2 balance (Yu et al. Citation2015). Hence, to evaluate the immunostimulatory effects of L. reuteri PSC102, rats treated with CTX were used as an immunosuppressed model.
We first assessed body weight, immune organ indices, spleen morphology, and immune blood cell counts. The obtained data revealed that hLR and LR treatment diminished the CTX-induced decrease in body weight, thymus and spleen indices, splenocyte proliferation, and neutrophil phagocytosis. The spleen is among the major organ maintaining immune homeostasis (Cui et al. Citation2016). The H&E results of the spleen indicated that hLR and LR could inhibit the CTX-induced damage on the spleen, revealing that hLR and LR could reverse the effect of immunosuppression in rats. Moreover, the total number of WBCs, lymphocytes, granulocytes, and MID increased compared with that in the CTX-treated rats upon administration of hLR and LR, thereby providing corroborative evidence for their immunostimulatory effects.
The ability of neutrophils to migrate to the site of foreign invaders and subsequently phagocytose them is essential for maintaining host immunity. The neutrophil migration and phagocytosis activity in hLR- and LR-treated groups improved compared with those in the CTX group, indicating that hLR and LR played a vital role in initiating and modulating nonspecific immunity; thus, the immunity of immunosuppressed rats has been improved. Lymphocyte proliferation in response to mitogen is commonly used to determine the efficiency of immunomodulatory agents; therefore, the proliferation of lymphocyte assay has been used to evaluate the effect of probiotics on immune function (Maroof et al. Citation2012). To determine the effect of hLR and LR on cellular immunity, we isolated splenocytes from rats and examined their proliferative activity. The results demonstrated that hLR and LR treatments significantly augmented splenocyte proliferation, suggesting that they could improve humoral and cell-mediated immunity.
Hematopoietic stem cells (HSCs) usually stay in the bone marrow, differentiate into different cell types, and mobilize to the periphery. T cell progenitors move from the bone marrow into the systemic circulation during development and then enter the thymus and spleen (Kisielow Citation2019). Our results showed that the population of thymic T lymphocytes, also known as thymocytes, appeared to be double positive (CD4+ and CD8+), as positive and negative selection have not taken place as part of the maturation process. From the representative dot plots, double-positive thymocytes constituted the majority of the total cell population, regardless of treatment. In the spleen, the total population of splenic T lymphocytes, commonly known as splenocytes, comprises single positive cells expressing either CD4 or CD8. Analysis of the hLR and LR-treated groups reveals an augmented CD4+ splenocyte population and a reduced CD8+ splenocyte population, suggesting potential impacts on CD4/CD8 lineage differentiation due to the treatment. The critical factor influencing lineage selection during T-cell development is the duration of the T-cell receptor (TCR) signaling. Prolonged signals, exemplified by extended exposure to hLR and LR treatment, favor CD4 lineage commitment, while transient signals favor CD8 lineage commitment (Steier et al. Citation2023).
In the immune system, Th1 and Th2 maintain humoral and cellular immunity. Cytokines also have a crucial role in improving immunological responses by regulating T cell proliferation and differentiation. IL-2 is an essential cytokine that helps in the survival and proliferation of T cells (Meng et al. Citation2018). Hence, hLR and LR treatment increased the release of IL-2, which might encourage the proliferation of T cells and the expression of IFN-γ, ultimately improving immune responses. The release of TNF-α and IL-12A is upregulated upon the treatment of Lactobacillus, which may help boost immunity; this finding corroborates our results (Jorjão et al. Citation2015). IL-6, secreted by Th2 cells, can regulate humoral immunity and modulate T cell proliferation and differentiation (Jang et al. Citation2013). IFN-γ helps differentiate Th cells into Th1, such as CD4+ T cells and CD8+ T cells, contributing to cellular immune responses (Chang et al. Citation2008; Li et al. Citation2013). Similarly, IL-4 stimulates the differentiation of Th2 (Keegan et al. Citation2021). IL-10 promotes the differentiation of Tregs (Zhang and Kuchroo Citation2019). The Treg cell CD28+ is expressed on the T cell surface and required for T cell survival. CD28+ produces stimulatory signals, stimulating the production of T cells to recognize invading agents (Boomer and Green Citation2010); the immunoregulatory functions of CD45RA+ cells include the direct suppression of immune responses or the stimulation of a suppressive activity by CD8+ cells (Rocamora-Reverte et al. Citation2020). Therefore, we examined the expression of the cytokines in this study and demonstrated that hLR and LR could reverse the CTX-induced decrease in cytokine concentrations.
The gut microbiota can regulate intestinal functions and immune homeostasis, thereby keeping the balanced health of the host. CTX can change the composition of the gut microbiota, while probiotic administration can modulate the microbiota composition changed by CTX and thus regulate host immunity (Meng et al. Citation2019). Probiotics have the ability to generate metabolic products that exert modulatory effects on the proliferation of other bacteria in the gut. It was shown that EPS, an extracellular macromolecule released by probiotics, can regulate the abundance of the major phyla (Kaur and Dey Citation2022). Furthermore, EPSs derived from L. rhamnosus are being studied due to their modulating effects on the human gut microbiota (Zhu et al. Citation2021). The S-layer proteins of lactobacilli have been demonstrated to enable probiotics to bind to intestinal epithelium cells, thereby modulating the gut microbiome (Guli et al. Citation2021). The other probiotic effector molecules, such as peptidoglycan, teichoic acid, and bacteriocins, can modulate the gut microbiota and improve the homeostasis of the intestine (Gao et al. Citation2022). In the present study, the possible effects of hLR and LR on the gut microbiota in CTX-treated rats were investigated. The results demonstrated that hLR and LR treatments significantly increased the alpha-diversity index of the CTX-treated rats. Poor immune functions are typically linked to limited species and diversity (Rehman et al. Citation2010). Our results indicated that hLR and LR were advantageous for host health. To fully understand the similarities and differences between various groups, we compared the dominating gut microbiota at several taxonomic levels (phylum, family, and genus). The relative abundances of Bacteroidetes and Firmicutes were respectively increased by LR and hLR treatments in the CTX-treated rats. Bacteroidetes and Firmicutes may be involved in various strategies to rely on carbohydrate-active enzymes, thereby contributing to host immunity (Kato et al. Citation2014). Kim et al. (Citation2020) has reported that treatment with L. sakei K040706 in CTX-induced immunosuppressed mice recovered Firmicutes and Bacteroidetes, positively impacting the immune response and gut health. The abundance of Proteobacteria significantly decreased in the sample treatment compared with those in the normal control and CTX-treated groups. This decrease in Proteobacteria may be beneficial for the gut health of the host because it is the major Gram-negative bacterial group comprising a wide range of pathogenic microorganisms, such as Helicobacter pylori, E. coli, and Salmonella (Zheng et al. Citation2017). Prevotella is one of the important members of gut microbiota, which was increased in the treatment groups. Prevotella is associated with cytokine release, which can modulate intestinal functional barriers (Han et al. Citation2021). Moreover, increasing other bacteria, such as Lactobacillus and Oscillibacter, may provide potential immunoregulatory roles. Gram-negative Oscillibacter mainly contains LPS and may trigger the release of cytokines through NF-κB activation (Ding et al. Citation2019). Lactobacillus, another important lactic acid bacteria, may play a crucial role in the immune system to provide healthy immune homeostasis in the host’s digestive tract (Han et al. Citation2021). Lactobacillus can also enhance gut mucosal immunity by stimulating TLR2 receptors in dendritic cells and macrophages (Tsai et al. Citation2010). Therefore, hLR and LR could modulate the gut microbiota composition, suggesting that the gut microbiota linked to immune responses may be involved in immunoregulation.
5. Conclusion
In this study, hLR and LR showed immunomodulatory effects by stimulating RAW264.7 cells. Furthermore, the oral administration of hLR and LR demonstrated the immunomodulatory effects in CTX-treated rats by promoting the development of immune organs and the hematopoietic system, increasing neutrophil migration and phagocytosis, changing lymphocyte proliferation and subpopulation expression, and upregulating cytokine levels. The intervention with hLR and LR modulated the gut microbiota composition, thereby increasing the relative abundance of Bacteroidetes and Firmicutes, which are positively linked to the immune response. Therefore, these results suggested that LR or hLR could be a potential immunostimulatory agent to improve immune functions in humans or other animals. However, further research is still required to elucidate the pharmacology active components in hLR and LR and signaling pathways related to immunomodulation.
Supplemental Material
Download MS Word (740.5 KB)Disclosure statement
No potential conflict of interest was reported by the author(s).
Data availability statement
The data obtained in the present study are available within the article and supplementary materials.
Additional information
Funding
References
- Ahmad W, Jantan I, Kumolosasi E, Bukhari SNA. 2015. Immunostimulatory effects of the standardized extract of Tinospora crispa on innate immune responses in Wistar Kyoto rats. Drug Des Devel Ther. 9:2961–2973. doi: 10.2147/DDDT.S85405.
- Ali MS, Lee E-B, Lim S-K, Suk K, Park S-C. 2023. Isolation and identification of Limosilactobacillus reuteri PSC102 and evaluation of its potential probiotic, antioxidant, and antibacterial properties. Antioxidants. 12(2):238. doi: 10.3390/antiox12020238.
- Ali MS, Lee E-B, Quah Y, Birhanu BT, Suk K, Lim S-K, Park S-C. 2022. Heat-killed Limosilactobacillus reuteri PSC102 ameliorates impaired immunity in cyclophosphamide-induced immunosuppressed mice. Front Microbiol. 13:820838. doi: 10.3389/fmicb.2022.820838.
- Ali S, Birhanu BT, Lee E-B, Quah Y, Boby N, Suk K, Lee S-P, Lee S-J, Park S-C. 2022. Immunomodulatory effects of Bacillus subtilis-fermented soybean extract in mice. Food Biotechnol. 36(4):303–327. doi: 10.1080/08905436.2022.2124265.
- Azad M, Kalam A, Sarker M, Wan D. 2018. Immunomodulatory effects of probiotics on cytokine profiles. Biomed Res Int. 2018:8063647–8063610. doi: 10.1155/2018/8063647.
- Barbieri N, Herrera M, Salva S, Villena J, Alvarez S. 2017. Lactobacillus rhamnosus CRL1505 nasal administration improves recovery of T-cell mediated immunity against pneumococcal infection in malnourished mice. Benef Microbes. 8(3):393–405. doi: 10.3920/BM2016.0152.
- Bermudez-Brito M, Muñoz-Quezada S, Gomez-Llorente C, Matencio E, Bernal MJ, Romero F, Gil A. 2013. Cell-free culture supernatant of Bifidobacterium breve CNCM I-4035 decreases pro-inflammatory cytokines in human dendritic cells challenged with Salmonella typhi through TLR activation. PLOS One. 8(3):e59370. doi: 10.1371/journal.pone.0059370.
- Bernardeau M, Cretenet M. 2019. Probiotic Effects of non-viable lactic acid bacteria. In Lact Acid Bact. Florida, USA: CRC Press; p. 609–629.
- Boomer JS, Green JM. 2010. An enigmatic tail of CD28 signaling. Cold Spring Harb Perspect Biol. 2(8):a002436–a002436. doi: 10.1101/cshperspect.a002436.
- Boumis E, Capone A, Galati V, Venditti C, Petrosillo N. 2018. Probiotics and infective endocarditis in patients with hereditary hemorrhagic telangiectasia: a clinical case and a review of the literature. BMC Infect Dis. 18(1):65. doi: 10.1186/s12879-018-2956-5.
- Castro-González JM, Castro P, Sandoval H, Castro-Sandoval D. 2019. Probiotic lactobacilli precautions. Front Microbiol. 10:375. doi: 10.3389/fmicb.2019.00375.
- Chang S, Collins PL, Aune TM. 2008. T-bet dependent removal of Sin3A-histone deacetylase complexes at the Ifng Locus drives Th1 differentiation. J Immunol. 181(12):8372–8381. doi: 10.4049/jimmunol.181.12.8372.
- Chen M-F, Weng K-F, Huang S-Y, Liu Y-C, Tseng S-N, Ojcius DM, Shih S-R. 2017. Pretreatment with a heat-killed probiotic modulates monocyte chemoattractant protein-1 and reduces the pathogenicity of influenza and enterovirus 71 infections. Mucosal Immunol. 10(1):215–227. doi: 10.1038/mi.2016.31.
- Colella M, Charitos IA, Ballini A, Cafiero C, Topi S, Palmirotta R, Santacroce L. 2023. Microbiota revolution: how gut microbes regulate our lives. World J Gastroenterol. 29(28):4368–4383. doi: 10.3748/wjg.v29.i28.4368.
- Cui H, Li T, Wang L, Su Y, Xian CJ. 2016. Dioscorea bulbifera polysaccharide and cyclophosphamide combination enhances anti-cervical cancer effect and attenuates immunosuppression and oxidative stress in mice. Sci Rep. 5:19185. doi: 10.1038/srep19185.
- Ding Y, Yan Y, Chen D, Ran L, Mi J, Lu L, Jing B, Li X, Zeng X, Cao Y. 2019. Modulating effects of polysaccharides from the fruits of: Lycium barbarum on the immune response and gut microbiota in cyclophosphamide-treated mice. Food Funct. 10(6):3671–3683. doi: 10.1039/c9fo00638a.
- El-Deeb NM, Yassin AM, Al-Madboly LA, El-Hawiet A. 2018. A novel purified Lactobacillus acidophilus 20079 exopolysaccharide, LA-EPS-20079, molecularly regulates both apoptotic and NF-κB inflammatory pathways in human colon cancer. Microb Cell Fact. 17(1):29. doi: 10.1186/s12934-018-0877-z.
- Fei W, Yue N, Li A, Yu S, Zhao D, Zhu Y, Wang C, Zhang J, Wang L. 2022. Immunomodulatory effects of Lepidium meyenii Walp. Polysaccharides on an immunosuppression model induced by cyclophosphamide. J Immunol Res. 2022:1210890. doi: 10.1155/2022/1210890.
- Gao D, Liu Z, Liu F, Chen L, Wang W, Ma J, Xu C, Jiang Z, Hou J. 2021. Study of the immunoregulatory effect of Lactobacillus rhamnosus 1.0320 in immunosuppressed mice. J Funct Foods. 79:104423. doi: 10.1016/j.jff.2021.104423.
- Gao X, Zhao J, Zhang H, Chen W, Zhai Q. 2022. Modulation of gut health using probiotics: the role of probiotic effector molecules. J Futur Foods [Internet]. 2(1):1–12. doi: 10.1016/j.jfutfo.2022.03.011.
- Goldenberg JZ, Yap C, Lytvyn L, Lo CK-F, Beardsley J, Mertz D, Johnston BC. 2017. Probiotics for the prevention of Clostridium difficile-associated diarrhea in adults and children. Cochrane Database Syst Rev. 12(12):CD006095. doi: 10.1002/14651858.CD006095.pub4.
- Guli M, Winarsih S, Barlianto W, Illiandri O, Sumarno SP. 2021. Mechanism of Lactobacillus reuteri probiotic in increasing intestinal mucosal immune system. Open Access Maced J Med Sci. 9(F):784–793. doi: 10.3889/oamjms.2021.7447.
- Håkansson Å, Tormo-Badia N, Baridi A, Xu J, Molin G, Hagslätt M-L, Karlsson C, Jeppsson B, Cilio CM, Ahrné S. 2015. Immunological alteration and changes of gut microbiota after dextran sulfate sodium (DSS) administration in mice. Clin Exp Med. 15(1):107–120. doi: 10.1007/s10238-013-0270-5.
- Han S-K, Shin Y-J, Lee D-Y, Kim KM, Yang S-J, Kim DS, Choi J-W, Lee S, Kim D-H. 2021. Lactobacillus rhamnosus HDB1258 modulates gut microbiota-mediated immune response in mice with or without lipopolysaccharide-induced systemic inflammation. BMC Microbiol. 21(1):146. doi: 10.1186/s12866-021-02192-4.
- Heinzel S, Marchingo JM, Horton MB, Hodgkin PD. 2018. The regulation of lymphocyte activation and proliferation. Curr Opin Immunol. 51:32–38. doi: 10.1016/j.coi.2018.01.002.
- Hirose Y, Murosaki S, Fujiki T, Yamamoto Y, Yoshikai Y, Yamashita M. 2010. Lipoteichoic acids on Lactobacillus plantarum cell surfaces correlate with induction of interleukin-12p40 production. Microbiol Immunol. 54(3):143–151. doi: 10.1111/j.1348-0421.2009.00189.x.
- Imperial ICVJ, Ibana JA. 2016. Addressing the antibiotic resistance problem with probiotics: reducing the risk of its double-edged sword effect. Front Microbiol. 7:1983. doi: 10.3389/fmicb.2016.01983.
- Indira M, Venkateswarulu TC, Abraham Peele K, Nazneen Bobby M, Krupanidhi S. 2019. Bioactive molecules of probiotic bacteria and their mechanism of action: a review. 3 Biotech. 9(8):306. doi: 10.1007/s13205-019-1841-2.
- Jang S-E, Joh E-H, Ahn Y-T, Huh C-S, Han MJ, Kim D-H. 2013. Lactobacillus casei HY7213 ameliorates cyclophosphamide-induced immunosuppression in mice by activating NK, cytotoxic T cells and macrophages. Immunopharmacol Immunotoxicol. 35(3):396–402. doi: 10.3109/08923973.2013.789055.
- Jørgensen MR, Kragelund C, Jensen PØ, Keller MK, Twetman S. 2017. Probiotic Lactobacillus reuteri has antifungal effects on oral Candida species in vitro. J Oral Microbiol. 9(1):1274582. doi: 10.1080/20002297.2016.1274582.
- Jorjão AL, Oliveira FEd, Leão MVP, Carvalho CAT, Jorge AOC, Oliveira LDd 2015. 2015. Lactobacillus rhamn Live and heat-killedosus ATCC 7469 may induce modulatory cytokines profiles on macrophages RAW 264.7. Sci World J. 2015:1–6. doi: 10.1155/2015/716749.
- Katkowska M, Garbacz K, Kusiak A. 2021. Probiotics: should all patients take them? Microorganisms. 9(12):2620. doi: 10.3390/microorganisms9122620.
- Kato LM, Kawamoto S, Maruya M, Fagarasan S. 2014. The role of the adaptive immune system in regulation of gut microbiota. Immunol Rev. 260(1):67–75. doi: 10.1111/imr.12185.
- Kaur N, Dey P. 2022. Bacterial exopolysaccharides as emerging bioactive macromolecules: from fundamentals to applications. Res Microbiol. 174(4):104024. doi: 10.1016/j.resmic.2022.104024.
- Keegan AD, Leonard WJ, Zhu J. 2021. Recent advances in understanding the role of IL-4 signaling. Fac Rev. 10:71. doi: 10.12703/r/10-71.
- Kim S-Y, Shin J-S, Chung K-S, Han H-S, Lee H-H, Lee J-H, Kim S-Y, Ji YW, Ha Y, Kang J, et al. 2020. Immunostimulatory effects of live Lactobacillus sakei K040706 on the CYP-Induced immunosuppression mouse model. Nutrients. 12(11):3573. doi: 10.3390/nu12113573.
- Kisielow P. 2019. How does the immune system learn to distinguish between good and evil? The first definitive studies of T cell central tolerance and positive selection. Immunogenetics. 71(8–9):513–518. doi: 10.1007/s00251-019-01127-8.
- Klotz C, Goh YJ, O’Flaherty S, Barrangou R. 2020. S-layer associated proteins contribute to the adhesive and immunomodulatory properties of Lactobacillus acidophilus NCFM. BMC Microbiol. 20(1):248. doi: 10.1186/s12866-020-01908-2.
- Kothari D, Patel S, Kim S-K. 2019. Probiotic supplements might not be universally-effective and safe: a review. Biomed Pharmacother. 111:537–547. doi: 10.1016/j.biopha.2018.12.104.
- Lee HY, Park YM, Lee YH, Kang YG, Lee HM, Park DS, Yang HJ, Kim MJ, Lee Y-R. 2018. Immunostimulatory Effect of Zanthoxylum schinifolium-based complex oil prepared by supercritical fluid extraction in splenocytes and cyclophosphamide-induced immunosuppressed rats. Tomczyk M , editor. Evid Based Complement Alternat Med. 2018:8107326–8107310. doi: 10.1155/2018/8107326.
- Lee J, Jung I, Choi JW, Lee CW, Cho S, Choi TG, Sohn M, Park YI. 2019. Micronized and Heat-Treated Lactobacillus plantarum LM1004 stimulates host immune responses via the TLR-2/MAPK/NF-κB signalling pathway in vitro and in vivo. J Microbiol Biotechnol. 29(5):704–712. doi: 10.4014/jmb.1812.12059.
- Li J, Cheng Y, Zhang X, Zheng L, Han Z, Li P, Xiao Y, Zhang Q, Wang F. 2013. The in vivo immunomodulatory and synergistic anti-tumor activity of thymosin α1-thymopentin fusion peptide and its binding to TLR2. Cancer Lett. 337(2):237–247. doi: 10.1016/j.canlet.2013.05.006.
- Li L, Fang Z, Liu X, Hu W, Lu W, Lee Y-K, Zhao J, Zhang H, Chen W. 2020. Lactobacillus reuteri attenuated allergic inflammation induced by HDM in the mouse and modulated gut microbes. PLOS One. 15(4):e0231865. doi: 10.1371/journal.pone.0231865.
- Luo Z, Chen A, Xie A, Liu X, Jiang S, Yu R. 2023. Limosilactobacillus reuteri in immunomodulation: molecular mechanisms and potential applications. Front Immunol. 14:1228754. doi: 10.3389/fimmu.2023.1228754.
- De Marco S, Sichetti M, Muradyan D, Piccioni M, Traina G, Pagiotti R, Pietrella D. 2018. Probiotic cell-free supernatants exhibited anti-inflammatory and antioxidant activity on human gut epithelial cells and macrophages stimulated with LPS. Evi-Based Comple Altern Med. 2018:1–12. doi: 10.1155/2018/1756308.
- Maroof H, Hassan ZM, Mobarez AM, Mohamadabadi MA. 2012. Lactobacillus acidophilus could modulate the immune response against breast cancer in murine model. J Clin Immunol. 32(6):1353–1359. doi: 10.1007/s10875-012-9708-x.
- Meng Y, Li B, Jin D, Zhan M, Lu J, Huo G. 2018. Immunomodulatory activity of Lactobacillus plantarum KLDS1.0318 in cyclophosphamide-treated mice. Food Nutr Res. 62(0):1–9. doi: 10.29219/fnr.v62.1296.
- Meng Y, Wang J, Wang Z, Zhang G, Liu L, Huo G, Li C. 2019. Lactobacillus plantarum KLDS1.0318 ameliorates impaired intestinal immunity and metabolic disorders in cyclophosphamide-treated mice. Front Microbiol. 10:731. doi: 10.3389/fmicb.2019.00731.
- Miyazawa K, Kawase M, Kubota A, Yoda K, Harata G, Hosoda M, He F. 2015. Heat-killed Lactobacillus gasseri can enhance immunity in the elderly in a double-blind, placebo-controlled clinical study. Benef Microbes. 6(4):441–449. doi: 10.3920/BM2014.0108.
- Nakai H, Murosaki S, Yamamoto Y, Furutani M, Matsuoka R, Hirose Y. 2021. Safety and efficacy of using heat-killed Lactobacillus plantarum L-137: high-dose and long-term use effects on immune-related safety and intestinal bacterial flora. J Immunotoxicol. 18(1):127–135. doi: 10.1080/1547691X.2021.1979698.
- Navarro-Tapia E, Sebastiani G, Sailer S, Almeida Toledano L, Serra-Delgado M, García-Algar Ó, Andreu-Fernández V. 2020. Probiotic supplementation during the perinatal and infant period: effects on gut dysbiosis and disease. Nutrients. 12(8):2243. doi: 10.3390/nu12082243.
- Noh EM, Kim JM, Lee HY, Song HK, Joung SO, Yang HJ, Kim MJ, Kim KS, Lee YR. 2019. Immuno-enhancement effects of Platycodon grandiflorum extracts in splenocytes and a cyclophosphamide-induced immunosuppressed rat model. BMC Complement Altern Med. 19(1):322. doi: 10.1186/s12906-019-2724-0.
- Ou C, Lin S, Tsai J, Lin M. 2011. Heat-killed lactic acid bacteria enhance immunomodulatory potential by skewing the immune response toward Th1 polarization. J Food Sci. 76(5):M260–M267.
- Parada Venegas D, De la Fuente MK, Landskron G, González MJ, Quera R, Dijkstra G, Harmsen HJM, Faber KN, Hermoso MA. 2019. Short chain fatty acids (SCFAs)-mediated gut epithelial and immune regulation and its relevance for inflammatory bowel diseases. Front Immunol. 10:277. doi: 10.3389/fimmu.2019.00277.
- Pérez-Ramos A, Madi-Moussa D, Coucheney F, Drider D. 2021. Current knowledge of the mode of action and immunity mechanisms of LAB-bacteriocins. Microorganisms. 9(10):2107. doi: 10.3390/microorganisms9102107.
- Piqué N, Berlanga M, Miñana-Galbis D. 2019. Health benefits of heat-killed (Tyndallized) probiotics: an overview. Int J Mol Sci. 20(10):2534. doi: 10.3390/ijms20102534.
- Quah Y, Lee S-J, Lee E-B, Birhanu BT, Ali MS, Abbas MA, Boby N, Im Z-E, Park S-C. 2020. Cornus officinalis ethanolic extract with potential anti-allergic, anti-inflammatory, and antioxidant activities. Nutrients. 12(11):3317. doi: 10.3390/nu12113317.
- Rehman A, Lepage P, Nolte A, Hellmig S, Schreiber S, Ott SJ. 2010. Transcriptional activity of the dominant gut mucosal microbiota in chronic inflammatory bowel disease patients. J Med Microbiol. 59(Pt 9):1114–1122. doi: 10.1099/jmm.0.021170-0.
- Rocamora-Reverte L, Melzer FL, Würzner R, Weinberger B. 2020. The complex role of regulatory T cells in immunity and aging. Front Immunol. 11:616949. doi: 10.3389/fimmu.2020.616949.
- Rohm TV, Meier DT, Olefsky JM, Donath MY. 2022. Inflammation in obesity, diabetes, and related disorders. Immunity. 55(1):31–55. doi: 10.1016/j.immuni.2021.12.013.
- Rosales C, Lowell CA, Schnoor M, Uribe-Querol E. 2017. Neutrophils: their role in innate and adaptive immunity 2017. J Immunol Res. 2017:1–2. doi: 10.1155/2017/9748345.
- Steier Z, Aylard DA, McIntyre LL, Baldwin I, Yoon Kim EJ, Lutes LK, Ergen C, Huang T-S, Robey EA, Yosef N. 2023. Single-cell multiomic analysis of thymocyte development reveals drivers of CD4/CD8 lineage commitment. Nat Immunol. 24(9):1579–1590. doi: 10.1038/s41590-023-01584-0.
- Sueiro-Benavides RA, Leiro-Vidal JM, Salas-Sánchez AÁ, Rodríguez-González JA, Ares-Pena FJ, López-Martín ME. 2021. Radiofrequency at 2.45 GHz increases toxicity, pro-inflammatory and pre-apoptotic activity caused by black carbon in the RAW 264.7 macrophage cell line. Sci Total Environ. 765:142681. doi: 10.1016/j.scitotenv.2020.142681.
- Taverniti V, Guglielmetti S. 2011. The immunomodulatory properties of probiotic microorganisms beyond their viability (ghost probiotics: proposal of paraprobiotic concept). Genes Nutr. 6(3):261–274. doi: 10.1007/s12263-011-0218-x.
- Tsai Y-T, Cheng P-C, Liao J-W, Pan T-M. 2010. Effect of the administration of Lactobacillus paracasei subsp. paracasei NTU 101 on Peyer’s patch-mediated mucosal immunity. Int Immunopharmacol. 10(7):791–798. doi: 10.1016/j.intimp.2010.04.012.
- Wang H, Wang M, Chen J, Tang Y, Dou J, Yu J, Xi T, Zhou C. 2011. A polysaccharide from Strongylocentrotus nudus eggs protects against myelosuppression and immunosuppression in cyclophosphamide-treated mice. Int Immunopharmacol. 11(11):1946–1953. doi: 10.1016/j.intimp.2011.06.006.
- Weiss GA, Hennet T. 2017. Mechanisms and consequences of intestinal dysbiosis. Cell Mol Life Sci. 74(16):2959–2977. doi: 10.1007/s00018-017-2509-x.
- Yatim KM, Lakkis FG. 2015. A brief journey through the immune system. Clin J Am Soc Nephrol. 10(7):1274–1281. doi: 10.2215/CJN.10031014.
- Yeşilyurt N, Yılmaz B, Ağagündüz D, Capasso R. 2021. Involvement of probiotics and postbiotics in the immune system modulation. Biologics. 1(2):89–110. doi: 10.3390/biologics1020006.
- Yoon S-H, Ha S-M, Kwon S, Lim J, Kim Y, Seo H, Chun J. 2017. Introducing EzBioCloud: a taxonomically united database of 16S rRNA gene sequences and whole-genome assemblies. Int J Syst Evol Microbiol. 67(5):1613–1617. doi: 10.1099/ijsem.0.001755.
- Yu Q, Nie S-P, Wang J-Q, Huang D-F, Li W-J, Xie M-Y. 2015. Molecular mechanism underlying chemoprotective effects of Ganoderma atrum polysaccharide in cyclophosphamide-induced immunosuppressed mice. J Funct Foods. 15:52–60. doi: 10.1016/j.jff.2015.03.015.
- Zhang H, Kuchroo V. 2019. Epigenetic and transcriptional mechanisms for the regulation of IL-10. Semin Immunol. 44 :101324. doi: 10.1016/j.smim.2019.101324.
- Zhang WN, Gong LL, Liu Y, Zhou ZB, Wan CX, Xu JJ, Wu QX, Chen L, Lu YM, Chen Y. 2020. Immunoenhancement effect of crude polysaccharides of Helvella leucopus on cyclophosphamide-induced immunosuppressive mice. J Funct Foods. 69:103942. doi: 10.1016/j.jff.2020.103942.
- Zhang Z, Hu X, Lin L, Ding G, Yu F. 2019. Immunomodulatory activity of low molecular-weight peptides from Nibea japonica in RAW264. 7 cells via NF-κB pathway. Mar Drugs. 17(7):404. doi: 10.3390/md17070404.
- Zheng C, Liu R, Xue B, Luo J, Gao L, Wang Y, Ou S, Li S, Peng X. 2017. Impact and consequences of polyphenols and fructooligosaccharide interplay on gut microbiota in rats. Food Funct. 8(5):1925–1932. doi: 10.1039/c6fo01783e.
- Zhu Y, Zhou J-M, Liu W, Pi X, Zhou Q, Li P, Zhou T, Gu Q. 2021. Effects of exopolysaccharide from Lactobacillus rhamnosus on human gut microbiota in in vitro fermentation model. LWT. 139:110524. doi: 10.1016/j.lwt.2020.110524.