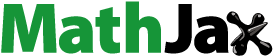
Abstract
Additive manufacturing (AM) allows for the fabrication of custom orthopaedic implant devices which have complex geometries and similar mechanical properties to bone. This paper reviews the corrosion, fatigue and wear properties of AM Ti alloys to confirm their safety for use in orthopaedic implants. Specifically, AM Ti lattice geometries are highlighted due to their improved osseointegration and better modulus matching with that of bone, making them an attractive option for more durable implant devices. Finally, the properties of current implants made via AM are compared with that made via conventional manufacturing methods to confirm their overall safety.
Introduction
Titanium alloys are commonly used in the biomedical industry due to their compatibility with body tissue [Citation1]. Specifically, orthopaedic implant devices require good biocompatibility with the surrounding tissue, good corrosion resistance and matching mechanical properties with that of bone, all of which are fulfilled with the use of Ti alloys [Citation2]. Since the first joint arthroplasty involving Ti alloys in the 1940s [Citation3], Ti orthopaedic implants have become widespread, mostly as standardised, commercially available devices [Citation4] that are mass-produced to address a specific clinical need. In the United States alone, it is projected that 572,000 hip and 3.48 million knee arthroplasties will be performed in 2030 [Citation5], demonstrating the large market for orthopaedic implants. Furthermore, as life expectancy and obesity rates increase worldwide [Citation6], so does the incidence of arthritis requiring joint replacements [Citation7].
In recent years, additive manufacturing (AM) of orthopaedic implants is becoming increasingly popular due to its ability to produce complex geometries, therefore overcoming the limitations of conventional manufacturing techniques [Citation8], such as shown in Figure . It is predicted that the market for additively manufactured devices will reach USD 26 billion by 2022 [Citation9]. Furthermore, AM allows for the production of custom-shaped implants, as well as the production of hollow structures, or lattices, to better mimic the structure of porous bone. In cage-like lattice implants, osseointegration and bone ingrowth determine their long-term success and durability, ensuring bioadhesion with surrounding bone which reduces the probability of implant loosening and the need for revision surgeries [Citation10–12].
Figure 1. Patient-specific AM Ti–6Al–4V implant. (Reproduced from [Citation13].)
![Figure 1. Patient-specific AM Ti–6Al–4V implant. (Reproduced from [Citation13].)](/cms/asset/8406ef7a-1844-4bd6-bec5-90519121510a/ymst_a_2230417_f0001_oc.jpg)
Porous orthopaedic Ti-based implants have been extensively studied in the literature for a wide variety of applications. Bittredge et al. [Citation11] have fabricated and optimised Ti–6Al–4V lattices for total shoulder implants, where 21% of orthopaedic revision surgeries is caused by the loosening of shoulder implants from the humerus bone [Citation14]. Ramhamadany et al. [Citation15] developed a lattice cage structure for talus replacements, which allows 75–90% of its entire volume to be filled with bone graft, enhancing osteogenic potential [Citation16]. This is especially important to treat avascular necrosis of the talus which has very little blood supply [Citation17]. Kuslich et al. [Citation18] have successfully trialled an open-cell lumbar cage in 196 patients presenting with degenerative invertebral disc disease, with a 95.1% bone fusion rate in four years. Popov et al. [Citation19] have successfully implanted a lattice structure to replace bone affected by osteosarcoma resection in canines, thereby providing a viable alternative to amputation. These examples show the breadth of applications of AM Ti implants, which allow for a patient-specific design in a cost-time competitive manner [Citation20–22].
Moreover, current orthopaedic implants are required to be approved by relevant regulatory bodies, such as the Food and Drug Administration in the United States [Citation23] and the CE (Conformite Europeenne) mark in the European Union [Citation24]. Devices such as newly developed implants need to pass pre-clinical testing through clinical studies to assess the efficacy, safety and durability of the devices by means of mechanical and biocompatibility testing. Furthermore, post-market follow-up is also required, where patient monitoring post-operation aims to minimise the need for revision surgeries [Citation25]. In contrast, there is a lack of regulatory requirements for custom-made orthopaedic implants using AM as they are not mass produced [Citation26], lowering the barriers to entry for AM companies in producing orthopaedic implants. It remains the responsibility of surgeons who implant these devices to ensure that the quality and safety of the devices are adequate [Citation27].
Despite being corrosion-resistant by means of a protective surface oxide layer, Ti alloys have been found to corrode in vivo especially in the presence of fatigue loading [Citation28], shown in Figure . This is a non-trivial issue as it often causes implant failure [Citation29–31] as well as the release of metallic ions and debris, which can lead to tissue inflammation and infection [Citation32]. Corrosion in head-neck taper connections in femoral hip prostheses made from Ti alloys occurs in 16–35% of cases according to Gilbert et al. [Citation33]. Furthermore, there are significant challenges in AM implants, such as the critical angle of overhanging structures [Citation34] in addition to inherent defects such as porosity and surface roughness from the AM process [Citation35].
Figure 2. Schematic illustration showing the complex interactions between the material's surface and the physiological environment. (Reproduced from [Citation36].)
![Figure 2. Schematic illustration showing the complex interactions between the material's surface and the physiological environment. (Reproduced from [Citation36].)](/cms/asset/a0de7197-d3f7-4489-95ba-771a12547146/ymst_a_2230417_f0002_oc.jpg)
This paper aims to review the corrosion, fatigue and wear behaviour of AM Ti alloys for orthopaedic implants by systematically looking at:
Manufacturing methods of orthopaedic implants using conventional methods as well as additive manufacturing
Corrosion behaviour of conventionally manufactured and AM Ti alloys for orthopaedic implant applications
Fatigue and corrosion fatigue behaviour of conventionally manufactured and AM Ti alloys for orthopaedic implant applications
Wear and corrosion wear behaviour of conventionally manufactured and AM Ti alloys for orthopaedic implant applications
Comparing AM and conventionally manufactured orthopaedic implants
Future prospects of AM orthopaedic implants.
Manufacturing methods
Conventionally manufactured orthopaedic implants are typically made via forging, where metal is shaped using compressive forces through dies, presses and/or hammers [Citation37]. Most orthopaedic implants are manufactured using closed-die forging to its final shape [Citation38]. Implant fixation is often necessary to provide stability to the surrounding bone, such as using acrylic cement [Citation39]. Depending on the location and complexity of the implant, it is also possible to have cementless fixation which involves a press-fit between the bone and implant [Citation40]. Fixation methods are highly dependent on the implant design, patient's age and surrounding bone quality. However, in the past the use of cement fixation has only proved successful in older patients and less so in younger patients who tend to be more active [Citation41]. Some implants, such as hip tapers, are manufactured from wrought alloys [Citation42, Citation43].
AM of implants
AM of orthopaedic implants is extremely lucrative as it has the ability to produce components with mechanical properties as similar to native bones [Citation44, Citation45]. The complex structure of bone can be replicated, such as its anisotropy [Citation46] and specific internal architecture containing macropores [Citation47]. Moreover, the use of computer-aided design (CAD) to manufacture the implants allows for patient-specific components which significantly reduces operation time while also catering for patients with unique implant requirements [Citation27]. For example, AM of implants can often salvage the joint, allowing joint preservation post-operation such as shown in Figure .
Figure 3. Using additive manufacturing to produce complex implant geometries allowing for joint preservation. (Reproduced from [Citation48].)
![Figure 3. Using additive manufacturing to produce complex implant geometries allowing for joint preservation. (Reproduced from [Citation48].)](/cms/asset/a1d4f4b2-6719-424f-ac84-955065b6eb06/ymst_a_2230417_f0003_oc.jpg)
Various types of experiments involving additively manufactured Ti–6Al–4V implants have been performed in the literature. These involve different AM techniques, which include selective laser melting (SLM), electron beam melting (EBM), laser powder bed fusion (L-PBF) and wire arc additive manufacturing (WAAM), among others. A few examples of previous work involving corrosion of AM Ti–6Al–4V for biomedical applications are summarised in Table .
Table 1. Previous studies involving corrosion of additively manufactured Ti–6Al–4V in the literature.
Different AM techniques produce different microstructures which therefore influence corrosion resistance. As such, studies involving different AM techniques may not be directly comparable with each other. For example, Bai et al. [Citation49] showed that the corrosion resistance of electron beam melted Ti–6Al–4V is better than that of wrought Ti–6Al–4V due to a more refined microstructure and a more compact protective oxide layer. Meanwhile, it has been found that Ti–6Al–4V manufactured by SLM typically has a higher corrosion rate in 3.5% NaCl compared to one conventionally manufactured due to a highly strained martensitic structure and therefore a more porous oxide layer [Citation50–52]. In contrast, the oxide film of SLM Ti–6Al–4V has been found to be more protective in PBS and SBF compared to EBM Ti–6Al–4V [Citation53–55]. Metalnikov et al. [Citation56] also corroborated this finding by comparing EBM and SLM Ti–6Al–4V, with the latter having a slightly better corrosion resistance than the former.
AM lattice geometries
One of the main advantages of AM is the fabrication of lattice structures through CAD, allowing the production of complex shapes requiring minimal post-processing [Citation66, Citation67]. A significant proportion of studies [Citation68–71] involving lattices utilised repeated unit cells throughout the geometry. These geometries comprise beams with a certain thickness, called struts, joined at nodes throughout the lattice. An advantage of strut-based lattices is the ease of design and manufacture, as well as the simplicity of design which allows for reliable computational modelling [Citation70]. However, strut-based structures have sharp curvatures [Citation8], with nodes acting stress concentration sites susceptible to fatigue failure [Citation71]. Additionally, thin struts (1 mm or thinner) are more prone to edge effects [Citation69] further reducing fatigue life.
A better option than struts are surface-based lattices, which have smooth curvatures [Citation72, Citation73], therefore promotes improved cell attachment and better osseointegration for orthopaedic implants. Among surface-based lattices, triple-periodical minimum surfaces (TPMS), shown in Figure , have been found to have zero mean curvature with a single interconnected domain [Citation8]. The lack of stress-concentration sites also reduce fatigue initiation sites, prolonging implant life. However, surface-based lattices are difficult to manufacture. Several studies have investigated different TPMS geometries [Citation74–76]. Kapfer et al. [Citation77] used finite element analysis to determine the mechanical properties of different TPMS geometries, however, it did not take into account surface roughness from the partial sintering of unmelted powders typical of AM.
Figure 4. Various TMPS geometries. (Reproduced from [Citation78].)
![Figure 4. Various TMPS geometries. (Reproduced from [Citation78].)](/cms/asset/0bdf8e56-78b4-44a4-84fe-9bc4e10a35ac/ymst_a_2230417_f0004_oc.jpg)
Lattice geometry is vital for bone regrowth. Zadpoor [Citation73] has studied this extensively, noting that the pores within the lattice should allow for not only mechanical support but also tissue invasion, cell nutrition and oxygenation, and that a minimum pore size of around 300 μm is essential for bone ingrowth and formation of capillaries [Citation79]. Similarly, Gotz et al. [Citation80] noted a minimum of 200 μm pore size is required for osseointegration. Moreover, the curvature of pores also strongly influences bone growth rates [Citation81, Citation82]. In particular, the rate of bone regeneration process has been found to increase with curvature [Citation83, Citation84]. Most importantly, a lattice geometry reduces the overall stiffness of the implant, therefore reducing the extent of stress-shielding [Citation85].
Besides lattices with repeating unit cells, some studies have looked at stochastic lattices, which are anisotropic and therefore more similar to bone. These types of lattices are much less studied than periodic ones. One type of stochastic lattices is Voronoi, based on a mathematical model initially described by Dirichlet and Voronoi in the early twentieth century. This model started as ‘seeds’ in a three-dimensional shape and a circle is drawn around each seed, expanding in radius at the same rate until two circles touch. This results in a three-dimensional diagram of circle boundaries which correspond to lattice scaffolds. Voronoi structures therefore are random lattices, dependent on the number of seeds initially used, which in turn is related to a target pore size [Citation86]. Another type of stochastic lattices can be generated using a Poisson disk algorithm which populates a three-dimensional volume with random points, studied by Ghouse et al. [Citation87]. These points are later joined with lines to form struts. This structure is therefore governed by the minimum proximity between points which determines the strut lengths [Citation88]. For stochastic lattices, it is even more difficult to predict the mechanical properties of these structures, however some models have described a relationship between density and mechanical properties of porous structures. One such model was developed by Gibson and Ashby [Citation89], which show that the strength and modulus of a porous material increase with density by a power law. This has been found to apply to additively manufactured lattices by Yan et al. [Citation90].
Subsequently, orthopaedic implant applications comprising different AM lattice geometries have varying corrosion properties. The following section aims to investigate corrosion mechanisms and properties of AM Ti alloys for orthopaedic implants.
Corrosion
Ti alloys are generally considered to be corrosion resistant owing to the spontaneous formation of a protective oxide layer on the metal surface, acting as a physical barrier between corrosive species and titanium metal [Citation91]. However, Ti alloys are not immune to corrosion once the passive layer is compromised [Citation92], and many studies have investigated the mechanism and extent of corrosion of Ti alloys under different environmental conditions, such as in Ringer's solution [Citation93], in NaCl solution [Citation94] and in HCl solution [Citation95].
The most direct way to measure the extent of corrosion is via electrochemical experiments such as potentiodynamic polarisation curves. Cyclic potentiodynamic polarisation (CPP) curves directly measure the corrosion potential, , the corrosion current density,
and the corrosion rate. In Ti–6Al–4V, this measurement involves a three-electrode polarisation cell with Ti–6Al–4V as the working electrode, Ag/AgCl as the reference electrode, a Pt mesh counter electrode and the corrosion medium as the electrolyte. A more negative
value, a larger
value and a higher corrosion rate suggest a more corrosive environment. ASTM F2129-19a states that breakdown potentials of implant devices should be at least 800 mV within a physiological environment [Citation96]. Cyclic potentiodynamic polarisation measurements can accurately assess the extent of corrosion, however, this is limited to a stationary sample and not for corrosion wear or fretting corrosion [Citation97–99].
The protective TiO layer on the surface of Ti alloys can undergo dissolution, therefore resulting in localised corrosion (i.e. pitting corrosion) [Citation58]. This dissolution of TiO
layer occurs at high enough potential, termed critical pitting potential, which have been well documented to have a linear function of the logarithm of chloride ion concentration in metals [Citation100]. The mechanism of corrosion therefore occurs by adsorption of chloride ions into the metal substrate and subsequent dissolution reactions occurring at the metal/oxide interface [Citation101, Citation102]. Soltis [Citation103] has reviewed this mechanism and showed agreement between computational models and experiments in Ti alloys.
Sivakumar et al. [Citation104] found that the corrosion potential is also further influenced by kinetic parameters, and once fretting removes the passive oxide film on the implant surface, there is a potential difference which further increases corrosion rate. This is owing to the fretting contact being the anode and the large area outside the fretting zone being the cathode, the former increasing in area as fretting worsens.
Corrosion medium
There have been many attempts to determine the corrosion mechanism of Ti alloys in the presence of stress and exposure to the body fluid environment, such as by Dimah et al. [Citation105] using phosphate buffered solution (PBS), Yaya et al. [Citation106] using 0.9% NaCl solution, Cvijovic-Alagic et al. [Citation93] with Ringer's solution and Bidhendi and Pouranvari [Citation107] with Hank's solution. Since these solutions contain different concentrations of various chemical species, it is difficult to elucidate the exact mechanism of corrosion by comparing these studies. Table highlights a few studies involving corrosion of AM Ti alloys using a variety of corrosion media. Furthermore, these solutions are not similar to body fluid in composition. For a more systematic study of body fluid corrosion, a solution which has similar ionic concentrations to that of body fluid is crucial. Such solution was developed by Kokubo and Takadama [Citation108] in 2006, which has very similar ionic concentrations to blood plasma. The chemical composition of these solutions as well as human blood plasma is shown in Table .
Table 2. Typical chemical composition of human blood plasma [Citation112] and different physiological solutions [Citation108, Citation113].
It is important to note that body fluid compositions do not remain constant throughout and also vary from person to person [Citation97]. For example, studies involving pH of body fluid before and after a metal implant is placed in vivo have found that it can drop from 7.4 to 5.5 due to the disruption of blood supply to the bone [Citation109] while a bacterial infection post-surgery can result in a pH of between 4.0 and 9.0 in the vicinity of implant surface. A localised decrease in pH can result in severe pitting corrosion of the implant. This has also been verified in in vitro experiments [Citation110]. Moreover, oxygen content in blood is lower than that of artificial physiological solutions in air due to the presence of haemoglobin in blood, thus repassivation of implant surface, once corroded, is much more difficult in the body [Citation28, Citation111]. Meanwhile, carbon dioxide in blood will reduce its pH which accelerates corrosion.
Corrosion in AM lattices
There has been very few studies involving corrosion of additively manufactured Ti–6Al–4V lattices in the literature. Sharp et al. [Citation114] studied the effect of porosity on LPBF Ti–6Al–4V Gyroid structures and found out that a lower porosity lattice is more susceptible to pitting corrosion. This suggests a trade-off between lattice optimisation and corrosion behaviour and thus a potential link between void volume, surface area and corrosion. As expected, corrosion appeared to initiate at corners and raised edges, and breakdown potentials for all samples tested were above that found in a physiological environment showing that the implants should not corrode in the body. Losiewicz et al. [Citation115] investigated the severity of corrosion among different TPMS lattices and found that Gyroid lattices are most corrosion resistant. Gabay et al. [Citation116] studied AM Ti–6Al–4V lattice that is infiltrated with a biodegradable Zn–2%Fe alloy and determined adequate corrosion resistance, improved osseointegration and satisfactory bonding between the two materials.
The lifetime of orthopaedic implants containing AM lattices is therefore difficult to predict accurately. In addition to corrosion due to exposure to body fluid environment, the application of mechanical loading will likely reduce their overall lifetime. The next section aims to investigate the effect of fatigue in AM Ti alloys for orthopaedic implants.
Fatigue
Fatigue is well known to be the most common cause of premature orthopaedic implant failure [Citation117], where the application of cyclic stress leads to the initiation and growth of cracks. Upon reaching a critical crack length, catastrophic failure occurs [Citation118]. Fatigue properties of Ti alloys are well studied and are greatly influenced by microstructure types and grain sizes, with bimodal microstructures possessing the highest fatigue strength, followed by lamellar and equiaxed [Citation119]. In many materials including titanium alloys, fatigue cracks often initiate at stress concentration sites such as inclusions, pores, residual surface stresses and grain boundaries. In dual-phase alloys such as Ti–6Al–4V, the softer phase is more prone to fatigue crack nucleation compared to the harder phase [Citation120].
Corrosion fatigue
Corrosion fatigue is a well-known behaviour in alloys where failure occurs under cyclic loading when exposed to corrosive species [Citation121]. Typical fatigue crack initiation in Ti alloys occurs as a result of plastic deformation during cyclic loading, resulting in regions termed persistent slip bands [Citation122].
Ti alloys are generally considered to be corrosion resistant owing to its protective TiO film that spontaneously forms in air on its surface [Citation123]. However, while this oxide film is resistant to chemical attack and corrosive environments under static conditions, it is not sufficiently stable under loading conditions [Citation124] when exposed to body fluids [Citation125]. Corrosion fatigue behaviour of conventionally manufactured Ti alloys is relatively well studied, especially owing to its widespread use in aero-engine and other industrial applications [Citation126]. Dawson and Pelloux [Citation127] studied the different crack propagation behaviours in Ti–6Al–4V when immersed to different environments. Similarly, Baragetti and Arcieri [Citation128] found that Ti–6Al–4V exposed to 3.5% NaCl solution has a 20% reduction in stress concentration factors in fatigue tests compared to that exposed to laboratory air.
Vallittu and Kononen [Citation129] also found that crack propagation in Ti alloys under cyclic loading is relatively rapid compared to cobalt-chromium and gold alloys. In the field of metallic implants, pitting corrosion is common owing to the dissolution of protective passive film due to contact with aggressive species such as chloride ions [Citation117]. Fatigue cracks have been found to nucleate near these pits, which continue to grow until fracture occurs. Studies by Azevedo [Citation130] and Magnissalis et al. [Citation131] have shown that metallic implants have reduced fatigue lives and faster fatigue crack propagation due to corrosion.
Corrosion fatigue behaviour in Ti alloys is strongly influenced by microstructure. This has been studied by Bache and Evans [Citation132], who found that the lamellar microstructure is the most sensitive to environmental conditions, presenting with a significant increase in crack growth rates, as compared to bimodal and mill annealed microstructures. Similarly, Gregory [Citation133] found that corrosion fatigue behaviour is influenced by crystal orientation (texture), showing only significant reduction in fatigue life when the basal planes of the hexagonal α phase are perpendicular to tensile stress. In contrast, Roach et al. [Citation134] found that the presence of a notch, rather than environmental conditions, contributes more to a reduced fatigue lifetime of Ti alloys, due to higher tri-axial stress state at the notch.
It is important to note that to date, there have been no studies to determine the relationship between microstructure, surface finish and fatigue crack growth in Ti alloy implants under body fluid environments [Citation117], owing to the difficulty in replicating these conditions in laboratory conditions, i.e. physiological medium containing proteins, enzyme and ions at with varying wear and cyclic loading conditions.
Fatigue in AM lattices
Fatigue behaviour in AM components is notoriously difficult to predict and is highly dependent on the sample geometry, surface finish and defect concentration. Typical AM components contain porosity which generally reduce their overall fatigue strength compared to conventionally manufactured components of the same geometry [Citation135]. The fatigue resistance of Gyroid lattices has been extensively studied by Yang et al. [Citation136], who found that the internal strut topology of lattices play a crucial role in its fatigue resistance. Specifically, lattices such as Gyroid with helical surfaces and round pores are effective in reducing tensile stress around nodes driving crack initiation and propagation. Other studies have presented that fatigue resistance improves with lower internal defect percentage. Specifically, Kelly et al. [Citation137] found that void defects within struts are typical sites for crack initiation, whereas Mahmoud et al. [Citation138] found that thicker struts require more laser exposure, leading to worse heat accumulation and unstable melt pools. These yield internal defects thereby decreasing fatigue resistance. Similarly, Li et al. [Citation139] investigated the compression fatigue behaviour of lattices with high porosities (60–85%), showing that fatigue failure in highly porous lattices is mainly dominated by cyclic ratcheting leading to fatigue crack initiation and propagation. An improved fatigue strength is observed in lattices with higher relative density.
Burton et al. [Citation140] performed finite element analysis on different lattice geometries and found that the Schwartz primitive (pinched) lattice has the strongest unit cell, with a 10% volume fraction sample displaying good fatigue properties. Moreover, controlling the volume fraction the overall stiffness of the implant which can be tuned to match that of the surrounding bone. In fact, it has been widely hypothesised that having a slightly lower Young's modulus than bone is beneficial for bone ingrowth and having some implant deformation promotes better bone formation [Citation141, Citation142]. In the study of stochastic lattices, Mhurchadha et al. [Citation143] have found that strut thickness in Voronoi structures has a significant influence on fatigue lifetime.
Few studies have studied the combined effect of corrosion and fatigue in AM Ti–6Al–4V. Jesus et al. [Citation59] found that fatigue crack propagation rate increased by 290% in Ringer's solution and more than 330% in 3.5% NaCl solution in comparison to an air environment.
Premature failure from corrosion and fatigue is common in orthopaedic implants. Another common cause for early failure of implants is due to fretting/wear leading to the release of metal debris harmful to tissue health. The following section aims to investigate the effect of wear on AM Ti alloys for orthopaedic implants.
Wear
Ti alloys are known to have relatively poor wear resistance [Citation144]. This is largely due to the low protection of the surface oxide layers against mechanical wear; oxides of Ti easily spall and fragment [Citation145]. This is especially the case for Ti on Ti contact.
Orthopaedic implants, especially joint replacements, may contain bearing surfaces which may wear during its lifetime. Metal on metal bearing surfaces typically cause less wear over time compared to metal on polymer and ceramic on polymer surfaces [Citation146], hence are preferable for younger patients [Citation147]. However, metal wear debris and metal ion release are detrimental to human health [Citation148]. Furthermore, the release of metal ions due to tribocorrosion can also negatively affect the mechanical stability of the implants, causing implant loosening [Citation105]. An example of bone loss due to wear debris causing implant loosening is shown in Figure .
Figure 5. Bone loss surrounding a hip implant. (Reproduced from [Citation149].)
![Figure 5. Bone loss surrounding a hip implant. (Reproduced from [Citation149].)](/cms/asset/ca0371c0-2798-4a7a-b686-83ef0483c829/ymst_a_2230417_f0005_ob.jpg)
Corrosion wear
Tribocorrosion is the combined effect of interactions between corrosion and mechanical wear [Citation150]. Tribocorrosion studies usually perform wear tests with and without body fluid solutions, such as that following ASTM G77-83 using block-on-disc configuration [Citation93], using pin-on-plate tribometer [Citation151] or using ball-on-disc tribometer [Citation105]. These methods produce different wear tracks and are not directly comparable with each other.
In the case of Ti–6Al–4V, Dimah et al. [Citation105] have found that the protective oxide layer formed when exposed to body fluid solution consists of mainly TiO, with TiO and Ti
O
in the metal/TiO
interface and Al
O
in the TiO
/solution interface. Milosev et al. [Citation152] found that the wear resistance of the alloy therefore depends on the mechanical properties of the oxides on the surface layer. This is due to the slow regeneration of the passive film once damaged by wear [Citation93]. Wear of implant devices can lead to implant loosening as well as surrounding tissue inflammation due to wear debris, which is highly undesirable [Citation40].
Wear in AM lattices
Tribocorrosion studies in AM Ti–6Al–4V lattices are limited and have generally shown worse wear properties compared to conventionally manufactured Ti–6Al–4V. This is mainly owing to the increased surface roughness in AM Ti–6Al–4V components. The inherent irregularities on the surface of AM components are due to the solidification of the melt pool, leading to partially melted powder particles [Citation153] on the component surface, which are in the range of 10–60 μm in diameter for SLM powders and 50–150 μm for EBM powders [Citation154]. Furthermore, the layer-by-layer deposition in AM causes surface defects such as the stair-step effect [Citation155]. Both these factors contribute to poorer tribocorrosion properties as surface irregularities can act as initiation sites for pitting corrosion [Citation156].
Interestingly, Shahsavari et al. [Citation154] found that corrosion resistance in AM Ti–6Al–4V is improved with decreasing surface roughness, which can be achieved using techniques such as electron beam surface remelting. However, this may be undesirable as a decreased surface roughness will be detrimental in aiding bone ingrowth and decrease bone adhesion.
Furthermore, tribocorrosion studies are only applicable in cases where the Ti implant has an articulating surface such as to replace ball-and-socket joints. However, where articulating surfaces are required, orthopaedic implants are often highly polished to remove surface roughness and coated with a ceramic layer such as titanium nitride (TiN) which has very high wear resistance, hardness and smoothness [Citation32]. Where articulating surfaces are not required, corrosion wear is unlikely to occur as there is no friction experienced by the implant. In these cases, bone fusion is a more significant issue to prevent implant loosening [Citation157], hence a porous implant such as open-cell lattices is beneficial. The pore size and shape can be optimised by varying the geometry of the lattice to ensure maximised bone ingrowth and adhesion to the implant [Citation72].
To improve tribocorrosion properties in AM Ti alloys, many studies have investigated various surface finishing methods, such as sandblasting [Citation158], chemical etching [Citation159], passivation and electropolishing [Citation64]. For example, adding diamond like coating (DLC) on the surface of implants has been found to improve the overall wear resistance, as well as encouraging cell growth [Citation146]. However, it is important to note that for orthopaedic implant applications, increased surface roughness is favourable for bone ingrowth to prevent loosening.
Discussion
Additive manufacturing inherently produces components with more defects than conventional manufacturing owing to its high cooling rate [Citation160], high residual stresses [Citation161], porosity [Citation162] and surface roughness from partially melted metal powders [Citation155]. As such, AM implants are more susceptible to localised corrosion as well as tribocorrosion [Citation53]. Subsequently, metal ion release to body fluid is a safety concern as these can cause toxic and allergic symptoms [Citation163]. In Ti–6Al–4V implants, there is a preferential release of Ti ions but also significant amounts of Al and a small amount of V ions, the latter being a concern as it is toxic to humans [Citation164].
The fatigue properties of AM lattices are challenging to predict due to its complicated geometries. This is in comparison with conventionally manufactured Ti implants for which corrosion fatigue behaviours have been extensively studied. In AM lattices, modelling fatigue crack growth is tricky as there are significant variations in strut surfaces between manufactured components and CAD data [Citation87]. Consequently, predicting the fatigue behaviour of stochastic AM lattices is even more difficult, however, some studies have attempted this and compared their results with experimental data [Citation88] with good consistency. Even so, AM artefacts such as residual stress and surface roughness are known to reduce the fatigue life of AM components. Under corrosive environments such as body fluid, fatigue life of these lattices is expected to be further reduced [Citation133], although the extent of this remains a gap in literature that needs to further studied.
In terms of wear, Chiu et al. [Citation165] have investigated and compared the performance of both conventionally manufactured and AM Ti–6Al–4V and showed worse corrosion wear behaviour in the AM specimen. This has been attributed to microstructure defects and porosity on the AM specimen causing an enrichment in oxygen vacancies on the surface layer when exposed to body fluid solution. Interestingly, the formation of passivating TiO layer on the surface of AM Ti–6Al–4V has been found to be faster in specimens with a finer grain structure. This suggests that the corrosion wear behaviour in AM Ti alloys can be improved through specific heat treatment cycles aimed at grain refinement.
Future prospects
The demand for orthopaedic implants is ever-increasing for a variety of medical needs. While the corrosion, fatigue and wear behaviour of AM implants are challenging to predict, this paper has shown the breadth of experiments conducted in the literature to determine these properties in AM implants, ensuring they are safe for clinical use. With its inherent defects and complicated geometries, the lifetime of AM implant devices might be reduced. However, with its lattice geometries, the devices allow for faster bone ingrowth, ensuring that the surrounding bone can quickly bear load thus reducing the reliance on implant devices themselves.
AM of orthopaedic implants will also continue to be the lucrative option especially in specialised cases such as oncology and paediatric cases, where commercially available modular implants are not appropriate and limb salvage is a priority [Citation48]. Furthermore, revision surgeries for patients with bone loss surrounding existing implant devices require custom-shaped implants which is only possible with AM [Citation166].
Conclusion
The development of AM technology allows for the fabrication of complex implant geometries, opening new opportunities in the field of custom-made implants. This paper reviews the corrosion, fatigue and wear behaviour of Ti alloys for orthopaedic implants fabricated by additive manufacturing.
AM lattice geometries enable implants to have mechanical properties that are similar to surrounding bone while allowing bone ingrowth, both of which are desirable for implant durability.
There are limited studies on the behaviour of these lattices under both mechanical loading and body fluid environment. However, due to fast cooling rates and poor surface finish in AM components, it is expected that both corrosion fatigue and wear properties of AM Ti–6Al–4V orthopaedic implants to be worse.
There is therefore a trade-off between osseointegration of implant and surrounding bone and their long-term mechanical and corrosion properties.
Overall, this opens up possibilities for research in the promising area of AM lattice geometry optimisation for optimum mechanical properties, enhanced osseointegration and corrosion resistance.
Disclosure statement
No potential conflict of interest was reported by the author(s).
Additional information
Funding
References
- Elias CNN, Lima JH, Valiev R, et al. Biomedical applications of titanium and its alloys. Jom. 2008;60(3):46–49. doi: 10.1007/s11837-008-0031-1
- Hao Y, Li S, Yang R. Biomedical titanium alloys and their additive manufacturing. Rare Met. 2016;35(9):661–671. doi: 10.1007/s12598-016-0793-5
- Navarro M, Michiardi A, Castaño O, et al. Biomaterials in orthopaedics. J R Soc Interface. 2008;5(27):1137–1158. doi: 10.1098/rsif.2008.0151
- Lee DH, Reasoner K, Stewart A. From concept to counter: a review of bringing an orthopaedic implant to market. J Am Acad Orthop Surg. 2020;28(14):e604–e611. doi: 10.5435/JAAOS-D-20-00017
- Iorio R, Robb WJ, Healy WL, et al. Orthopaedic surgeon workforce and volume assessment for total hip and knee replacement in the United States: preparing for an epidemic. J Bone Jt Surg. 2008;90(7):1598–1605. doi: 10.2106/JBJS.H.00067
- Crowninshield RD, Rosenberg AG, Sporer SM. Changing demographics of patients with total joint replacement. Clin Orthop Relat Res. 2006;443(443):266–272. doi: 10.1097/01.blo.0000188066.01833.4f
- C. f. D. C. (CDC) and Prevention, Prevalence of Self-Reported Arthritis or Chronic Joint Symptoms Among Adults–United States, 2001, 2002.
- Barba D, Reed RC, Alabort E. Design of metallic lattices for bone implants by additive manufacturing. Minerals, Metals and Materials Series. 2020:745–759. doi: 10.1007/978-3-030-36296-6_69
- Ricles LM, Coburn JC, Prima MD, et al. Regulating 3D-printed medical products. Sci Transl Med. 2018;6521:1–7. doi: 10.1126/scitranslmed.aan6521
- Tsai PI, Lam TN, Wu MH, et al. Multi-scale mapping for collagen-regulated mineralization in bone remodeling of additive manufacturing porous implants. Mater Chem Phys. 2019;230:83–92. doi: 10.1016/j.matchemphys.2019.03.047
- Bittredge O, Hassanin H, El-Sayed MA, et al. Fabrication and optimisation of Ti–6Al–4V lattice-structured total shoulder implants using laser additive manufacturing. Materials. 2022;15(9):3095. doi: 10.3390/ma15093095
- Hassanin H, Al-Kinani AA, Elshaer A, et al. Stainless steel with tailored porosity using canister-free hot isostatic pressing for improved osseointegration implants. J Mater Chem B. 2017;5(47):9384–9394. doi: 10.1039/C7TB02444D
- Benady A, Meyer SJ, Golden E, et al. Patient-specific Ti–6Al–4V lattice implants for critical-sized load-bearing bone defects reconstruction. Mater Des. 2023;226:111605. doi: 10.1016/j.matdes.2023.111605
- Boileau P, Melis B, Duperron D, et al. Revision surgery of reverse shoulder arthroplasty. J Shoulder Elbow Surg. 2013;22(10):1359–1370. doi: 10.1016/j.jse.2013.02.004
- Ramhamadany E, Chadwick C, Davies MB. Treatment of severe avascular necrosis of the talus using a novel keystone-shaped 3D-printed titanium truss implant. Foot Ankle Orthop. 2021;6(4):1–9. doi: 10.1177/24730114211043516
- Nwankwo EC, Chen F, Nettles DL, et al. Five-year follow-up of distal tibia bone and foot and ankle trauma treated with a 3D-Printed titanium cage. Case Rep Orthop. 2019;2019:1–6. doi: 10.1155/2019/7571013
- Mulfinger GL, Trueta J. The blood supply of the talus.. J Bone Jt Surg. 1970;52-B(1):160–167. doi: 10.1302/0301-620X.52B1.160
- Kuslich SD, Danielson G, Dowdle JD, et al. Four-year follow-up result of lumbar spine arthrodesis using the Bagby and Kuslich lumbar fusion age. Spine. 2000;25(20):2656–2662. doi: 10.1097/00007632-200010150-00018
- Popov VV, Muller-Kamskii G, Katz-Demyanetz A, et al. Additive manufacturing to veterinary practice: recovery of bony defects after the osteosarcoma resection in canines. Biomed Eng Lett. 2019;9(1):97–108. doi: 10.1007/s13534-018-00092-7
- Mangano F, Chambrone L, Van Noort R, et al. Direct metal laser sintering titanium dental implants: A review of the current literature. International Journal of Biomaterials, vol. 2014, 2014.
- Dabrowski B, Swieszkowski W, Godlinski D, et al. Highly porous titanium scaffolds for orthopaedic applications. J Biomed Mater Res B Appl Biomater. 2010;95B(1):53–61. doi: 10.1002/jbm.b.31682
- Mangano C, Raspanti M, Traini T, et al. Stereo imaging and cytocompatibility of a model dental implant surface formed by direct laser fabrication. J Biomed Mater Res A. 2009;88A(3):823–831. doi: 10.1002/jbm.a.v88a:3
- Kirkpatrick JS, Stevens T. The FDA process for the evaluation and approval of orthopaedic devices. J Am Acad Orthop Surg. 2008;16(5):260–267. doi: 10.5435/00124635-200805000-00004
- Sharma S, Lammin K, Kay P. Introduction and follow-up of new implants. Orthop Trauma. 2012;26(4):231–236. doi: 10.1016/j.mporth.2012.08.002
- Skinner JA, Kay PR, Hart AJ. Introducing new joint replacements to clinical practice. BMJ. 2012;344(7839):2011–2012. doi: 10.1136/bmj.d8188
- Otero JJ, Vijverman A, Mommaerts MY. Use of fused deposit modeling for additive manufacturing in hospital facilities: European certification directives. J Craniomaxillofac Surg. 2017;45(9):1542–1546. doi: 10.1016/j.jcms.2017.06.018
- Garot C, Bettega G, Picart C. Additive manufacturing of material scaffolds for bone regeneration: toward application in the clinics. Adv Funct Mater. 2021;31(5):2006967. doi: 10.1002/adfm.v31.5.
- Morita M, Sasada T, Hayashi H, et al. The corrosion fatigue properties of surgical implants in a living body. J Biomed Mater Res. 1988;22(6):529–540. doi: 10.1002/(ISSN)1097-4636
- Khan MA, Williams RL, Williams DF. Conjoint corrosion and wear in titanium alloys. Biomaterials. 1999;20(8):765–772. doi: 10.1016/S0142-9612(98)00229-4
- Okazaki Y. Effect of friction on anodic polarization properties of metallic biomaterials. Biomaterials. 2002;23(9):2071–2077. doi: 10.1016/S0142-9612(01)00337-4
- Qu J, Blau PJ, Watkins TR, et al. Friction and wear of titanium alloys sliding against metal, polymer, and ceramic counterfaces. Wear. 2005;258(9):1348–1356. doi: 10.1016/j.wear.2004.09.062
- Monticelli C, Zucchi F, Tampieri A. Triboelectrochemical behaviour of a Si3N4-TiN ceramic composite and a titanium alloy commonly used in biomedical applications. Wear. 2009;266(1–2):327–336. doi: 10.1016/j.wear.2008.07.005
- Gilbert JL, Buckley CA, Jacobs JJ. In vivo corrosion of modular hip prosthesis components in mixed and similar metal combinations. the effect of crevice, stress, motion, and alloy coupling. J Biomed Mater Res. 1993;27(12):1533–1544. doi: 10.1002/(ISSN)1097-4636
- Wang X, Xu S, Zhou S, et al. Topological design and additive manufacturing of porous metals for bone scaffolds and orthopaedic implants: a review. Biomaterials. 2016;83:127–141. doi: 10.1016/j.biomaterials.2016.01.012
- Yuan L, Ding S, Wen C. Additive manufacturing technology for porous metal implant applications and triple minimal surface structures: a review. Bioact Mater. 2019;4:56–70. doi: 10.1016/j.bioactmat.2018.12.003
- Teoh SH. Fatigue of biomaterials: a review. Int J Fatigue. 2000;22(10):825–837. doi: 10.1016/S0142-1123(00)00052-9
- Chandrasekaran M. 10 – Forging of metals and alloys for biomedical applications. 2nd ed., In: Metals for Biomedical Devices. Woodhead Publishing Series in Biomaterials, 2019. p. 293–310.
- Lange K, Pohlandi K, Raghupathi PS, et al. Handbook of metal forming. Columbus: McGraw-Hill; 1985.
- Murr LE, Gaytan SM, Martinez E, et al. Next generation orthopaedic implants by additive manufacturing using electron beam melting. International Journal of Biomaterials, vol. 2012, 2012.
- Pal B, Puthumanapully PK, Amis AA. (ii) biomechanics of implant fixation. Orthop Trauma. 2013;27(2):76–84. doi: 10.1016/j.mporth.2013.02.004
- Beckenbaugh RD, Ilstrup DM. Total hip arthroplasty. J Bone Jt Surg. 1978;60(3):306–313. doi: 10.2106/00004623-197860030-00005
- Mroczkowski ML, Hertzler JS, Humphrey SM, et al. Effect of impact assembly on the fretting corrosion of modular hip tapers. J Orthop Res. 2006;24(2):271–279. doi: 10.1002/(ISSN)1554-527X
- Esfahani EA, Bukuaghangin O, Banfield S, et al. Surface engineering of wrought and additive layer manufactured Ti–6Al–4V alloy for enhanced load bearing and bio-tribocorrosion applications. Surf Coat Technol. 2022;442:128139. doi: 10.1016/j.surfcoat.2022.128139.
- Bagaria V, Deshpande S, Rasalkar DD, et al. Use of rapid prototyping and three-dimensional reconstruction modeling in the management of complex fractures. Eur J Radiol. 2011;80(3):814–820. doi: 10.1016/j.ejrad.2010.10.007
- Holzapfel BM, Reichert JC, Schantz JT, et al. How smart do biomaterials need to be? A translational science and clinical point of view. Adv Drug Deliv Rev. 2013;65(4):581–603. doi: 10.1016/j.addr.2012.07.009
- Rajasekharan AK, Lotsari A, Lutz-Bueno V, et al. Bioinspired structural hierarchy within macroscopic volumes of synthetic composites. Adv Healthc Mater. 2018;7(18):1–7. doi: 10.1002/adhm.v7.18
- Krut'ko VK, Kazbanov VV, Musskaya ON, et al. Physicochemical properties and structure of the bone matrix in simulated tuberculous osteitis. Tech Phys. 2019;64(1):121–126. doi: 10.1134/S1063784219010183
- Park JW, Kang HG. Application of 3-dimensional printing implants for bone tumors. Clin Exp Pediatr. 2022;65(10):476–482. doi: 10.3345/cep.2021.01326
- Gai X, Bai Y, Li J, et al. Electrochemical behaviour of passive film formed on the surface of Ti–6Al–4V alloys fabricated by electron beam melting. Corros Sci. 2018;145:80–89. doi: 10.1016/j.corsci.2018.09.010
- Dai N, Zhang LC, Zhang J, et al. Corrosion behavior of selective laser melted Ti–6Al–4 V alloy in NaCl solution. Corros Sci. 2016;102:484–489. doi: 10.1016/j.corsci.2015.10.041
- Leon A, Levy GK, Ron T, et al. The effect of strain rate on stress corrosion performance of Ti6Al4V alloy produced by additive manufacturing process. J Mater Res Technol. 2020;9(3):4097–4105. doi: 10.1016/j.jmrt.2020.02.035
- Sharma A, Oh MC, Kim JT, et al. Investigation of electrochemical corrosion behavior of additive manufactured Ti–6Al–4V alloy for medical implants in different electrolytes. J Alloys Compd. 2020;830:154620. doi: 10.1016/j.jallcom.2020.154620
- Zhao B, Wang H, Qiao N, et al. Corrosion resistance characteristics of a Ti–6Al–4V alloy scaffold that is fabricated by electron beam melting and selective laser melting for implantation in vivo. Mater Sci Eng C. 2017;70:832–841. doi: 10.1016/j.msec.2016.07.045
- Fojt J, Fousova M, Jablonska E, et al. Corrosion behaviour and cell interaction of Ti–6Al–4V alloy prepared by two techniques of 3D printing. Mater Sci Eng C. 2018;93:911–920. doi: 10.1016/j.msec.2018.08.066
- Koike M, Greer P, Owen K, et al. Evaluation of titanium alloys fabricated using rapid prototyping technologies-electron beam melting and laser beam melting. Materials. 2011;4(10):1776–1792. doi: 10.3390/ma4101776
- Metalnikov P, Ben-Hamu G, Eliezer D. Corrosion behavior of AM-Ti–6Al–4V: a comparison between EBM and SLM. Prog Addit Manuf. 2022;7(3):509–520. doi: 10.1007/s40964-022-00293-8
- Ettefagh AH, Zeng C, Guo S, et al. Corrosion behavior of additively manufactured Ti–6Al–4V parts and the effect of post annealing. Addit Manuf. 2019;28:252–258. doi: 10.1016/j.addma.2019.05.011
- Yang J, Yang H, Yu H, et al. Corrosion behavior of additive manufactured Ti–6Al–4V alloy in NaCl solution. Metall Mater Trans A Phys Metall Mater Sci. 2017;48(7):3583–3593. doi: 10.1007/s11661-017-4087-9
- Jesus JS, Borrego LP, Ferreira JAM, et al. Fatigue crack growth under corrosive environments of Ti–6Al–4V specimens produced by SLM. Eng Fail Anal. 2020;118:104852. doi: 10.1016/j.engfailanal.2020.104852
- Chen L, Zhang HY, Zheng C, et al. Corrosion behavior and characteristics of passive films of laser powder bed fusion produced Ti–6Al–4V in dynamic Hank's solution. Mater Des. 2021;208:109907. doi: 10.1016/j.matdes.2021.109907
- Gayathri YKB, Kumar RL, Ramalingam VV, et al. Additive manufacturing of Ti–6Al–4V alloy for biomedical applications. J Bio- Tribo-Corros. 2022;8(98):1–20. doi: 10.1007/s40735-022-00700-1
- Bai Y, Gai X, Li S, et al. Improved corrosion behaviour of electron beam melted Ti–6Al–4V alloy in phosphate buffered saline. Corros Sci. 2017;123:289–296. doi: 10.1016/j.corsci.2017.05.003
- Lu P, Wu M, Liu X, et al. Study on corrosion resistance and bio-tribological behavior of porous structure based on the SLM manufactured medical Ti6Al4V. Met Mater Int. 2020;26(8):1182–1191. doi: 10.1007/s12540-019-00506-w
- Wu Y, Kuo C, Chung Y, et al. Effects of electropolishing on mechanical properties beam melting additive manufacturing. Materials. 2019;12(9):1466. doi: 10.3390/ma12091466
- Wegner N, Kotzem D, Wessarges Y, et al. Corrosion and corrosion fatigue properties of additively manufactured magnesium alloy WE43 in comparison to titanium alloy Ti–6Al–4V in physiological environment. Materials. 2019;12(18):2892. doi: 10.3390/ma12182892
- Hawreliak JA, Lind J, Maddox B, et al. Dynamic behavior of engineered lattice materials. Sci Rep. 2016;6:1–7. doi: 10.1038/srep28094
- Lozanovski B, Downing D, Tino R, et al. Non-destructive simulation of node defects in additively manufactured lattice structures. Addit Manuf. 2020;36:101593. doi:10.1016/j.addma.2020.101593
- Syam WP, Jianwei W, Zhao B, et al. Design and analysis of strut-based lattice structures for vibration isolation. Precis Eng. 2018;52:494–506. doi: 10.1016/j.precisioneng.2017.09.010
- Britt C, Montgomery CJ, Brand MJ, et al. Effect of processing parameters and strut dimensions on the microstructures and hardness of stainless steel 316L lattice-emulating structures made by powder bed fusion. Addit Manuf. 2021;40:101943. doi: 10.1016/j.addma.2021.101943
- Sereshk MRV, Triplett K, St John C, et al. A computational and experimental investigation into mechanical characterizations of strut-based lattice structures. Solid Freeform Fabrication 2019: Proceedings of the 30th Annual International Solid Freeform Fabrication Symposium – An Additive Manufacturing Conference, SFF 2019, 2019. p. 2196–2207.
- Dallago M, Raghavendra S, Luchin V, et al. The role of node fillet, unit-cell size and strut orientation on the fatigue strength of Ti–6Al–4V lattice materials additively manufactured via laser powder bed fusion. Int J Fatigue. 2021;142:105946. doi: 10.1016/j.ijfatigue.2020.105946
- Van Bael S, Chai YC, Truscello S, et al. The effect of pore geometry on the in vitro biological behavior of human periosteum-derived cells seeded on selective laser-melted Ti6Al4V bone scaffolds. Acta Biomater. 2012;8(7):2824–2834. doi: 10.1016/j.actbio.2012.04.001
- Zadpoor AA. Bone tissue regeneration: the role of scaffold geometry. Biomater Sci. 2015;3(2):231–245. doi: 10.1039/C4BM00291A
- Schoen AH. Infinite periodic minimal surfaces without self-intersections. NASA Tech Note. 1970;1970002047:191–210. doi: 10.1057/9781137465160.0020
- Hyde S, Blum Z, Landh T, et al. The language of shape. 1 ed., Amsterdam: Elsevier Science; 1997.
- Lord EA, Mackay AL. Periodic minimal surfaces of cubic symmetry. Curr Sci. 2003;85(3):346–362.
- Kapfer SC, Hyde ST, Mecke K, et al. Minimal surface scaffold designs for tissue engineering. Biomaterials. 2011;32(29):6875–6882. doi: 10.1016/j.biomaterials.2011.06.012
- Lehder EF, Ashcroft IA, Wildman RD, et al. A multiscale optimisation method for bone growth scaffolds based on triply periodic minimal surfaces. Biomech Model Mechanobiol. 2021;20(6):2085–2096. doi: 10.1007/s10237-021-01496-8
- Karageorgiou V, Kaplan D. Porosity of 3D biomaterial scaffolds and osteogenesis. Biomaterials. 2005;26(27):5474–5491. doi: 10.1016/j.biomaterials.2005.02.002
- Götz HE, Müller M, Emmel A, et al. Effect of surface finish on the osseointegration of laser-treated titanium alloy implants. Biomaterials. 2004;25(18):4057–4064. doi: 10.1016/j.biomaterials.2003.11.002
- Knychala J, Bouropoulos N, Catt CJ, et al. Pore geometry regulates early stage human bone marrow cell tissue formation and organisation. Ann Biomed Eng. 2013;41(5):917–930. doi: 10.1007/s10439-013-0748-z
- Bidan CM, Kommareddy KP, Rumpler M, et al. How linear tension converts to curvature: geometric control of bone tissue growth. PLoS ONE. 2012;7(5):e36336. doi: 10.1371/journal.pone.0036336
- Ambrosi D, Guillou A. Growth and dissipation in biological tissues. Contin Mech Thermodyn. 2007;19(5):245–251. doi: 10.1007/s00161-007-0052-y
- Ambrosi D, Guana F. Stress-modulated growth. Math Mech Solids. 2007;12(3):319–342. doi: 10.1177/1081286505059739
- Niinomi M, Liu Y, Nakai M, et al. Biomedical titanium alloys with Young's moduli close to that of cortical bone. Regen Biomater. 2016;3(3):173–185. doi: 10.1093/rb/rbw016
- Fantini M, Curto M, De Crescenzio F. A method to design biomimetic scaffolds for bone tissue engineering based on voronoi lattices. Virtual Phys Prototyp. 2016;11(2):77–90. doi: 10.1080/17452759.2016.1172301
- Ghouse S, Babu S, Nai K, et al. The influence of laser parameters, scanning strategies and material on the fatigue strength of a stochastic porous structure. Addit Manuf. 2018;22:290–301. doi: 10.1016/j.addma.2018.05.024
- Munford M, Hossain U, Ghouse S, et al. Prediction of anisotropic mechanical properties for lattice structures. Addit Manuf. 2020;32:101041. doi: 10.1016/j.addma.2020.101041
- Ashby MF. The properties of foams and lattices. Philos Trans Royal Soc A Math Phys Eng Sci. 2006;364(1838):15–30. doi: 10.1098/rsta.2005.1678
- Yan C, Hao L, Hussein A, et al. Advanced lightweight 316L stainless steel cellular lattice structures fabricated via selective laser melting. Mater Des. 2014;55:533–541. doi: 10.1016/j.matdes.2013.10.027
- Yang X, Du C, Wan H, et al. Influence of sulfides on the passivation behavior of titanium alloy TA2 in simulated seawater environments. Appl Surf Sci. 2018;458:198–209. doi: 10.1016/j.apsusc.2018.07.068
- Perini N, Corradini PG, Nascimento VP, et al. Characterization of AISI 1005 corrosion films grown under cyclic voltammetry of low sulfide ion concentrations. Corros Sci. 2013;74:214–222. doi: 10.1016/j.corsci.2013.04.045
- Cvijović-Alagić I, Cvijović Z, Mitrović S, et al. Wear and corrosion behaviour of Ti–13Nb–13Zr and Ti–6Al–4V alloys in simulated physiological solution. Corros Sci. 2011;53(2):796–808. doi: 10.1016/j.corsci.2010.11.014
- Abou Shahba RM, Ghannem WA, El-Shenawy AES, et al. Corrosion and inhibition of Ti–6Al–4V alloy in NaCl solution. Int J Electrochem Sci. 2011;6(11):5499–5509.
- Atapour M, Pilchak A, Frankel GS, et al. Corrosion behavior of Ti–6Al–4V with different thermomechanical treatments and microstructures. Corrosion. 2010;66(6):065004–065004-9. doi: 10.5006/1.3452400
- ASTM International, ASTM-F2129-19a: Standard Test Method for Conducting Cyclic Potentiodynamic Polarisation Measurements to Determine the Corrosion Susceptibility of Small Implant Devices. tech. rep., West Conshohocken, PA, USA: ASTM International; 2019..
- Eliaz N. Corrosion of metallic biomaterials: a review. Materials. 2019;12(3):407. doi: 10.3390/ma12030407
- Souza JCM, Apaza-Bedoya K, Benfatti CA, et al. A comprehensive review on the corrosion pathways of titanium dental implants and their biological adverse effects. Metals. 2020;10(9):1–14. doi: 10.3390/met10091272
- Dini C, Costa RC, Sukotjo C, et al. Progression of bio-tribocorrosion in implant dentistry. Front Mech Eng. 2020;6:1–14. doi: 10.3389/fmech.2020.00001
- MacDonald DD. The history of the point defect model for the passive state: a brief review of film growth aspects. Electrochim Acta. 2011;56(4):1761–1772. doi: 10.1016/j.electacta.2010.11.005
- Metikoš-Huković M, Kwokal A, Piljac J. The influence of niobium and vanadium on passivity of titanium-based implants in physiological solution. Biomaterials. 2003;24(21):3765–3775. doi: 10.1016/S0142-9612(03)00252-7
- McCafferty E. Sequence of steps in the pitting of aluminum by chloride ions. Corros Sci. 2003;45(7):1421–1438. doi: 10.1016/S0010-938X(02)00231-7
- Soltis J. Passivity breakdown, pit initiation and propagation of pits in metallic materials – review. Corros Sci. 2015;90:5–22. doi: 10.1016/j.corsci.2014.10.006
- Sivakumar B, Pathak LC, Singh R. Role of surface roughness on corrosion and fretting corrosion behaviour of commercially pure titanium in Ringer's solution for bio-implant application. Appl Surf Sci. 2017;401:385–398. doi: 10.1016/j.apsusc.2017.01.033
- Dimah MK, Devesa Albeza F, Amigó Borrás V, et al. Study of the biotribocorrosion behaviour of titanium biomedical alloys in simulated body fluids by electrochemical techniques. Wear. 2012;294-295:409–418. doi: 10.1016/j.wear.2012.04.014
- Yaya K, Khelfaoui Y, Malki B, et al. Numerical simulations study of the localized corrosion resistance of AISI 316L stainless steel and pure titanium in a simulated body fluid environment. Corros Sci. 2011;53(10):3309–3314. doi: 10.1016/j.corsci.2011.06.006
- Bidhendi HRA, Pouranvari M. Corrosion study of metallic biomaterials in simulated body fluid. Metalurgija J Metall. 2011;17(1):13–22. doi: 10.30544/384
- Kokubo T, Takadama H. How useful is SBF in predicting in vivo bone bioactivity?. Biomaterials. 2006;27(15):2907–2915. doi: 10.1016/j.biomaterials.2006.01.017
- Winter GD. Tissue reaction to metallic wear and corrosion products in human patients. J Biomed Mater Res. 1974;8(3):11–26. doi: 10.1002/(ISSN)1097-4636
- Ciolac S, Vasilescu E, Drob P, et al. Long term in vitro investigation of titanium and some titanium alloys used for surgery implants. Rev Chim Buchar Orig Ed. 2000;51(1):36–41.
- Hughes AN, Jordan BA, Orman S. The corrosion fatigue properties of surgical implant materials. third progress report: May 1973. Eng Med. 1978;7(3):135–141. doi: 10.1243/EMED_JOUR_1978_007_036_02
- Oyane A, Kim HM, Furuya T, et al. Preparation and assessment of revised simulated body fluids. J Biomed Mater Res A. 2003;65A(2):188–195. doi: 10.1002/(ISSN)1097-4636
- Hansen AW, Führ LT, Antonini LM, et al. The electrochemical behavior of the NiTi alloy in different simulated body fluids. Mater Res. 2015;18(1):184–190. doi: 10.1590/1516-1439.305614
- Sharp R, Pelletier MH, Walsh WR, et al. Corrosion resistance of 3D printed Ti6Al4V gyroid lattices with varying porosity. Materials. 2022;15(14):4805. doi: 10.3390/ma15144805
- Łosiewicz B, Maszybrocka J, Kubisztal J, et al. Corrosion resistance of the cpti g2 cellular lattice with tpms architecture for gas diffusion electrodes. Materials. 2021;14(1):1–18. doi: 10.3390/ma14010081
- Gabay N, Ron T, Vago R, et al. Evaluating the prospects of Ti-base lattice infiltrated with biodegradable Zn-2%Fe alloy as a structural material for osseointegrated implants-in vitro study. Materials. 2021;14(16):4682. doi: 10.3390/ma14164682
- Antunes RA, De Oliveira MCL. Corrosion fatigue of biomedical metallic alloys: mechanisms and mitigation. Acta Biomater. 2012;8(3):937–962. doi: 10.1016/j.actbio.2011.09.012
- Gagg CR, Lewis PR. In-service fatigue failure of engineered products and structures – case study review. Eng Fail Anal. 2009;16(6):1775–1793. doi: 10.1016/j.engfailanal.2008.08.008
- Wu GQ, Shi CL, Sha W, et al. Effect of microstructure on the fatigue properties of Ti–6Al–4V titanium alloys. Mater Des. 2013;46:668–674. doi: 10.1016/j.matdes.2012.10.059
- Chan KS. Roles of microstructure in fatigue crack initiation. Int J Fatigue. 2010;32(9):1428–1447. doi: 10.1016/j.ijfatigue.2009.10.005
- Meyn DA. An analysis of frequency and amplitude effects on corrosion fatigue crack propagation in Ti–8Al–1Mo–1V. Metall Trans. 1971;2(3):853–865. doi: 10.1007/BF02662746
- Suresh S. Fatigue of materials. Cambridge University Press; 1998.
- Zavanelli RA, Henriques GEP, Ferreira I, et al. Corrosion-fatigue life of commercially pure titanium and Ti–6Al–4V alloys in different storage environments. J Prosthet Dent. 2000;84(3):274–279. doi: 10.1067/mpr.2000.108758
- Lucas LC, Lemons JE. Biodegradation of restorative metallic systems. Adv Dent Res. 1992;6:32–37. doi: 10.1177/08959374920060011301
- Rodrigues DC, Urban RM, Jacobs JJ, et al. In vivo severe corrosion and hydrogen embrittlement of retrieved modular body titanium alloy hip-implants. J Biomed Mater Res B Appl Biomater. 2009;88B(1):206–219. doi: 10.1002/jbm.b.v88b:1
- Baragetti S. Notch corrosion fatigue behavior of Ti–6Al–4V. Materials. 2014;7(6):4349–4366. doi: 10.3390/ma7064349
- Dawson DB, Pelloux RM. Corrosion fatigue crack growth of titanium alloys in aqueous environments. Metall Trans. 1974;5(3):723–731. doi: 10.1007/BF02644669
- Baragetti S, Arcieri EV. Corrosion fatigue behavior of Ti–6Al–4V: chemical and mechanical driving forces. Int J Fatigue. 2018;112:301–307. doi: 10.1016/j.ijfatigue.2018.02.033
- Vallittu PK, Kokkonen M. Deflection fatigue of cobalt-chromium, titanium, and gold alloy cast denture clasp. J Prosthet Dent. 1995;74(4):412–419. doi: 10.1016/S0022-3913(05)80384-1
- Azevedo CR. Failure analysis of a commercially pure titanium plate for osteosynthesis. Eng Fail Anal. 2003;10(2):153–164. doi: 10.1016/S1350-6307(02)00067-5
- Magnissalis EA, Zinelis S, Karachalios T, et al. Failure analysis of two Ti-alloy total hip arthroplasty femoral stems fractured in vivo. J Biomed Mater Res B Appl Biomater. 2003;66B(1):299–305. doi: 10.1002/(ISSN)1097-4636
- Bache MR, Evans WJ. The fatigue crack propagation resistance of Ti–6Al–4V under aqueous saline environments. Int J Fatigue. 2001;23(SUPPL. 1):319–323. doi: 10.1016/S0142-1123(01)00141-4
- Gregory JK. Corrosion-fatigue crack growth in titanium alloys. Mater Corros. 1999;50(1):7–11. doi: 10.1002/(ISSN)1521-4176
- Roach MD, Williamson RS, Zardiackas LD. Comparison of the corrosion fatigue characteristics of CP Ti-Grade 4, Ti–6Al–4V ELI, Ti–6Al–7Nb, and Ti–15Mo. ASTM Compass. 2005;2(7):1–19. doi: 10.1520/JAI12786
- Leuders S, Thöne M, Riemer A, et al. On the mechanical behaviour of titanium alloy TiAl6V4 manufactured by selective laser melting: fatigue resistance and crack growth performance. Int J Fatigue. 2013;48:300–307. doi: 10.1016/j.ijfatigue.2012.11.011
- Yang L, Wu S, Yan C, et al. Fatigue properties of Ti–6Al–4V gyroid graded lattice structures fabricated by laser powder bed fusion with lateral loading. Addit Manuf. 2021;46:102214. doi: 10.1016/j.addma.2021.102214
- Kelly CN, Francovich J, Julmi S, et al. Fatigue behavior of as-built selective laser melted titanium scaffolds with sheet-based gyroid microarchitecture for bone tissue engineering. Acta Biomater. 2019;94:610–626. doi: 10.1016/j.actbio.2019.05.046
- Mahmoud D, Al-Rubaie KS, Elbestawi MA. The influence of selective laser melting defects on the fatigue properties of Ti6Al4V porosity graded gyroids for bone implants. Int J Mech Sci. 2021;193:106180. doi: 10.1016/j.ijmecsci.2020.106180
- Li SJ, Murr LE, Cheng XY, et al. Compression fatigue behavior of Ti–6Al–4V mesh arrays fabricated by electron beam melting. Acta Mater. 2012;60(3):793–802. doi: 10.1016/j.actamat.2011.10.051
- Burton HE, Eisenstein NM, Lawless BM, et al. The design of additively manufactured lattices to increase the functionality of medical implants. Mater Sci Eng C. 2019;94:901–908. doi: 10.1016/j.msec.2018.10.052
- Schouman T, Schmitt M, Adam C, et al. Influence of the overall stiffness of a load-bearing porous titanium implant on bone ingrowth in critical-size mandibular bone defects in sheep. J Mech Behav Biomed Mater. 2016;59:484–496. doi: 10.1016/j.jmbbm.2016.02.036
- Van Der Stok J, Van Der Jagt OP, Amin Yavari S, et al. Selective laser melting-produced porous titanium scaffolds regenerate bone in critical size cortical bone defects. J Orthop Res. 2013;31(5):792–799. doi: 10.1002/jor.22293
- Uí Mhurchadha SM, Marques S, Givet L, et al. Effect of Voronoi lattice geometry on the fatigue performance of Ti–6Al–4V. Solid Freeform Fabrication 2021: Proceedings of the 32nd Annual International Solid Freeform Fabrication Symposium. 2021. p. 13–22.
- Budinski K. Tribological properties of titanium alloys. Wear. 1991;151:203–217. doi: 10.1016/0043-1648(91)90249-T
- Molinari A, Straffelini G, Tesi B, et al. Dry sliding wear mechanisms of the Ti6A14V alloy. Wear. 1997;208(1-2):105–112. doi: 10.1016/S0043-1648(96)07454-6
- Love CA, Cook RB, Harvey TJ, et al. Diamond like carbon coatings for potential application in biological implants – a review. Tribol Int. 2013;63:141–150. doi: 10.1016/j.triboint.2012.09.006
- Ebramzadeh E, Campbell PA, Takamura KM, et al. Failure modes of 433 metal-on-metal hip implants: how, why, and wear. Orthop Clin North Am. 2011;42(2):241–250. doi: 10.1016/j.ocl.2011.01.001
- Lachiewicz PF. Metal-on-metal hip resurfacing: a skeptic's view. Clin Orthop Relat Res. 2007;465(465):86–91. doi: 10.1097/BLO.0b013e3181468911
- Abu-Amer Y, Darwech I, Clohisy JC. Aseptic loosening of total joint replacements: mechanisms underlying osteolysis and potential therapies. Arthritis Res Ther. 2007;9(Suppl 1):S6–7. doi: 10.1186/ar2170
- Ponthiaux P, Wenger F, Drees D, et al. Electrochemical techniques for studying tribocorrosion processes. Wear. 2004;256(5):459–468. doi: 10.1016/S0043-1648(03)00556-8
- Manhabosco TM, Tamborim SM, dos Santos CB, et al. Tribological, electrochemical and tribo-electrochemical characterization of bare and nitrided Ti6Al4V in simulated body fluid solution. Corros Sci. 2011;53(5):1786–1793. doi: 10.1016/j.corsci.2011.01.057
- Milošev I, Metikoš-Huković M, Strehblow HH. Passive film on orthopaedic TiAlV alloy formed in physiological solution investigated by X-ray photoelectron spectroscopy. Biomaterials. 2000;21(20):2103–2113. doi: 10.1016/S0142-9612(00)00145-9
- Murr LE, Esquivel EV, Quinones SA, et al. Microstructures and mechanical properties of electron beam-rapid manufactured Ti–6Al–4V biomedical prototypes compared to wrought Ti–6Al–4V. Mater Charact. 2009;60(2):96–105. doi: 10.1016/j.matchar.2008.07.006
- Shahsavari M, Imani A, Setavoraphan A, et al. Electron beam surface remelting enhanced corrosion resistance of additively manufactured Ti–6Al–4V as a potential in-situ re-finishing technique. Sci Rep. 2022;12(1):11589. doi: 10.1038/s41598-022-14907-2
- Sarker A, Tran N, Rifai A, et al. Angle defines attachment: switching the biological response to titanium interfaces by modifying the inclination angle during selective laser melting. Mater Des. 2018;154:326–339. doi: 10.1016/j.matdes.2018.05.043
- Schaller RF, Mishra A, Rodelas JM, et al. The role of microstructure and surface finish on the corrosion of selective laser melted 304L. J Electrochem Soc. 2018;165(5):C234–C242. doi: 10.1149/2.0431805jes
- Willert HG, Brobäck LG, Buchhorn GH, et al. Crevice corrosion of cemented titanium alloy stems in total hip replacements. Clin Orthop Relat Res (1976–2007). 1996;333:51–75. doi: 10.1097/00003086-199612000-00006
- Linez-Bataillon P, Monchau F, Bigerelle M, et al. In vitro MC3T3 osteoblast adhesion with respect to surface roughness of Ti6Al4V substrates. Biomol Eng. 2002;19(2-6):133–141. doi: 10.1016/S1389-0344(02)00024-2
- Dolimont A, Demarbaix A, Ducobu F, et al. Chemical etching as a finishing process for electron beam melting (EBM) parts. AIP Conf Proc. 2019;2113:150020. doi: 10.1063/1.5112696
- Herzog D, Seyda V, Wycisk E, et al. Additive manufacturing of metals. Acta Mater. 2016;117:371–392. doi: 10.1016/j.actamat.2016.07.019
- Mercelis P, Kruth JP. Residual stresses in selective laser sintering and selective laser melting. Rapid Prototyp J. 2006;12(5):254–265. doi: 10.1108/13552540610707013
- Cunningham R, Narra SP, Ozturk T, et al. Evaluating the effect of processing parameters on porosity in electron beam melted Ti–6Al–4V via synchrotron X-ray microtomography. Jom. 2016;68(3):765–771. doi: 10.1007/s11837-015-1802-0
- Virtanen S, Milošev I, Gomez-Barrena E, et al. Special modes of corrosion under physiological and simulated physiological conditions. Acta Biomater. 2008;4(3):468–476. doi: 10.1016/j.actbio.2007.12.003
- Costa BC, Tokuhara CK, Rocha LA, et al. Vanadium ionic species from degradation of Ti–6Al–4V metallic implants: in vitro cytotoxicity and speciation evaluation. Mater Sci Eng C. 2019;96:730–739. doi: 10.1016/j.msec.2018.11.090
- Chiu TM, Mahmoudi M, Dai W, et al. Corrosion assessment of Ti–6Al–4V fabricated using laser powder-bed fusion additive manufacturing. Electrochim Acta. 2018;279:143–151. doi: 10.1016/j.electacta.2018.04.189
- Albrektsson T, Becker W, Coli P, et al. Bone loss around oral and orthopedic implants: an immunologically based condition. Clin Implant Dent Relat Res. 2019;21(4):786–795. doi: 10.1111/cid.12793