Abstract
Salt bladders and salt glands of recretohalophytes are specific salt-secreting structures evolving from trichomes that allow plants to adapt to extreme environmental conditions (such as salinity or drought) by precise regulation of the salt load in metabolically active leaf tissues. The knowledge of the process by which these structures are formed and operate may provide a blueprint for introducing them into cultivated crops thus creating climate-resilient genotypes. This review summarizes the recent progress in understanding mechanisms involved in the development and operation of salt bladders and glands, at physiological, molecular, and genetic levels. We show that these processes differ depending on the anatomical structure of glands (unicellular vs bi-cellular vs multicellular), as well as between salt glands and bladders. We also show that recretohalophytes with different types of salt glands may have different trichome development. We discuss new evidence for the role of vesicular transport in salt secretion and highlight the importance of multi-omics in understanding mechanisms of salt secretion and sequestration in epidermal bladder cells, and their contribution to salinity stress tolerance in plants.
The importance of secretory structures in salinity tolerance
Soil salinity imposes three major constraints on plants, namely osmotic stress, nutritional disbalance (ion toxicity), and oxidative stress (Zhao et al., Citation2020). The latter two are manifest in shoots, and their impact can be minimized by the efficient removal of potentially harmful ions (Na+ and Cl-) from metabolically active intracellular compartments. In most cases, this process implies sequestration of excessive harmful ions in vacuoles. Both halophytes and glycophytes use this mechanism, although with different levels of efficiency (Shabala and Mackay, Citation2011; Flowers and Colmer, Citation2015). Another way of handling a toxic salt load in the shoots is to remove excessive ions from mesophyll, either by storage in epidermis bladder cells (EBCs) or by transfer to salt glands and microhairs (for secretion). All these structures are classified as modified trichomes (Shabala et al., Citation2014). They originate from epidermis cells followed by their differentiation into unicellular or multicellular structures (Johnson, Citation1975; Adams et al., Citation1998; Szymanski et al., Citation2000). Most plant species have trichomes on the surface of their leaves; however, only halophyte species possess this ability to deposit the salt into these structures. We have previously argued that understanding the mechanistic basis of EBC and gland formation and operation may allow this trait to be introduced into domesticated crop species (Shabala, Citation2013; Shabala et al., Citation2014; Rawat et al., Citation2022). Although this idea is gaining momentum (Dassanayake and Larkin, Citation2017), it is still in its infancy, due to a shortage of available high-quality genome data on halophytes, a lack of genetic transformation systems, difficulties in isolating secreting structures, and limited availability of germplasm resources. This review summarizes up-to-date progress in the field focusing on mechanisms involved in the development and operation of salt bladders and glands, at physiological, molecular, and genetic levels.
Types of salt-secreting modified trichomes
Trichomes are accessory structures that originate from plant epidermal cells (Adams et al., Citation1998; Werker, Citation2000; Balkunde et al., Citation2010). In glycophytes, trichomes are typically classified into glandular trichomes (GTs) and nonglandular trichomes (NGTs), depending on their secretory ability (Singh et al., Citation2019). In recretohalophytes, modified trichomes are categorized as salt glands and salt bladders, based on their morphology. Although they also originate from epidermal cells, they evolve to have functions that differ from those of glycophytes, particularly in salt secretion under saline conditions (Shabala et al., Citation2014; Yuan and Wang, Citation2020; Yun and Shabala, Citation2021; Koteyeva et al., Citation2022) and, as such, play a vital role in salt tolerance of halophytes (Marcum, Citation1999; Kiani‐Pouya et al., Citation2017).
Each salt bladder complex consists of an epidermal bladder cell with a notably large vacuole, and a stalk cell (). EBCs are numerous on both abaxial and adaxial leaf surfaces in Atriplex centralasiatica, Chenopodium quinoa and Mesembryanthemum crystallinum. In a recent study, Lai et al. (Citation2022) reported that Glycine soja, a wild relative of cultivated soybean, possesses salt glands on the leaf surface. However, using the term “gland” is misleading in this context, as no secretory activity was shown and, anatomically, these structures consisted of a large bladder cell and a stalk cell, similar to the salt bladders of Atriplex and Chenopodium. Once the genes conferring these structures in G. soja are known, it may be possible to transfer them to cultivated soybean species, in order to improve the ability to tolerate high salinity in the soil.
Figure 1. Structure of salt-secreting modified trichomes in recretohalophytes. A. A salt bladder complex includes an epidermal bladder cell (EBC) and a stalk cell in C. album (from Zhang et al. (Citation2022) and only EBC in M. crystallinum (from Oh et al. (Citation2015); scalebar: 0.5 mm); B. Salt gland structures include single salt gland in O. coarctata (Koteyeva et al., Citation2022; n, nucleus; scalebar: 10 µm), bicellular salt gland in Chloris gayana Kunth (Oi et al., Citation2012; BC, basal cell; BR, base region of basal cell; Ca, cavity; CC, cap cell; Cu, cuticular layer; E, epidermal cell; M, mesophyll cell, n, nucleus; NR, neck region of basal cell; Va, valleculae; arrows: plasmodesmata) and multicellular salt gland in L. bicolor (Yuan et al., Citation2015; SC, secretory cell; AC, accessory cell; IC, inner cup cell; OC, outer cup cell; CC collection cell; scalebar: 5 µm). Modified from: A - Zhang et al. (Citation2022) and Oh et al. (Citation2015); B – Koteyeva et al. (Citation2022), Oi et al., (Citation2012) and Yuan et al. (Citation2015) with permission from John Wiley and Sons; Springer Nature; and University of Chicago Press.
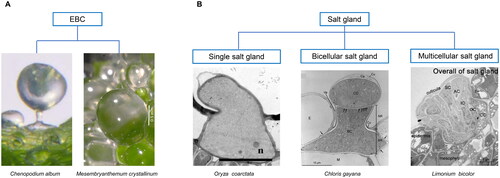
EBCs secrete salt only once upon their rupture, while, by contrast, salt glands do it on a regular basis. Salt glands can be further categorized into unicellular, bicellular and multicellular (Yuan and Wang, Citation2020; Yun and Shabala, Citation2021). Multicellular salt glands are commonly found in dicots such as in mangroves like Avicennia marina (Shimony et al., Citation1973; Chen et al., Citation2010; Guo et al., Citation2023), or Aegiceras corniculatum (Cardale and Field, Citation1971; Field et al., Citation1984), as well as in Limonium bicolor (Leng et al., Citation2018; Zhao et al., Citation2023), and Tamarix aphylla (Thomson et al., Citation1969; Bosabalidis, Citation2012). Bicellular salt glands are only found in monocots, more precisely in the Poaceae such as Zoysia matrella (Rao, Citation2011; Yamamoto et al., Citation2016), Chloris gayana (Oi et al., Citation2012, Citation2013, Citation2014), Sporobolus anglicus and Urochondra setulosa (Koteyeva et al., Citation2022). Here, a basal cell and a cap cell operate in collecting salt and in secretion, respectively (Bicellular salt gland of Citation2012; Koteyeva et al., Citation2022). The wild rice Oryza coarctata is a special example of a halophyte with a single-cell salt gland (Single salt gland of ), and salt is secreted through these glands (Koteyeva et al., Citation2022) but not the co-located microhairs (Flowers et al., Citation1990; Rajakani et al., Citation2019). Although multicellular and bicellular salt glands differ with respect to cell number and composition, they all contain collecting cells and secretory cells, and all glands typically comprise a cuticle envelope, cuticular chamber, cap cell, and basal cell (Multicellular salt gland of ) Unicellular salt glands operate in a different way that has not been characterized yet.
In addition to salt-secreting structures including EBCs and salt glands found in halophytes, other potentially secreting structures in glycophytes have also been found to secrete salt. For example, microhairs on the surface of leaves of Zea mays have been shown to secrete salt, and the microhair number and secretion rate could increase with increasing salinity concentration (Ramadan and Flowers, Citation2004). Johnsongrass could discharge salt via trichomes on the surface of its leaves when grown not in soil with high NaCl concentration but rather in soil containing lime (McWhorter et al., Citation1995). Salt crystals have been found on the leaf surface of Gossypium hirsutum after NaCl treatment, and this phenomenon was more obvious in the salt-tolerant genotype compared to the salt-sensitive one. Glandular trichomes were responsible for the salt secretion (Peng et al., Citation2016), potentially conferring stronger salt-tolerance to the upland cotton.
How do salt bladders and salt glands form?
Trichome formation is a complex process that may involve several different mechanisms (Serna and Martin, Citation2006). Most of the current knowledge comes from nonhalophytes and, specifically, Arabidopsis, where transcription factors such as R2R3 MYB, HD-ZIP IV, and basic helix-loop-helix (bHLH) were shown to play a critical role. It was shown that an R2R3 MYB transcription factor GLABRA1 (GL1), a bHLH transcription factor GLABRA3 (GL3) and ENHANCER OF GL3 (EGL3), and a WD40-repeat protein TRANSPARENT TESTA GLABRA1 (TTG1) can form a so-called MBW complex to activate the downstream gene HD-ZIP IV GLABRA2 (GL2) for initiating trichome differentiation (Zhang et al., Citation2003; Serna and Martin, Citation2006; Morohashi et al., Citation2007; Zhao et al., Citation2008). Besides, the MBW complex can activate TRIPTYCHON (TRY), CAPRICE (CPC), ENHANCER OF TRY AND CPC 1 (ETC1), ETC2, ETC3, TRICHOMELESS1 (TCL1) and TCL2, promoting their diffusion to neighboring cells to compete with GL1 for binding to GL3, thus locally suppressing trichome formation (Kirik et al., Citation2004a, Citation2004b; Wang et al., Citation2007; Pesch and Hülskamp, Citation2009; Edgar et al., Citation2014; Wang and Chen, Citation2014). Recently, this regulatory mechanism has also been described in Camellia sinensis where CsMYB1 interacts with CsGL3 and CsWD40 to form a transcriptional MBW complex of MYB-bHLH-WD40 to activate trichome regulator genes CsGL2 and CsCPC and this MBW complex also participates in catechin biosynthesis in tea (Li et al., Citation2022). These findings suggest that trichome development is a complex process where various transcription factors form a type of complex to interact with other transcription factors, and in plants like C. sinensis this complex could be involved in multiple biological pathways.
Salt bladder development-related genes
Despite EBC being a prominent feature in many species, most of the knowledge comes from M. crystallinum and C. quinoa. A small number of genes involved in EBCs or trichome formation have been partially characterized (). In M. crystallinum, WM28, a putative jasmonate-induced gene, may elevate GL1 and GL3 expression known to facilitate trichome development in Arabidopsis (Roeurn et al., Citation2016), indicating this gene could potentially be involved in EBC formation. Homologs of trichome development-involved genes in A. thaliana have been found in both M. crystallinum and C. quinoa and have been reported to participate in EBC development. R2R3-MYB McMYB2, the homolog of AtMYB5, can interact with bHLH proteins and a WD40 protein to form a MBW complex that regulates trichome development. The homolog of GL2, McC4HDZ encoding a Class IV Homeodomain Leucine Zip (HD-Zip IV) protein, can recover the phenotype of the Arabidopsis gl2 mutant to some extent, thus taking over GL2 function in this species. Thus, this gene may contribute to EBCs formation (Roeurn et al., Citation2017). Imamura et al. (Citation2020) identified a gene, REDUCED EPIDERMAL BLADDER CELLS (REBC), encoding a WD40 protein, which was responsible for EBC formation in quinoa. In a rebc mutant the number of EBCs decreased to less than 0.5% of the wild type. However, REBC has a low similarity to WD40-protein encoded by TTG1 in Arabidopsis and REBC cannot complement the ttg1 mutant of that species, whereas two quinoa homologs of TTG1, CqTTG1-like1 and CqTTG1-like2 can do this. This indicates that CqTTG1-like1/2 may be involved in root hair but not in trichome development because there are no other trichomes on the leaf surface of quinoa. Mutating simultaneously REBC and its homolog, REBC-like1, completely suppressed EBC formation on the leaf surface and on the inflorescence of quinoa plants, which was not observed by only mutating REBC-like1. Hence, REBC-like1 may act as an enhancer of REBC in regulating EBCs formation (Moog et al., Citation2022). These studies suggest EBC formation is likely to be different from the trichome formation of Arabidopsis. In the future, TTG1 in Arabidopsis needs to be tested with respect to its ability to complement rebc or/and rebc-like1 mutants of quinoa when the genetic transformation system for quinoa becomes mature, to further supplement or refine our knowledge of the mechanisms of EBC formation. Interestingly, rebc rebc-like1 double mutants did not differ from the wild types in terms of salt tolerance when treated with low and high NaCl concentrations, despite the mutants lacking EBCs completely, which contradicts the accepted view that EBCs contribute to salt tolerance of quinoa by Na+ and Cl- secretion (Agarie et al., Citation2007; Kiani‐Pouya et al., Citation2017; Kiani-Pouya et al., Citation2019; Yun and Shabala, Citation2021). However, it should be noted that in Moog et al. (Citation2022) WT plants responded to salinity treatment by a dose-dependent decline in their biomass (i.e., possessed a glycophytic type of response) while in other papers cited above moderate levels of salt (around 100 mM NaCl for quinoa) were found to be optimal for plant growth. It appears that some other factors (in addition to salinity) affected plant performance in these experiments, making a direct comparison impossible. In lay terms: under conditions of experiments by Moog et al. (Citation2022) WT quinoa plants did not behave like a halophyte and, hence, may not have used EBCs to deal with the salt load.
Table 1. Genes involved in formation of salt bladders or glands in recretohalophytes.
Salt gland development-related genes
L. bicolor is arguably the most studied species for understanding salt gland development in halophytes, but knowledge of genes involved in salt gland development is very limited (). Even though homologs of some trichome development-related genes like GL1, TTG1, GL3/EGL3 can be detected in L. bicolor, homologs of GL2 and TTG2 are absent (), which may explain why there is no other type of trichomes on its leaf surface (Yuan et al., Citation2022). Remarkably though, GL2, GL3 and TTG2 are highly conserved among glycophytes and other recretohalophytes, respectively, as shown in . A homolog of TTG1, LbTTG1 has been detected in L. bicolor, and Yuan et al. (Citation2019) found that when overexpressed heterologously in Arabidopsis, LbTTG1 could up-regulate the expression of GL1 and GL3, promoting trichome development. A decreased expression of TRY and CPC inhibits trichome development, consistent with the function of TTG1 in trichome development in Arabidopsis (Zhao et al., Citation2008; Yuan et al., Citation2019). It was also shown that LbHLH functions in the same way as LbTTG1 when interacting with GL1 (Wang et al., Citation2021). However, these two genes act as a negative regulator in salt gland development, as overexpression of LbTTG1 and LbHLH in L. bicolor led to a reduced salt gland number and abnormal salt glands (Yuan et al., Citation2022). Meanwhile, LbHLH cannot complement gl3 single and gl3 egl3 double mutants. These reports suggest that genes involved in salt gland development in recretohalophytes like LbTTG1 and LbHLH have specific functions that differ from those in nonhalophytes, although they have the ability to participate in trichome and root hair development to some degree. Interestingly, the homologs of TTG1 are absent in O. coarctata (), suggesting that such unicellular salt gland development may differ from the bicellular and multicellular salt glands provided that TTG1 really participates in bicellular and multicellular salt gland development. In L. bicolor, R1-type MYB LbMYB48 might indirectly negatively regulate the expression of LbCPC-like, a homolog of AtCPC known to bind TTG1 and GL3 to form complexes inhibiting trichome development in Arabidopsis and DISTORTED3 (LbDIS3) to negatively regulate the formation of salt glands (Wada et al., Citation1997; Tominaga et al., Citation2007; Han et al., Citation2022). Another gene, Lb1G04794, located in the nucleus, promoted salt gland development as a transcription factor when overexpressed in L. bicolor, while also increasing trichome number but reducing root hairs when overexpressed in Arabidopsis (Jiao et al., Citation2022). Unlike Lb1G04794, a MYB LbCPC, that is homologous to AtCPC, has the opposite effect. This gene inhibited salt gland formation and density when overexpressed in L. bicolor and inhibited trichome development. It was shown that it may combine with GL3 to restrain the formation of TTG1-GL3-GL1 complex when overexpressed in Arabidopsis (Zou et al., Citation2023). Except for transcription factors, Limonium homologs of other genes in Arabidopsis involved in trichome initiation have been reported. LbSAD2, a homolog of SUPER SENSITIVE TO ABA AND DROUGHT2 (AtSAD2), has been reported to positively affect trichome numbers through mediating probably GL3 and improving salt tolerance when overexpressed in Arabidopsis. This gene was located and highly expressed in the salt gland during their formation of L. bicolor (Xu et al., Citation2020), indicating its functional role in this process.
Figure 2. Maximum likelihood tree of GL2 (A), TTG2 (B) and GL3 (C) proteins from glycophytes and recretohalophytes. Genes in glycophytes and recretohalophytes were marked by solid circle. The percentage of trees in which the associated taxa clustered together is shown next to the branches. The tree is drawn to scale, with branch lengths measured in the number of substitutions per site. Evolutionary analyses were conducted in MEGA X.
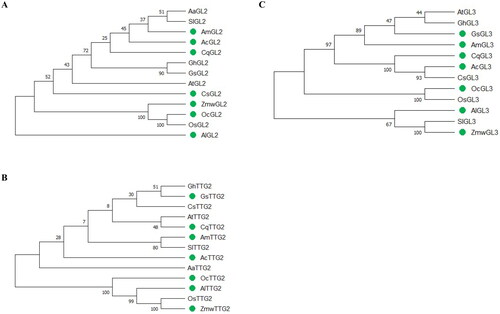
Table 2. Homologs of trichome development-related genes in glycophytes and recretohalophytes.
How do salt bladders or salt glands of recretohalophytes secrete NaCl?
There is currently a limited knowledge on the molecular mechanisms conferring ion transport to salt glands and bladders and their secretion. The major hurdles are difficulties in isolating them, and the absence of a genetic transformation systems. As a result, most of the current knowledge comes from microscopical studies and multiple omics including genomics, transcriptomics, proteomics, metabolomics and ionomics.
The majority of published research originates from salt bladders of C. quinoa and M. crystallinum. As the progress on ion transport of salt bladders has been reviewed recently by Yun and Shabala (Citation2021), it will not be discussed in detail in this section. Instead, we will focus on ion transport and secretion in salt glands. The latter involves three steps: the entry of ions into the glands from mesophyll and epidermal cells; the transport of ions within the salt glands; and the secretion of ions out of the glands. While several hypotheses have been proposed regarding salt secretion in recretohalophytes, it is still unclear which pathways - apoplastic or symplastic pathways or both – play a key role in the transfer of ions from mesophyll and epidermal cells to the basal cells of the glands. Plasmodesmata have been observed between basal cells and neighboring mesophyll and epidermal cells in dicots and the Poaceae S. virginicus, S. anglicus and U. setulose (Naidoo and Naidoo, Citation2008; Koteyeva et al., Citation2022). However, plasmodesmata were present only between the gland and adjacent epidermal cells in O. coarctata (Koteyeva et al., Citation2022), and in other species (Z. matrella) such plasmodesmata were reported for some but not all genotypes (Rao, Citation2011).
In Z. matrella, ZmatHKT1, Na+-K+ transporter was predominately expressed in the salt glands, bundle sheath cells and mesophyll cells between salt glands in the adaxial side leaf, but not in the nonsecreting abaxial side (Rao, Citation2011), indicating ZmatHKT1 would be likely to participate in ion transport into basal cells from their adjacent cells. Once the ions enter the salt glands, they move via similar pathways. Additionally, these ions are likely to enter the secretory cells via vesicle transport. Electron-dense zones were found inside the vesicles by Thomson and Liu (Citation1967), and these vesicles could fuse with the plasma membrane after salt treatment (Feng et al., Citation2014; Yuan et al., Citation2015). Using Corona Green, a sodium dye, we localized the Na+ distribution and found that Na+ was enriched in these vesicles. The salt glands showed more Na+ in their vesicles under saline conditions when compared to the control without salt as shown in . Hence, ions, at least Na+, could be transported from the basal cells to secretory cells by exocytosis. Finally, the ions enter the collecting chamber and are secreted out of the salt glands through pores. Four pores were observed in L. bicolor using scanning electron microscopy (SEM) (Leng et al., Citation2019) whereas no pores were found on the globular surface of salt glands in the Poaceae recretohalophyte Rhodes grass (Chloris gayana Kunth) using SEM (Oi et al., Citation2013). This raises the question if ions are secreted via pores or if other pathways are used, calling for more studies on these issues.
Figure 3. Confocal imaging of Na+ fluorescence using CoroNa Green AM in salt glands of A. lagopoides under control and 300 mM NaCl for 3 days.
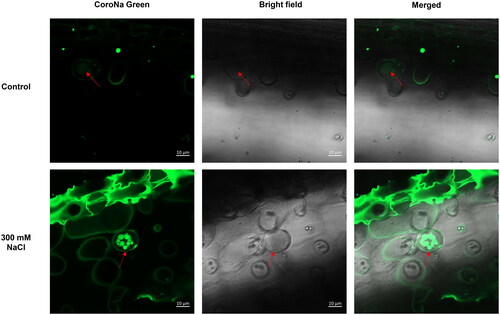
In addition to the aforementioned Na+, Cl- and K+ could also be secreted via salt glands alongside a long list of other inorganic and organic compounds such as macro- and micro-elements, toxic metals and even some metabolites (Pollak and Waisel, Citation1970; Kadukova et al., Citation2008; Naz et al., Citation2009; Ding et al., Citation2010). Glandular trichomes (GTs) can produce, store, and secrete secondary metabolites (Konarska and Łotocka, Citation2020; Sun et al., Citation2022). In addition, GTs in the leaves of G. hirsutism have the ability to secrete salt ions (Peng et al., Citation2016), and GTs of tobacco can secrete cadmium (Cd) and calcium (Ca) (Choi et al., Citation2001), and secrete more cadmium than NGTs in a Cd-enriched environment (Liu et al., Citation2019). Salt glands exhibit similar secretion functions, including ions and metabolites secretion, as well as morphology with GTs.
Sequenced recretohalophyte genomes and their roles in understanding formation of secreting structures
Whole genome sequencing has been applied to different types of organisms including microorganisms, animals, and plants. It helps to explore the biological functions of genes, to unravel evolutionary history through comparative genome analysis and to advance breeding programs. Near 600 plant genomes have been sequenced and completely assembled (Kersey, Citation2019). High-quality plant genomes, with superior assembly and annotation, provide valuable insights that address issues pertaining to plant biology, by integrating transcriptomes from various perspectives. So far, 12 genomes of halophytes possessing secreting structures including epidermal bladder cells (EBCs), multicellular, bicellular and unicellular salt glands have been sequenced as shown in . Among these genomes, only five genomes have been assembled at the chromosome-level including M. crystallinum, A. marina, A. corniculatum, G. soja and L. bicolor. However, only the genomes of A. marina and A. corniculatum are available to the public.
Table 3. Genomes-sequenced of recretohalophytes with salt bladders or salt glands until 2023.
Based on high-quality genomes, transcriptomes of EBCs of C. quinoa revealed the key genetic mechanisms in ion transport into EBCs. Ion transporters such as SLAH, NRT, ClC, NHX, and HKT1, as well as sugar transporters like SWEET, were found to be involved in the sequestration of ions and energy into specialized cells known as EBCs (Böhm et al., Citation2018). The EBC transcriptome of M. crystallinum comprised differentially expressed genes involved, among other things, in ion transport, energy metabolism and osmotic adjustment (Oh et al., Citation2015). Transcription factors of the bHLH family were identified in the high-quality genome of the mangrove Avicennia marina, followed by MYB, indicating these TFs play important roles in salt tolerance and are likely involved in salt gland development (Natarajan et al., Citation2021). L. bicolor genome contains trichome development-related transcription factors and some like the mentioned LbHLH and LbTTG1 have been characterized in more detail, providing new insights into salt gland development and salt tolerance (Yuan et al., Citation2022). These reports suggest that multi-omics, especially genome and transcriptome sequencing, plays a vital role in understanding the various aspects of secreting structures.
Perspectives
Until now, research progress on EBCs and salt glands has been slow, with many studies focusing on their physiological or morphological traits, while the mechanisms of their development and secretion remain poorly understood. Although EBCs can readily be isolated due to their larger size, and genomes of C. quinoa and M. crystallinum have been published, a suitable transformation system is still lacking. Despite the fact that the development of EBCs has been partially revealed through the use of mutants, a genetic transformation system of quinoa needs to be established. The role of EBC in salt tolerance also needs to be studied in more detail, given some controversial reports in the literature (e.g., Kiani-Pouya et al. 2017, Citation2019 vs Moog et al. Citation2022). It is plausible to suggest that the roles of EBC may be numerous, operating not only in damping salt load but also being a major storage reservoir for K+ and water.
Even though some genes involved in salt glands have been characterized, most of them are detected based on homology search, and their function was verified in a heterologous expression system, which may render misleading results. Applying multi-omics to mutants with salt gland abnormalities may be combined with genetic manipulation in the future to reveal mechanisms of salt gland formation and salt secretion. In light of the available genetic transformation system, recently published chromosome-level genome, and a wealth of previous studies, L. bicolor represents an excellent candidate for the study of salt gland development and secreting mechanisms, especially since it enables easy exploration of multicellular salt gland development and secreting mechanisms. Given that salt glands comprise different cellular types, the mechanisms regulating their development and secretion may differ to some extent, just as trichome development varies between Arabidopsis and rice. For a full understanding of salt gland evolution and their potential transfer to crops, it is necessary to study the development and secreting mechanisms of salt glands with different cellular types. O. coarctata, a wild relative of rice, is a type of unicellular salt gland and only secreted on the adaxial leaf surface, which makes it a suitable model for studying how salt glands form and how salt is secreted through them using conventional and modern methods. The Aeluropus family can be regarded as a representative of the bicellular salt glands that can be easily and quickly reproduced using cottage. G. soja, as a wild relative of cultivated soybean G. max, has the potential of making it possible to apply salt bladders or glands to G. max based on multiple-omics as there is a high homology between them, making it easy to exchange genetic materials (Li et al., Citation2014; Singh, Citation2019; Xie et al., Citation2019). Additionally, cultivated soybean genetic transformation system and CRISPR/Cas9 can be further applied to wild soybeans, to understand mechanisms underlying salt secretion. However, the primary challenge in studying salt gland development and secreting mechanisms is the difficulty of isolating salt glands from multiple epidermal cells. Salt glands do exhibit autofluorescence under ultraviolet excitation at the wavelength of 330-380 nm (Deng et al., Citation2015), which can be utilized in combination with laser capture microdissection (LCM) to acquire salt glands in the future. Following the acquisition of salt glands, multi-omics analysis can be employed to identify key genes responsible for salt gland development and salt secretion, which can subsequently be confirmed through transformation systems.
Additional information
Funding
References
- Adams, P., Nelson, D. E., Yamada, S., Chmara, W., Jensen, R. G., Bohnert, H. J., and Griffiths, H. 1998. Growth and development of Mesembryanthemum crystallinum (Aizoaceae). New Phytol. 138:171–190. doi:10.1046/j.1469-8137.1998.00111.x
- Agarie, S., Shimoda, T., Shimizu, Y., Baumann, K., Sunagawa, H., Kondo, A., Ueno, O., Nakahara, T., Nose, A., and Cushman, J. C. 2007. Salt tolerance, salt accumulation, and ionic homeostasis in an epidermal bladder-cell-less mutant of the common ice plant Mesembryanthemum crystallinum. J. Exp. Bot. 58:1957–1967. doi:10.1093/jxb/erm057
- Balkunde, R., Pesch, M., and Hülskamp, M. 2010. Trichome patterning in Arabidopsis thaliana from genetic to molecular models. Curr. Top. Dev. Biol. 91:299–321. doi:10.1016/S0070-2153(10)91010-7
- Bansal, J., Gupta, K., Rajkumar, M. S., Garg, R., and Jain, M. 2021. Draft genome and transcriptome analyses of halophyte rice Oryza coarctata provide resources for salinity and submergence stress response factors. Physiol. Plant. 173:1309–1322. doi:10.1111/ppl.13284
- Böhm, J., Messerer, M., Müller, H. M., Scholz-Starke, J., Gradogna, A., Scherzer, S., Maierhofer, T., Bazihizina, N., Zhang, H., Stigloher, C., Ache, P., Al-Rasheid, K. A. S., Mayer, K. F. X., Shabala, S., Carpaneto, A., Haberer, G., Zhu, J.-K., and Hedrich, R. 2018. Understanding the molecular basis of salt sequestration in epidermal bladder cells of Chenopodium quinoa. Curr. Biol. 28:3075–3085.e7. doi:10.1016/j.cub.2018.08.004
- Bosabalidis, A. M. 2012. Programmed cell death in salt glands of Tamarix aphylla L.: an electron microscope analysis. Cent. Eur. J. Biol. 7:927–930. doi:10.2478/s11535-012-0067-7
- Cardale, S., and Field, C. D. 1971. The structure of the salt gland of Aegiceras corniculatum. Planta 99:183–191. doi:10.1007/BF00386836
- Chen, J., Xiao, Q., Wu, F., Dong, X., He, J., Pei, Z., Zheng, H., and Näsholm, T. 2010. Nitric oxide enhances salt secretion and Na+ sequestration in a mangrove plant, Avicennia marina, through increasing the expression of H+-ATPase and Na+/H+ antiporter under high salinity. Tree Physiol. 30:1570–1585. doi:10.1093/treephys/tpq086
- Choi, Y.-E., Harada, E., Wada, M., Tsuboi, H., Morita, Y., Kusano, T., and Sano, H. 2001. Detoxification of cadmium in tobacco plants: formation and active excretion of crystals containing cadmium and calcium through trichomes. Planta 213:45–50. doi:10.1007/s004250000487
- Dassanayake, M., and Larkin, J. C. 2017. Making plants break a sweat: the structure, function, and evolution of plant salt glands. Front. Plant Sci. 8:406. doi:10.3389/fpls.2017.00406
- Deng, Y., Feng, Z., Yuan, F., Guo, J., Suo, S., and Wang, B. 2015. Identification and functional analysis of the autofluorescent substance in Limonium bicolor salt glands. Plant Physiol. Biochem. 97:20–27. doi:10.1016/j.plaphy.2015.09.007
- Ding, F., Yang, J.-C., Yuan, F., and Wang, B.-S. 2010. Progress in mechanism of salt excretion in recretohalopytes. Front. Biol. 5:164–170. doi:10.1007/s11515-010-0032-7
- Edgar, B. A., Zielke, N., and Gutierrez, C. 2014. Endocycles: a recurrent evolutionary innovation for post-mitotic cell growth. Nat. Rev. Mol. Cell Biol. 15:197–210. doi:10.1038/nrm3756
- Feng, X., Li, G., Xu, S., Wu, W., Chen, Q., Shao, S., Liu, M., Wang, N., Zhong, C., He, Z., and Shi, S. 2021. Genomic insights into molecular adaptation to intertidal environments in the mangrove Aegiceras corniculatum. New Phytol. 231:2346–2358. doi:10.1111/nph.17551
- Feng, Z., Sun, Q., Deng, Y., Sun, S., Zhang, J., and Wang, B. 2014. Study on pathway and characteristics of ion secretion of salt glands of Limonium bicolor. Acta Physiol. Plant. 36:2729–2741. doi:10.1007/s11738-014-1644-3
- Field, C. D., Hinwood, B. G., and Stevenson, I. 1984. Structural features of the salt gland of Aegiceras. In Physiology and Management of Mangroves; Teas H. J., Ed. Dordrecht: Springer. p. 37–42.
- Flowers, T. J., and Colmer, T. D. 2015. Plant salt tolerance: adaptations in halophytes. Ann. Bot. 115:327–331. doi:10.1093/aob/mcu267
- Flowers, T. J., Flowers, S. A., Hajibagheri, M. A., and Yeo, A. R. 1990. Salt tolerance in the halophytic wild rice, Porteresia coarctata Tateoka. New Phytol. 114:675–684. doi:10.1111/j.1469-8137.1990.tb00439.x
- Friis, G., Vizueta, J., Smith, E. G., Nelson, D. R., Khraiwesh, B., Qudeimat, E., Salehi-Ashtiani, K., Ortega, A., Marshell, A., Duarte, C. M., et al. 2020. A high-quality genome assembly and annotation of the gray mangrove, Avicennia marina. G3-Genes Genomes Genet. 11:jkaa025.
- Guo, Z., Wei, M.-Y., Zhong, Y.-H., Wu, X., Chi, B.-J., Li, J., Li, H., Zhang, L.-D., Wang, X.-X., Zhu, X.-Y., and Zheng, H.-L. 2023. Leaf sodium homeostasis controlled by salt gland is associated with salt tolerance in mangrove plant Avicennia marina. Tree Physiol. 43:817–831. doi:10.1093/treephys/tpad002
- Han, G., Qiao, Z., Li, Y., Yang, Z., Zhang, Z., Zhang, Y., Guo, J., Liu, L., Wang, C., and Wang, B. 2022. LbMYB48 positively regulates salt gland development of Limonium bicolor and salt tolerance of plants. Front. Plant Sci. 13:1039984. doi:10.3389/fpls.2022.1039984
- Hashemi-Petroudi, S. H., Arab, M., Dolatabadi, B., Kuo, Y.-T., Baez, M. A., Himmelbach, A., Nematzadeh, G., Maibody, S. A. M. M., Schmutzer, T., Mälzer, M., Altmann, T., and Kuhlmann, M. 2022. Initial Description of the Genome of Aeluropus littoralis, a Halophile Grass. Front. Plant Sci. 13:906462. doi:10.3389/fpls.2022.906462
- Imamura, T., Yasui, Y., Koga, H., Takagi, H., Abe, A., Nishizawa, K., Mizuno, N., Ohki, S., Mizukoshi, H., and Mori, M. 2020. A novel WD40-repeat protein involved in formation of epidermal bladder cells in the halophyte quinoa. Commun. Biol. 3:513. doi:10.1038/s42003-020-01249-w
- Jarvis, D. E., Ho, Y. S., Lightfoot, D. J., Schmöckel, S. M., Li, B., Borm, T. J. A., Ohyanagi, H., Mineta, K., Michell, C. T., Saber, N., Kharbatia, N. M., Rupper, R. R., Sharp, A. R., Dally, N., Boughton, B. A., Woo, Y. H., Gao, G., Schijlen, E. G. W. M., Guo, X., Momin, A. A., Negrão, S., Al-Babili, S., Gehring, C., Roessner, U., Jung, C., Murphy, K., Arold, S. T., Gojobori, T., Linden, C. G. v d., van Loo, E. N., Jellen, E. N., Maughan, P. J., and Tester, M. 2017. The genome of Chenopodium quinoa. Nature 542:307–312. doi:10.1038/nature21370
- Jiao, X., Zhao, B., Wang, B., and Yuan, F. 2022. An uncharacterized gene Lb1G04794 from Limonium bicolor promotes salt tolerance and trichome development in Arabidopsis. Front. Plant Sci. 13:1079534. doi:10.3389/fpls.2022.1079534
- Johnson, H. B. 1975. Plant pubescence: An ecological perspective. Bot. Rev. 41:233–258. doi:10.1007/BF02860838
- Kadukova, J., Manousaki, E., and Kalogerakis, N. 2008. Pb and Cd accumulation and phyto-excretion by salt cedar (Tamarix Smyrnensis Bunge). Int. J. Phytorem. 10:31–46. doi:10.1080/15226510701827051
- Kersey, P. J. 2019. Plant genome sequences: past, present, future. Curr. Opin. Plant Biol. 48:1–8. doi:10.1016/j.pbi.2018.11.001
- Kiani-Pouya, A., Rasouli, F., Bazihizina, N., Zhang, H., Hedrich, R., and Shabala, S. 2019. A large-scale screening of quinoa accessions reveals an important role of epidermal bladder cells and stomatal patterning in salinity tolerance. Environ. Exp. Bot. 168:103885. doi:10.1016/j.envexpbot.2019.103885
- Kiani‐Pouya, A., Roessner, U., Jayasinghe, N. S., Lutz, A., Rupasinghe, T., Bazihizina, N., Bohm, J., Alharbi, S., Hedrich, R., and Shabala, S. 2017. Epidermal bladder cells confer salinity stress tolerance in the halophyte quinoa and Atriplex species. Plant. Cell Environ. 40:1900–1915. doi:10.1111/pce.12995
- Kirik, V., Simon, M., Huelskamp, M., and Schiefelbein, J. 2004a. The ENHANCER OF TRY AND CPC1 gene acts redundantly with TRIPTYCHON and CAPRICE in trichome and root hair cell patterning in Arabidopsis. Dev. Biol. 268:506–513. doi:10.1016/j.ydbio.2003.12.037
- Kirik, V., Simon, M., Wester, K., Schiefelbein, J., and Hulskamp, M. 2004b. ENHANCER of TRYand CPC 2(ETC2) reveals redundancy in the region-specific control of trichome development of Arabidopsis. Plant Mol. Biol. 55:389–398. doi:10.1007/s11103-004-0893-8
- Konarska, A., and Łotocka, B. 2020. Glandular trichomes of Robinia viscosa Vent. var. hartwigii (Koehne) Ashe (Faboideae, Fabaceae)—morphology, histochemistry and ultrastructure. Planta 252:102. doi:10.1007/s00425-020-03513-z
- Koteyeva, N. K., Voznesenskaya, E. V., Berim, A., Gang, D. R., and Edwards, G. E. 2022. Structural diversity in salt excreting glands and salinity tolerance in Oryza coarctata, Sporobolus anglicus and Urochondra setulosa. Planta 257:9. doi:10.1007/s00425-022-04035-6
- Lai, Y., Lu, J., Yu, H., Yang, G., Cao, N., Ma, Y., Bi, Y.-D., Tao, J., Gao, W., Niu, L., et al. 2022. Structural evolution is the key to paternal identification in salt-resistant soybean breeding. PREPRINT (Version 1) Available at Research Square. doi:10.21203/rs.3.rs-1533022/v1
- Leng, B. Y., Yuan, F., Dong, X. X., and Wang, B. S. 2018. Salt gland distribution in limonium bicolor at the individual level. In Proceedings of the IOP Conference Series: Earth and Environmental Science, 3rd International Conference on Advances in Energy Resources and Environment Engineering, Harbin, China, Dec 8–10; Vol. 113, p 012202.
- Leng, B., Dong, X., Lu, C., Li, K., Xu, Y., Yuan, F., and Wang, B. 2019. The lb23 mutant of recretohalophyte Limonium bicolor (Bag.) Kuntze with 20-, 24-, 28- and 32-cell salt glands shows elevated salt secretion. Flora 259:151441. doi:10.1016/j.flora.2019.151441
- Li, P., Fu, J., Xu, Y., Shen, Y., Zhang, Y., Ye, Z., Tong, W., Zeng, X., Yang, J., Tang, D., Li, P., Zuo, H., Wu, Q., Xia, E., Wang, S., and Zhao, J. 2022. CsMYB1 integrates the regulation of trichome development and catechins biosynthesis in tea plant domestication. New Phytol. 234:902–917. doi:10.1111/nph.18026
- Li, Y-H., Zhou, G., Ma, J., Jiang, W., Jin, L-G., Zhang, Z., Guo, Y., Zhang, J., Sui, Y., Zheng, L., Zhang, S.-S., Zuo, Q., Shi, X.-H., Li, Y.-F., Zhang, W.-K., Hu, Y., Kong, G., Hong, H.-L., Tan, B., Song, J., Liu, Z.-X., Wang, Y., Ruan, H., Yeung, C. K. L., Liu, J., Wang, H., Zhang, L-J., Guan, R.-X., Wang, K.-J., Li, W.-B., Chen, S.-Y., Chang, R.-Z., Jiang, Z., Jackson, S. A., Li, R., and Qiu, L.-J. 2014. De novo assembly of soybean wild relatives for pan-genome analysis of diversity and agronomic traits. Nat. Biotechnol. 32:1045–1052. doi:10.1038/nbt.2979
- Liu, P., Yan, X., Yang, X., Yang, Y., Zhang, H., Cui, H., and Wang, Z. 2019. Different roles of tobacco glandular and non-glandular trichomes in response to cadmium stress. J. Agric. Sci. Technol. 21:55–60.
- Ma, D., Guo, Z., Ding, Q., Zhao, Z., Shen, Z., Wei, M., Gao, C., Zhang, L., Li, H., Zhang, S., Li, J., Zhu, X., and Zheng, H.-L. 2021. Chromosome‐level assembly of the mangrove plant Aegiceras corniculatum genome generated through Illumina, PacBio and Hi‐C sequencing technologies. Mol. Ecol. Resour. 21:1593–1607. doi:10.1111/1755-0998.13347
- Marcum, K. B. 1999. Salinity tolerance mechanisms of grasses in the subfamily Chloridoideae. Crop Sci. 39:1153–1160. doi:10.2135/cropsci1999.0011183X003900040034x
- McWhorter, C. G., Paul, R. N., and Ouzts, J. C. 1995. Bicellular trichomes of johnsongrass (Sorghum halepense) leaves: morphology, histochemistry, and function. Weed Sci. 43:201–208. doi:10.1017/S0043174500081078
- Mochida, K., Sakurai, T., Seki, H., Yoshida, T., Takahagi, K., Sawai, S., Uchiyama, H., Muranaka, T., and Saito, K. 2017. Draft genome assembly and annotation of Glycyrrhiza uralensis, a medicinal legume. Plant J. 89:181–194. doi:10.1111/tpj.13385
- Moog, M. W., Trinh, M. D. L., Nørrevang, A. F., Bendtsen, A. K., Wang, C., Østerberg, J. T., Shabala, S., Hedrich, R., Wendt, T., and Palmgren, M. 2022. The epidermal bladder cell‐free mutant of the salt‐tolerant quinoa challenges our understanding of halophyte crop salinity tolerance. New Phytol. 236:1409–1421. doi:10.1111/nph.18420
- Morohashi, K., Zhao, M., Yang, M., Read, B., Lloyd, A., Lamb, R., and Grotewold, E. 2007. Participation of the Arabidopsis bHLH factor GL3 in trichome initiation regulatory events. Plant Physiol. 145:736–746. doi:10.1104/pp.107.104521
- Naidoo, Y., and Naidoo, G. 2008. Localization of potential ion transport pathways in the salt glands of the halophyte Sporobolus virginicus. In Ecophysiology of High Salinity Tolerant Plants; Khan, M. A., and Weber, D. J., Eds. Springer: Dordrecht, pp 173–185.
- Natarajan, P., Murugesan, A. K., Govindan, G., Gopalakrishnan, A., Kumar, R., Duraisamy, P., Balaji, R., Shyamli, P. S., Parida, A. K., Parani, M. and Tanuja,. 2021. A reference-grade genome identifies salt-tolerance genes from the salt-secreting mangrove species Avicennia marina. Commun. Biol. 4:851. doi:10.1038/s42003-021-02384-8
- Naz, N., Hameed, M., Wahid, A., Arshad, M., and Ahmad, M. S. A. 2009. Patterns of ion excretion and survival in two stoloniferous arid zone grasses. Physiol. Plant. 135:185–195. doi:10.1111/j.1399-3054.2008.01187.x
- Oh, D., Barkla, B. J., Vera‐Estrella, R., Pantoja, O., Lee, S., Bohnert, H. J., and Dassanayake, M. 2015. Cell type‐specific responses to salinity – the epidermal bladder cell transcriptome of Mesembryanthemum crystallinum. New Phytol. 207:627–644. doi:10.1111/nph.13414
- Oi, T., Hirunagi, K., Taniguchi, M., and Miyake, H. 2013. Salt excretion from the salt glands in Rhodes grass (Chloris gayana Kunth) as evidenced by low-vacuum scanning electron microscopy. Flora 208:52–57. doi:10.1016/j.flora.2012.12.006
- Oi, T., Miyake, H., and Taniguchi, M. 2014. Salt excretion through the cuticle without disintegration of fine structures in the salt glands of Rhodes grass (Chloris gayana Kunth). Flora 209:185–190. doi:10.1016/j.flora.2014.02.004
- Oi, T., Taniguchi, M., and Miyake, H. 2012. Morphology and ultrastructure of the salt glands on the leaf surface of Rhodes grass (Chloris gayana Kunth). Int. J. Plant Sci. 173:454–463. doi:10.1086/665588
- Peng, Z., He, S., Sun, J., Pan, Z., Gong, W., Lu, Y., and Du, X. 2016. Na+ compartmentalization related to salinity stress tolerance in upland cotton (Gossypium hirsutum) seedlings. Sci. Rep. 6:34548. doi:10.1038/srep34548
- Pesch, M., Hülskamp, M. 2009. One, two, three…models for trichome patterning in Arabidopsis? Curr. Opin. Plant Biol. 12:587–592. doi:10.1016/j.pbi.2009.07.015
- Pollak, G., and Waisel, Y. 1970. Salt secretion in Aeluropus litoralis (Willd.) Parl. Ann. Bot. 34:879–888. doi:10.1093/oxfordjournals.aob.a084419
- Rajakani, R., Sellamuthu, G., V, S., S, K., Shabala, L., Meinke, H., Chen, Z., Zhou, M., Parida, A., Shabala, S., and Venkataraman, G. 2019. Microhair on the adaxial leaf surface of salt secreting halophytic Oryza coarctata Roxb. show distinct morphotypes: Isolation for molecular and functional analysis. Plant Sci. 285:248–257. doi:10.1016/j.plantsci.2019.05.004
- Ramadan, T., and Flowers, T. J. 2004. Effects of salinity and benzyl adenine on development and function of microhairs of Zea mays L. Planta 219:639–648. doi:10.1007/s00425-004-1269-7
- Rao, S. S. 2011. Elucidation of mechanisms of salinity tolerance in Zoysia matrella cultivars: A study of structure and function of salt glands. PhD thesis, Texas A&M University, College Station.
- Rawat, N., Wungrampha, S., Singla-Pareek, S. L., Yu, M., Shabala, S., and Pareek, A. 2022. Rewilding staple crops for the lost halophytism: Toward sustainability and profitability of agricultural production systems. Mol. Plant. 15:45–64. doi:10.1016/j.molp.2021.12.003
- Roeurn, S., Hoshino, N., Soejima, K., Inoue, Y., Cushman, J. C., and Agarie, S. 2016. Suppression subtractive hybridization library construction and identification of epidermal bladder cell related genes in the common ice plant, Mesembryanthemum crystallinum L. Plant Prod. Sci. 19:552–561. doi:10.1080/1343943X.2016.1221320
- Roeurn, S., Hoshino, N., Soejima, K., Inoue, Y., Cushman, J. C., and Agarie, S. 2017. MYB and HD-ZIP IV homologs related to trichome formation are involved in epidermal bladder cell development in the halophyte Mesembryanthemum crystallinum L. Plant Prod. Sci. 20:72–82. doi:10.1080/1343943X.2017.1279528
- Serna, L., and Martin, C. 2006. Trichomes: different regulatory networks lead to convergent structures. Trends Plant Sci. 11:274–280. doi:10.1016/j.tplants.2006.04.008
- Shabala, S. 2013. Learning from halophytes: physiological basis and strategies to improve abiotic stress tolerance in crops. Ann. Bot. 112:1209–1221. doi:10.1093/aob/mct205
- Shabala, S., and Mackay, A. 2011. Ion transport in halophytes. Adv. Bot. Res. 57:151–199.
- Shabala, S., Bose, J., and Hedrich, R. 2014. Salt bladders: do they matter? Trends Plant Sci. 19:687–691. doi:10.1016/j.tplants.2014.09.001
- Shen, S., Li, N., Wang, Y., Zhou, R., Sun, P., Lin, H., Chen, W., Yu, T., Liu, Z., Wang, Z., Tan, X., Zhu, C., Feng, S., Zhang, Y., and Song, X. 2022. High‐quality ice plant reference genome analysis provides insights into genome evolution and allows exploration of genes involved in the transition from C3 to CAM pathways. Plant Biotechnol. J. 20:2107–2122. doi:10.1111/pbi.13892
- Shimony, C., Fahn, A., and Reinhold, L. 1973. Ultrastructure and ion gradients in the salt glands of Avicennia marina (Forssk.) Vierh. New Phytol. 72:27–36. doi:10.1111/j.1469-8137.1973.tb02006.x
- Singh, R. J. 2019. Cytogenetics and genetic introgression from wild relatives in soybean. Nucleus 62:3–14. doi:10.1007/s13237-019-00263-6
- Singh, S. P., Upadhyay, S. K., Pandey, A., and Kumar, S. 2019. Molecular approaches in plant biology and environmental challenges. In: Singh, S. P., Upadhyay, S. K., Pandey, A., and Kumar, S., Eds. Molecular Approaches in Plant Biology and Environmental Challenges. Springer: Singapore. pp 1–5.
- Sun, M., Zhang, Y., Zhu, L., Liu, N., Bai, H., Sun, G., Zhang, J., and Shi, L. 2022. Chromosome-level assembly and analysis of the Thymus genome provide insights into glandular secretory trichome formation and monoterpenoid biosynthesis in thyme. Plant Commun. 3:100413. doi:10.1016/j.xplc.2022.100413
- Szymanski, D. B., Lloyd, A. M., and Marks, M. D. 2000. Progress in the molecular genetic analysis of trichome initiation and morphogenesis in Arabidopsis. Trends Plant Sci. 5:214–219. doi:10.1016/s1360-1385(00)01597-1
- Tanaka, H., Hirakawa, H., Kosugi, S., Nakayama, S., Ono, A., Watanabe, A., Hashiguchi, M., Gondo, T., Ishigaki, G., Muguerza, M., Shimizu, K., Sawamura, N., Inoue, T., Shigeki, Y., Ohno, N., Tabata, S., Akashi, R., and Sato, S. 2016. Sequencing and comparative analyses of the genomes of zoysiagrasses. DNA Res. 23:171–180. doi:10.1093/dnares/dsw006
- Thomson, W. W., and Liu, L. L. 1967. Ultrastructural features of the salt gland of Tamarix aphylla L. Planta 73:201–220. doi:10.1007/BF00387033
- Thomson, W. W., Berry, W. L., and Liu, L. L. 1969. Localization and secretion of salt by the salt glands of Tamarix aphylla. Proc. Natl. Acad. Sci. USA 63:310–317. doi:10.1073/pnas.63.2.310
- Tominaga, R., Iwata, M., Okada, K., and Wada, T. 2007. Functional analysis of the epidermal-specific MYB genes CAPRICE and WEREWOLF in Arabidopsis. Plant Cell 19:2264–2277. doi:10.1105/tpc.106.045732
- Valliyodan, B., Cannon, S. B., Bayer, P. E., Shu, S., Brown, A. V., Ren, L., Jenkins, J., Chung, C. Y.-L., Chan, T.-F., Daum, C. G., Plott, C., Hastie, A., Baruch, K., Barry, K. W., Huang, W., Patil, G., Varshney, R. K., Hu, H., Batley, J., Yuan, Y., Song, Q., Stupar, R. M., Goodstein, D. M., Stacey, G., Lam, H.-M., Jackson, S. A., Schmutz, J., Grimwood, J., Edwards, D., and Nguyen, H. T. 2019. Construction and comparison of three reference‐quality genome assemblies for soybean. Plant J. 100:1066–1082. doi:10.1111/tpj.14500
- Wada, T., Tachibana, T., Shimura, Y., and Okada, K. 1997. Epidermal cell differentiation in Arabidopsis determined by a Myb homolog, CPC. Science 277:1113–1116. doi:10.1126/science.277.5329.1113
- Wang, S., and Chen, J.-G. 2014. Regulation of cell fate determination by single-repeat R3 MYB transcription factors in Arabidopsis. Front. Plant Sci. 5:133. doi:10.3389/fpls.2014.00133
- Wang, S., Kwak, S.-H., Zeng, Q., Ellis, B. E., Chen, X.-Y., Schiefelbein, J., and Chen, J.-G. 2007. TRICHOMELESS1 regulates trichome patterning by suppressing GLABRA1 in Arabidopsis. Development 134:3873–3882. doi:10.1242/dev.009597
- Wang, X., Zhou, Y., Xu, Y., Wang, B., and Yuan, F. 2021. A novel gene LbHLH from the halophyte Limonium bicolor enhances salt tolerance via reducing root hair development and enhancing osmotic resistance. BMC Plant Biol. 21:284. doi:10.1186/s12870-021-03094-3
- Werker, E. 2000. Trichome diversity and development. Adv. Bot. Res. 31:1–35.
- Xie, M., Chung, C. Y.-L., Li, M.-W., Wong, F.-L., Wang, X., Liu, A., Wang, Z., Leung, A. K.-Y., Wong, T.-H., Tong, S.-W., Xiao, Z., Fan, K., Ng, M.-S., Qi, X., Yang, L., Deng, T., He, L., Chen, L., Fu, A., Ding, Q., He, J., Chung, G., Isobe, S., Tanabata, T., Valliyodan, B., Nguyen, H. T., Cannon, S. B., Foyer, C. H., Chan, T.-F., and Lam, H.-M. 2019. A reference-grade wild soybean genome. Nat. Commun. 10:1216. doi:10.1038/s41467-019-09142-9
- Xu, Y., Jiao, X., Wang, X., Zhang, H., Wang, B., and Yuan, F. 2021. Importin-β from the recretohalophyte Limonium bicolor enhances salt tolerance in Arabidopsis thaliana by reducing root hair development and abscisic acid sensitivity. Front. Plant Sci. 11:582459. doi:10.3389/fpls.2020.582459
- Yamamoto, A., Hashiguchi, M., Akune, R., Masumoto, T., Muguerza, M., Saeki, Y., and Akashi, R. 2016. The relationship between salt gland density and sodium accumulation/secretion in a wide selection from three Zoysia species. Aust. J. Bot. 64:277–284. doi:10.1071/BT15261
- Yuan, F., and Wang, B. 2020. Adaptation of recretohalophytes to salinity. In Handbook of Halophytes: From Molecules to Ecosystems towards Biosaline Agriculture; Grigore, M.-N., Ed. Springer: Cham (Switzerland). pp 1–21.
- Yuan, F., Leng, B., Zhang, H., Wang, X., Han, G., and Wang, B. 2019. A WD40-repeat protein from the recretohalophyte Limonium bicolor enhances trichome formation and salt tolerance in Arabidopsis. Front. Plant Sci. 10:1456. doi:10.3389/fpls.2019.01456
- Yuan, F., Lyu, M. A., Leng, B., Zheng, G., Feng, Z., Li, P., Zhu, X., and Wang, B. 2015. Comparative transcriptome analysis of developmental stages of the Limonium bicolor leaf generates insights into salt gland differentiation. Plant. Cell Environ. 38:1637–1657. doi:10.1111/pce.12514
- Yuan, F., Wang, X., Zhao, B., Xu, X., Shi, M., Leng, B., Dong, X., Lu, C., Feng, Z., Guo, J., Han, G., Zhang, H., Huang, J., Chen, M., and Wang, B.-S. 2022. The genome of the recretohalophyte Limonium bicolor provides insights into salt gland development and salinity adaptation during terrestrial evolution. Mol. Plant. 15:1024–1044. doi:10.1016/j.molp.2022.04.011
- Yun, P., and Shabala, S. 2021. Ion transport in salt glands and bladders in halophyte species. In Handbook of Halophytes: From Molecules to Ecosystems towards Biosaline Agriculture; Grigore, M.-N., Ed. Springer: Cham (Switzerland). pp 1859–1876.
- Zhang, F., Gonzalez, A., Zhao, M., Payne, C. T., and Lloyd, A. 2003. A network of redundant bHLH proteins functions in all TTG1-dependent pathways of Arabidopsis. Development 130:4859–4869. doi:10.1242/dev.00681
- Zhang, Y., Mutailifu, A., and Lan, H. 2022. Structure, development, and the salt response of salt bladders in Chenopodium album L. Front. Plant Sci. 13:989946. doi:10.3389/fpls.2022.989946
- Zhao, B., Zhou, Y., Jiao, X., Wang, X., Wang, B., and Yuan, F. 2023. Bracelet salt glands of the recretohalophyte Limonium bicolor: Distribution, morphology, and induction. J. Integr. Plant Biol. 65:950–966. doi:10.1111/jipb.13417
- Zhao, C., Zhang, H., Song, C., Zhu, J.-K., and Shabala, S. 2020. Mechanisms of plant responses and adaptation to soil salinity. Innovation (Camb) 1:100017. doi:10.1016/j.xinn.2020.100017
- Zhao, M., Morohashi, K., Hatlestad, G., Grotewold, E., and Lloyd, A. 2008. The TTG1-bHLH-MYB complex controls trichome cell fate and patterning through direct targeting of regulatory loci. Development 135:1991–1999. doi:10.1242/dev.016873
- Zou, C., Chen, A., Xiao, L., Muller, H. M., Ache, P., Haberer, G., Zhang, M., Jia, W., Deng, P., Huang, R., Lang, D., Li, F., Zhan, D., Wu, X., Zhang, H., Bohm, J., Liu, R., Shabala, S., Hedrich, R., Zhu, J.-K., and Zhang, H. 2017. A high-quality genome assembly of quinoa provides insights into the molecular basis of salt bladder-based salinity tolerance and the exceptional nutritional value. Cell Res. 27:1327–1340. doi:10.1038/cr.2017.124
- Zou, H., Leng, B., Gao, Y., Wang, B., and Yuan, F. 2023. The MYB transcription factor LbCPC of Limonium bicolor negatively regulates salt gland development and salt tolerance. Environ. Exp. Bot 209:105310. doi:10.1016/j.envexpbot.2023.105310