ABSTRACT
Enzymes related to β-hydroxyacid dehydrogenases/3-hydroxyisobutyrate dehydrogenases are ubiquitous, but most of them have not been characterized. An uncharacterized protein with moderate sequence similarities to Gluconobacter oxydans succinic semialdehyde reductase and plant glyoxylate reductases/succinic semialdehyde reductases was found in the genome of Acetobacter aceti JCM20276. The corresponding gene was cloned and expressed in Escherichia coli. The gene product was purified and identified as a glyoxylate reductase that exclusively catalyzed the NAD(P)H-dependent reduction of glyoxylate to glycolate. The strict substrate specificity of this enzyme to glyoxylate, the diverged sequence motifs for its binding sites with cofactors and substrates, and its phylogenetic relationship to homologous enzymes suggested that this enzyme represents a novel class of enzymes in the β-hydroxyacid dehydrogenase family. This study may provide an important clue to clarify the metabolism of glyoxylate in bacteria.
Abbreviations: GR: glyoxylate reductase; GRHPR: glyoxylate reductase/hydroxypyruvate reductase; HIBADH: 3-hydroxyisobutyrate dehydrogenase; SSA: succinic semialdehyde; SSAR: succinic semialdehyde reductase
GRAPHICAL ABSTRACT
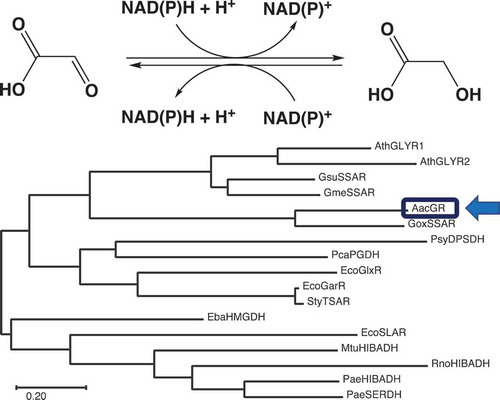
AceGR is NAD(P)H-dependent glyoxylate reductase representing a novel class of enzymes in the β-hydroxyacid dehydrogenase family.
Glyoxylate is an important intermediate in the microbial glyoxylate bypass pathway, which is required for growth on carbon sources such as acetate or fatty acids [Citation1]. Since glyoxylate is highly reactive, it is metabolized into less toxic intermediates by enzymatic processes in almost all organisms [Citation2]. Glyoxylate reductase (GR) catalyzes the reduction of glyoxylate to glycolate using either NADPH or NADH as a coenzyme. GRs are highly conserved and present in most organisms [Citation3–Citation6]. Most GRs also catalyze the conversion of hydroxypyruvate into d-glycerate [Citation4,Citation5]. Due to their overlapping substrate specificity and metabolic roles, such enzymes are called glyoxylate reductase/hydroxypyruvate reductase (GRHPR) [Citation7]. GRHPRs are categorized as belonging to the d-2-hydroxyacid dehydrogenase protein family [Citation8], which also includes d-lactate dehydrogenase [Citation9], d-glycerate dehydrogenase [Citation10], d-3-phosphoglycerate dehydrogenase [Citation11], and vancomycin resistance protein H [Citation12]. These class of enzymes show specificity for d-isomer of their substrates having 1-carboxy and 2-carbonyl/hydroxy groups.
Arabidopsis thaliana contains two isoforms of a distinct GR, namely cytosolic GLYR1 and plastidial/mitochondrial GLYR2. In contrast to GRHPR, these enzymes catalyze NADPH-dependent reduction of glyoxylate into glycolate, but have negligible activity toward hydroxypyruvate [Citation13]. They also convert succinic semialdehyde (SSA) into 4-hydroxybutyrate, albeit with lower catalytic efficiency [Citation14]. Thus, GLYR1 and GLYR2 are often termed plant GR/SSA reductases (GR/SSARs). Plant GR/SSARs are clearly distinct from GRHPRs in both their substrate specificities and structures, and belong to the 3-hydroxyisobutyrate dehydrogenase (HIBADH)-related enzyme family [Citation13]. The HIBADH-related family belongs to the β-hydroxyacid dehydrogenases (β-HAD) superfamily, a large superfamily of structurally and mechanistically related enzymes found in all three domains of life [Citation15,Citation16]. Enzymes in the β-HAD superfamily and those in the d-2-hydroxyacid dehydrogenase protein family are related one another in that both members utilize NAD(P) for catalysis, but little consensus is seen between the families in the NAD(P)-binding and the catalytic sequence motifs [Citation11,Citation15,Citation16]. Enzymes in the HIBADH-related family catalyze the NAD(P)-dependent oxidation/reduction of substrates containing β-hydroxy acid/β-aldehyde moieties. In addition to plant GR/SSARs, member of this family include HIBADH [Citation15], l-serine dehydrogenase [Citation17], and numerous uncharacterized homologs (approximately 7,200 sequences in the InterPro 79.0 database, IPR015815) [Citation18]. In addition, bacterial SSARs with high substrate specificity for SSA have been characterized from Geobacter spp. [Citation19]. and Gluconobacter oxydans 621H [Citation20]. Although members of this family generally exhibit promiscuous substrate selectivity, no GR activity has been reported for any HIBADH-related members except for plant GLYR1 and GLYR2 [Citation14]. Due to the diverse substrate specificities and primary structures of HIBADH-related enzymes, enzymatic characteristics of each HIBADH-related superfamily member need to be defined, along with their cofactor and substrate preferences.
An acetic acid bacterium, Acetobacter aceti JCM20276, possesses the tricarboxylic acid (TCA) cycle and the glyoxylate pathway implicated in acetic acid production [Citation21]. This bacterium also possesses two enzymatic activities, a membrane-bound glyoxylate dehydrogenase that acts specifically on glyoxylate to yield oxalic acid, and a soluble NAD-linked GR [Citation21]. However, neither of these enzymes has been purified to homogeneity, and the genes responsible for these enzyme activities have not been identified. By analyzing the genome of A. aceti JCM20276, we found the aac4036 gene coding for a putative enzyme, AAC4036, which exhibits moderate amino acid sequence similarities to the SSAR from G. oxydans [Citation20] and GR/SSARs from Arabidopsis [Citation14]. In this study, the predicted oxidoreductase, AAC4036, was overproduced in Escherichia coli, purified, and characterized. We showed that AAC4036 is an NAD(P)H-dependent GR with high substrate specificity for glyoxylate. The enzyme, named AacGR, exhibited no activity against SSA or hydroxypyruvate, and was markedly distinct from previously known GRHPRs and HIBADH-related GR/SSAR enzymes. The results of our phylogenetic analysis suggested that AacGR represents a new class of enzymes in the β-hydroxyacid dehydrogenases superfamily.
Materials and methods
Bacterial strains and culture conditions
A. aceti JCM20276 was provided by the RIKEN BRC through the National BioResource Project of the MEXT/AMED, Japan. A. aceti was grown in a lactate medium consisting of 0.6% sodium lactate, 0.3% yeast extract, and 0.2% polypepton. E. coli strains DH5α and BL21(DE3) were used for DNA manipulation and cloned gene overexpression, respectively. E. coli strains were grown aerobically at 37°C in lysogeny broth (LB) medium containing 100 µg/mL ampicillin.
Cloning of the AacGR gene, aac4036
A DNA fragment containing the aac4036 gene was amplified by polymerase chain reaction (PCR) with genomic DNA of A. aceti JCM20276 and the primers 5´-ATTACATATGGGACTTAGCAGCATGCAGATTC-3´ and 5´-TAATCTCGAGCTACGCCTTTAGCGATCGTTGG-3´. After digestion with NdeI and XhoI, the PCR product was ligated into pColdI (Takara Bio Inc., Shiga, Japan) to yield pAAC4036 encoding AAC4036 with a His-tag sequence at the N-terminal region. This construct was introduced into E. coli DH5α for plasmid manipulation.
Purification of AacGR
E. coli BL21(DE3) cells carrying pAAC4036 were cultured in LB medium supplemented with ampicillin (100 µg/mL) at 37°C. When the optical density at 600 nm (OD600) reached 0.5, aac4036 gene expression was induced with 1 mM isopropyl β-d-thiogalactopyranoside, and cells were cultured at 16°C overnight. The cells were harvested by centrifugation and resuspended in 50 mM Tris-HCl buffer (pH 8.0) containing 150 mM NaCl. The cells were disrupted by sonication for 10 min and centrifuged. The supernatant was then passed through a Ni-NTA column (GE Healthcare) equilibrated with the same buffer. The enzyme was eluted by a stepwise increase in the imidazole concentration (100 to 150 mM) in the same buffer. The enzyme fractions were then pooled and dialyzed with this buffer.
Enzyme assay
GR activity was assayed at 45°C in a reaction mixture containing 100 mM citrate buffer (pH 4.0), 1 mM glyoxylate, 0.15 mM NADH or NADPH, and 0.1–1 µg/mL of enzyme in a total volume of 1 mL. The absorbance at 340 nm was recorded during the reaction with a Shimadzu UV1800 spectrophotometer (Shimadzu, Kyoto, Japan). NAD(P)H-dependent reduction of a substrate by the enzyme was examined with various carboxylic acids, aldehydes, and dicarbonyls at pH of 4.0 and 6.0. NAD(P)+-dependent oxidation of a substrate by the enzyme was examined with various hydroxy acids and amino acids at 5 mM at pH of 4.0 (100 mM citrate buffer), 6.0 (100 mM citrate-phosphate buffer), 7.0 (100 mM citrate-phosphate buffer), and 9.0 (100 mM glycine–NaOH buffer) at 45°C. One unit of activity was defined as the amount of enzyme that catalyzed the reduction and the oxidation of one µmol of NAD(P)H and NAD(P)+, respectively, per min at 45°C. Protein was determined using a BCA Protein Assay Kit (Merck, Tokyo, Japan), with bovine serum albumin as a standard.
Effects of pH and temperature on enzyme activity
The effect of pH on enzyme activity was tested by determining the enzyme activity in a reaction mixture containing 100 mM buffer with pH varying between 3.0 and 11.0. The effect of pH on enzyme stability was examined by determining the remaining activity of the enzyme after incubation for 1 h on ice in 100 mM buffers with pH varying from 3.0 to 13.0. The effect of temperature on enzyme activity was determined at various temperatures from 25 to 70°C. The effect of temperature on enzyme stability was examined by determining the remaining activity of the enzyme after incubation in 100 mM citrate buffer (pH 4.0) for 10 min at various temperatures from 45 to 75°C.
Effects of metal ions, inhibitors, and organic solvents on enzyme stability
The enzyme was incubated on ice for 1 h in 100 mM citrate buffer (pH 4.0) containing metal ions or inhibitors at a final concentration of 1 mM before the assay. A portion (3 µL) of the incubated solution was added to the assay mixture with a total volume of 800 µL, which accordingly contained 0.00375 mM metal ions or inhibitors. To test the effects of organic solvents on enzyme activity, the enzyme was incubated on ice for 1 h in 100 mM citrate buffer (pH 4.0) containing 20–60% methanol, ethanol, or acetone, after which the remaining enzyme activity was determined in the same manner as described for the examination of the effects of metal ions or inhibitors on the enzyme.
Results and discussion
Bioinformatics analysis of AAC4036
Analysis of the genome sequence of A. aceti JCM20276 revealed that this bacterial strain had a gene, termed aac4036 (GenBank/EMBL/DDBJ accession no. LC549188), that was predicted to encode a putative NAD(P)-dependent oxidoreductase. The putative gene product, AAC4036, showed the highest sequence identity (54%) with G. oxydans SSAR (GoxSSAR) [Citation20] among the biochemically characterized enzymes. GoxSSAR exhibits SSAR activity, with low activity toward glyoxal, phenylglyoxal, and methylglyoxal [Citation20]. AAC4036 also shared slight to moderate amino acid sequence identities with enzymes belonging to the HIBADH-related superfamily, such as Geobacter sulfurreducens SSARs (30%) [Citation19,Citation20], A. thaliana GLYRs (27%) [Citation14], Eubacterium barkeri 2-(hydroxymethyl)glutarate dehydrogenase (27%), and Pyrobaculum calidifontis 6-phosphogluconate dehydrogenase (26%) [Citation22] (). This implied that AAC4036 is a member of the HIBADH-related enzyme superfamily. InterProScan Search [Citation18] (https://www.ebi.ac.uk/interpro/) results also suggested that AAC4036 belongs to the HIBADH-related family (IPR015815). We selected a few representative proteins from each class, including biochemically characterized enzymes, to analyze the phylogeny of AAC4036 (). AAC4036 and GoxSSAR clearly belonged to a distinct clade and were the most divergent from the HIBADH clade. Since the AAC4036 and GoxSSAR clade was related to the clade containing plant GLYRs and Geobacter SSARs, we hypothesized that AAC4036 was likely to catalyze the reduction of an aldehyde substrate.
Figure 1. Sequence analysis of Acetobacter aceti glyoxylate reductase (AacGR/AAC4036) and β-hydroxyacid dehydrogenases (β-HADs). (a) Amino acid sequence alignment of AacGR. (b) Phylogenetic tree of AacGR (LC549188) and related enzymes. Multiple alignments were performed using Multalin [Citation23] and rendered using ESPript [Citation24]. Residues conserved in all sequences are shown with reversed text. Asterisks indicate functionally important residues [Citation16]. Phylogenetic analyses were conducted in MEGA X [Citation25]. Proteins used for analyses were: GoxSSAR, G. oxydans SSAR (Q5FQ06); AthGLYR1, A. thaliana GR/SSAR1 (Q9LSV0); AthGLYR2, A. thaliana GR/SSAR2 (F4I907); GsuSSAR, G. sulfurreducens SSAR (Q74DE4); GmeSSAR, G. metallireducens SSAR (Q39R98); EcoGarR, Escherichia coli NADPH-dependent tartronate semialdehyde reductase (CZAR) (P0ABQ2); EcoGlxR, E. coli NADH-dependent CZAR (P77161); EbaHMGDH, E. barkeri 2-(hydroxymethyl)glutarate dehydrogenase (Q0QLF5); PcaPGDH, Pyrobaculum calidifontis 6-phosphogluconate dehydrogenase (A3MU08); EcoSLAR, E. coli 3-sulfolactaldehyde reductase (P0A9V8); PsyDPSDH, Pseudomonas syringae d-phenylserine dehydrogenase (E5RM11); StyTSAR, Salmonella typhi CZAR (Q8Z3K1); MtuHIBADH, 3-hydroxyisobutyrate dehydrogenase (HIBADH) (P9WNY5); RnoHIBADH, Rattus norvegicus HIBADH (P29266); PaeHIBADH, Pseudomonas aeruginosa HIBADH (P28811); and PaeSERDH, P. aeruginosa NAD-dependent l-serine dehydrogenase (Q9I5I6).
![Figure 1. Sequence analysis of Acetobacter aceti glyoxylate reductase (AacGR/AAC4036) and β-hydroxyacid dehydrogenases (β-HADs). (a) Amino acid sequence alignment of AacGR. (b) Phylogenetic tree of AacGR (LC549188) and related enzymes. Multiple alignments were performed using Multalin [Citation23] and rendered using ESPript [Citation24]. Residues conserved in all sequences are shown with reversed text. Asterisks indicate functionally important residues [Citation16]. Phylogenetic analyses were conducted in MEGA X [Citation25]. Proteins used for analyses were: GoxSSAR, G. oxydans SSAR (Q5FQ06); AthGLYR1, A. thaliana GR/SSAR1 (Q9LSV0); AthGLYR2, A. thaliana GR/SSAR2 (F4I907); GsuSSAR, G. sulfurreducens SSAR (Q74DE4); GmeSSAR, G. metallireducens SSAR (Q39R98); EcoGarR, Escherichia coli NADPH-dependent tartronate semialdehyde reductase (CZAR) (P0ABQ2); EcoGlxR, E. coli NADH-dependent CZAR (P77161); EbaHMGDH, E. barkeri 2-(hydroxymethyl)glutarate dehydrogenase (Q0QLF5); PcaPGDH, Pyrobaculum calidifontis 6-phosphogluconate dehydrogenase (A3MU08); EcoSLAR, E. coli 3-sulfolactaldehyde reductase (P0A9V8); PsyDPSDH, Pseudomonas syringae d-phenylserine dehydrogenase (E5RM11); StyTSAR, Salmonella typhi CZAR (Q8Z3K1); MtuHIBADH, 3-hydroxyisobutyrate dehydrogenase (HIBADH) (P9WNY5); RnoHIBADH, Rattus norvegicus HIBADH (P29266); PaeHIBADH, Pseudomonas aeruginosa HIBADH (P28811); and PaeSERDH, P. aeruginosa NAD-dependent l-serine dehydrogenase (Q9I5I6).](/cms/asset/01de94e3-a6b3-4d46-b814-72a6a887917b/tbbb_a_1797470_f0001_b.gif)
Heterologous expression and purification of AAC4036
To characterize the catalytic properties of AAC4036, A. aceti AAC4036 with an N-terminal His-tag was produced in E. coli BL21(DE3) cells harboring the pAAC4036 construct and purified by Ni-NTA column chromatography. Analysis of the soluble crude cell extract by sodium dodecyl sulfate-polyacrylamide gel electrophoresis (SDS-PAGE) showed that the overproduction of AAC4036 was achieved, with the protein produced having an approximate molecular mass of 33 kDa, which was consistent with the theoretical molecular mass (32.8 kDa) calculated from the amino acid sequence (). AAC4036 was purified to homogeneity, based on a single band occurring on the SDS-PAGE gel (). During purification, we found that AAC0436 catalyzed glyoxylate reduction while using NADPH as a cofactor. We obtained 6.5 mg of the enzyme with a specific activity of 1300 units/mg (2.8-fold purity, with a recovery rate of 119%) ().
Table 1. Purification of the recombinant AacGR (AAC4036).
Figure 2. Analysis by SDS-PAGE of the AAC4036 (AacGR) protein purified from recombinant E. coli cells. Lanes 1, 2, and 3 indicate the size marker proteins, crude extract, and enzyme purified by Ni-affinity chromatography, respectively. The numbers on the left indicate the molecular masses of the marker proteins.

Substrate specificity
We initially hypothesized that AAC0436 might utilize SSA as a substrate because it shared 54% amino acid sequence identity with G. oxydans SSAR. However, we found that AAC0436 showed no activity toward SSA. When glyoxylate was tested as a substrate, the enzyme exhibited markedly high activity in the presence of NADPH or NADH as an electron donor. The specific activities of the enzyme with NADH (1,150 units/mg) and NADPH (1,300 units/mg) were similar, but slightly higher with NADPH. NAD(P)H-dependent substrate reduction by the enzyme was not observed with other small molecules, such as carboxylic acids (formate, acetate, oxalate, 3-hydroxypropionate, dl-glycerate, pyruvate, hydroxypyruvate, and phenylpyruvate), aldehydes (formaldehyde, acetaldehyde, glutaraldehyde, and succinic semialdehyde), and dicarbonyls (glyoxal, methylglyoxal, and phenylglyoxal). We also examined NAD(P)+-dependent glycolate oxidation by the enzyme. The enzyme showed no detectable activity for the reverse reaction at acidic (pH 4.0) and neutral (pH 7.0) pH conditions, whereas it exhibited a specific activity of 1.1 units/mg (with NAD+) and 2.4 units/mg (with NADP+) when tested at pH 9.0 with 50 mM glycolate. The specific activity of the enzyme for the forward reaction at pH 9.0 was determined to be 540 units/mg with 1 mM glyoxylate and NADPH. Therefore, the enzyme appeared to favor the forward reaction not only at pH 4.0 but also at pH 7.0 and 9.0. In contrast, all of the other hydroxy acids (dl-lactate, l-malate, (S)-hydroxyisobutyrate, and (R)-hydroxyisobutyrate) and amino acids (d-serine, l-serine, d-threonine, and l-threonine) were inert as substrates of the enzyme when examined at pH of 4.0, 6.0, and 9.0. These results suggest that AAC0436 is a distinctive GR (hereinafter called AacGR) that exclusively catalyzes the reversible NAD(P)H-dependent reduction of glyoxylate to glycolate.
Effect of pH on activity and stability of AacGR
The effect of pH on AacGR activity was examined over the pH range of 3.0–11.0 (). The maximum activity was observed at a pH of 4.0, with approximately 50% of this activity retained at a pH of 3.5 and 8.0, respectively. The enzyme activity was significantly low in Tris–HCl buffer (pH 7 and 8), which might be due to an amino group of Tris reacting with the substrate aldehyde. The optimal pH of the reaction catalyzed by AacGR was lower than that of most known GRs/GRHPRs from plants [Citation13], fungi [Citation26], bacteria [Citation27], and archaea [Citation6], which have an optimum pH around 6–7, but it was comparable with that of the GR from Chlamydomonas reinhardtii, which exhibits relatively high activity at a pH of around 5.0 [Citation28]. AacGR exhibited a high pH stability, such that more than 80% of the activity was retained over the pH range of 4.0–11.0 for 1 h on ice (). The fungal GR from Paecilomyes thermophila also shows a broad pH stability at pH of 4.5–10.0 [Citation26].
Figure 3. Effect of pH on (a) activity and (b) stability of AacGR. Effect of temperature on (c) activity and (d) stability of AacGR. Buffers used in (a) and (b) were: citrate, pH 3–5 (closed circles); citrate-phosphate, pH 4–7 (closed squares); phosphate, pH 6–7 (closed triangles); Tris–HCl, pH 7–8 (closed diamonds); glycine–NaOH, pH 8–11 (asterisks); and KCl-NaOH, pH 12–13 (crosses).

Effect of temperature on activity and stability of AacGR
The effects of temperature on the activity of AacGR were examined from 25 to 70°C (). AacGR showed relatively high activity over a broad temperature range (30–45°C), with maximum activity at 45°C. The enzyme was stable up to 65°C for 10 min and inactivated rapidly when the temperature exceeded 70°C (). In contrast, A. thaliana GLYR1 is heat-labile and has an optimum reaction temperature of 22°C [Citation13]. AacGR retained about 90% of its activity after 10-min incubation at 65°C, at which temperature it did not show activity in the reaction mixture. This observed discrepancy may be related to the reversible nature of the enzyme denaturation by heat, because the heat-treated enzyme was cooled on ice until the assay.
Kinetic analysis of AacGR
Kinetic constants of the AacGR-catalyzed reaction of glyoxylate were investigated using NADH or NADPH as a cofactor. The Km values of AacGR for glyoxylate in the presence of NADH and NADPH were 0.58 and 0.38 mM, respectively (). The Vmax value of AacGR for glyoxylate in the presence of NADPH (1,020 µmol·min−1·mg−1) was comparable to or slightly higher than that in the presence of NADH (951 µmol·min−1·mg−1). Kinetic constants of the enzyme for the reverse reaction (NAD(P)+-dependent oxidation of glycolate) were also determined. In this case, the enzyme exhibited markedly high Km values (> 300 mM) for glycolate using either cofactor (). The Vmax values of AacGR for glycolate were markedly lower than those found for glyoxylate. The catalytic efficiency (kcat/Km) of AacGR for glyoxylate with NADPH was 5 × 104 times higher than that for glycolate with NADP+. These results suggest a clear preference of AacGR for NAD(P)H-dependent glyoxylate reduction. The high catalytic efficiency of AacGR is comparable to that of the GR from Thermus thermophilus HB27 [Citation27].
Table 2. Kinetic parameters of AacGR*.
Effects of metal ions, chemical compounds, and organic solvents on stability of AacGR
We examined the effects of various metal ions and chemical compounds on the stability of AacGR by incubating the enzyme for 1 h on ice in the presence of the additives. Most metal ions did not affect AacGR stability, except that Fe3+and Hg2+ decreased enzyme activity by about 15 and 30%, respectively (Supplementary Figure S1). On the other hand, enzyme activity was decreased to 20% by incubation with 60% ethanol (Supplementary Figure S1).
Comparison of AacGR with other related enzymes
In this study, we showed that AacGR exclusively catalyzes glyoxylate reduction using NADPH or NADH as a cofactor. To the best of our knowledge, there is no other example of a glyoxylate-specific NAD(P)-dependent reductase. Most GRs are GRHPR-type enzymes that have broad substrate specificity, most commonly to hydroxypyruvate, and belong to the d-2-hydroxyacid dehydrogenase family [Citation6–Citation8], which is structurally and functionally distinct from the HIBADH family. Meanwhile, the HIBADH-related family of enzymes generally acts on the common 3-hydroxypropionate moiety of their substrates (in addition to HIBA), including d/l-glycerate [Citation29], l-serine [Citation17], and d-phenylserine [Citation22], to perform NAD(P)+-dependent oxidation. Therefore, AacGR and the closely related SSARs from G. oxydans [Citation20] and Geobacter spp [Citation19]. and the plant GLYRs [Citation14] differ from typical HIBADH-related enzymes in that they preferentially act on the aldehyde group at the C2- and C4-positions in their substrates (glyoxylate and SSA, respectively) to perform NAD(P)H-dependent reduction.
Enzymes in the β-HAD superfamily are typically around 300 amino acids in length, and contain four conserved regions: the N-terminal dinucleotide cofactor-binding, substrate-binding, catalysis, and second cofactor-binding regions [Citation16] (). The multiple sequence alignment of AacGR with several representative enzymes from each class of the β-HAD superfamily revealed that AacGR and GoxSSAR have diverged cofactor- and substrate-binding sequences that differ from those of most members of the HIBADH-related family (). The amino acid sequence of residues 14–27 in AacGR is “FIGFGAMASRMGDH”, while the sequence of the “HIBADH signature” is “[LIVMFY](2)GLGX[MQ]GXX[MA][SAV]X[SNHR]” (accession no. PS00895 in the PROSITE database, https://prosite.expasy.org/prosite.html) [Citation16,Citation30]. Specificity to NAD or NADP is known to depend on residues 18–20 on the C-terminal side of the conserved GLGX[MQ]G motif [Citation15]. In the majority of family members, the NAD-dependent enzyme contains the DXX motif, in contrast to the NRX motif in the NADP-dependent enzyme in the same region [Citation15] (). However, AacGR and GoxSSAR contain the “TPS” and “APS” motifs, respectively, in the corresponding position in their amino acid sequences. The absence of either Asp or Arg at this position in AacGR and GoxSSAR is consistent with their unusual dual cofactor specificity to NADH and NADPH, which is atypical for the β-HAD superfamily. In the substrate-binding consensus region, AacGR, GoxSSAR, GmeSSAR, GLYR1, and GLYR2 have the “SGS triad” motif in place of the highly conserved “SGG triad” found in typical β-HAD superfamily members, which provides a binding site for a carbonyl group in their substrates.
Among the biochemically characterized enzymes, AacGR shares the highest sequence identity (54%) with GoxSSAR. Unfortunately, the activity of GoxSSAR toward glyoxylate is unknown, but AacGR is at least distinct from GoxSSAR in that AacGR does not utilize SSA as a substrate, while GoxSSAR prefers to act on SSA [Citation20]. Because of the typically wide substrate diversity of β-HADs and the observation that multiple homologs of them are present in the genomes of various species, some β-HAD superfamily members may have species-specific metabolic roles. There are marked differences in glyoxylate-related metabolism between A. aceti and G. oxydans: A. aceti JCM20276 and NBRC14818 [Citation31] contains genes encoding isocitrate lyase (aceA) and malate synthase (glcB) in the glyoxylate pathway, whereas several TCA cycle and glyoxylate pathway genes are missing in G. oxydans [Citation32]. Therefore, it is not surprising that AacGR exhibits distinct substrate specificity from that of GoxSSAR, even though both A. aceti and G. oxydans are “acetic acid bacteria”. When the sequences of close, uncharacterized homologs of AacGR and GoxSSAR were compared, AacGR was assigned to a clade phylogenetically distinct from that of GoxSSAR (Supplementary Figure S2). Based on these findings, we propose that AacGR constitute a new class of enzyme in the β-HAD superfamily. The metabolic function of AacGR is unknown in A. aceti. Our finding of the glyoxylate specificity of AacGR may provide an important clue to clarify bacterial glyoxylate metabolism. Future research on the relationship between the structure and function of these enzyme will clarify the mechanisms of their substrate selectivity.
Author contribution
JK, RT, and HM conceived this work and designed the experiments. JK performed the experiments and analyzed the data together with RT and HM. TK provided valuable advices. TK, YH, and TO helped acquire specialized experimental data. JK and HM wrote the manuscript. All authors contributed to and approved the final version.
SupplementaryFigs0715.pdf
Download PDF (119.7 KB)Acknowledgments
The authors would like to thank Ms. K. Kitayama for technical assistance.
Disclosure statement
No potential conflict of interest was reported by the authors.
Supplementary material
Supplemental data for this article can be accessed here.
Additional information
Funding
References
- Kornberg HL. The role and control of the glyoxylate cycle in Escherichia coli. Biochem J. 1966;99:1–11.
- Puckett S, Trujillo C, Wang Z, et al. Glyoxylate detoxification is an essential function of malate synthase required for carbon assimilation in Mycobacterium tuberculosis. Proc Natl Acad Sci U S A. 2017;114:E2225–E2232.
- Rumsby G, Cregeen DP. Identification and expression of a cDNA for human hydroxypyruvate/glyoxylate reductase. Biochim Biophys Acta. 1999;1446:383–388.
- Greenler JM, Sloan JS, Schwartz BW, et al. Isolation, characterization and sequence analysis of a full-length cDNA clone encoding NADH-dependent hydroxypyruvate reductase from cucumber. Plant Mol Biol. 1989;13:139–150.
- Yoshida T, Yamaguchi K, Hagishita T, et al. Cloning and expression of the gene for hydroxypyruvate reductase (D-glycerate dehydrogenase from an obligate methylotroph Hyphomicrobium methylovorum GM2. Eur J Biochem. 1994;223:727–732.
- Ohshima T, Nunoura-Kominato N, Kudome T, et al. A novel hyperthermophilic archaeal glyoxylate reductase from Thermococcus litoralis. Characterization, gene cloning, nucleotide sequence and expression in Escherichia coli. Eur J Biochem. 2001;268:4740–4747.
- Booth MP, Conners R, Rumsby G, et al. Structural basis of substrate specificity in human glyoxylate reductase/hydroxypyruvate reductase. J Mol Biol. 2006;360:178–189.
- Kutner J, Shabalin IG, Matelska D, et al. Structural, biochemical, and evolutionary characterizations of glyoxylate/hydroxypyruvate reductases show their division into two distinct subfamilies. Biochemistry. 2018;57:963–977.
- Taguchi H, Ohta T. d-Lactate dehydrogenase is a member of the d-isomer-specific 2-hydroxyacid dehydrogenase family. Cloning, sequencing, and expression in Escherichia coli of the d-lactate dehydrogenase gene of Lactobacillus plantarum. J Biol Chem. 1991;266:12588–12594.
- Goldberg JD, Yoshida T, Brick P. Crystal structure of a NAD-dependent d-glycerate dehydrogenase at 2.4 Å resolution. J Mol Biol. 1994;236:1123–1140.
- Grant GA. A new family of 2-hydroxyacid dehydrogenases. Biochem Biophys Res Commun. 1989;165:1371–1374.
- Arthur M, Molinas C, Dutka-Malen S, et al. Structural relationship between the vancomycin resistance protein VanH and 2-hydroxycarboxylic acid dehydrogenases. Gene. 1991;103:133–134.
- Hoover GJ, Van Cauwenberghe OR, Breitkreuiz KE, et al. Characteristics of an Arabidopsis glyoxylate reductase: general biochemical properties and substrate specificity for the recombinant protein, and developmental expression and implications for glyoxylate and succinic semialdehyde metabolism in planta. Can J Bot. 2007;85:883–895.
- Zarei A, Brikis CJ, Bajwa VS, et al. Plant glyoxylate/succinic semialdehyde reductases: comparative biochemical properties, function during chilling stress, and subcellular localization. Front Plant Sci. 2017;8:1399.
- Hawes JW, Harper ET, Crabb DW, et al. Structural and mechanistic similarities of 6-phosphogluconate and 3-hydroxyisobutyrate dehydrogenases reveal a new enzyme family, the 3-hydroxyacid dehydrogenases. FEBS Lett. 1996;389:263–267.
- Njau RK, Herndon CA, Hawes JW. New developments in our understanding of the β-hydroxyacid dehydrogenases. Chem Biol Interact. 2001;130-132:785–791.
- Tchigvintsev A, Singer A, Brown G, et al. Biochemical and structural studies of uncharacterized protein PA0743 from Pseudomonas aeruginosa revealed NAD+-dependent l-serine dehydrogenase. J Biol Chem. 2012;287:1874–1883.
- Mitchell AL, Attwood TK, Babbitt PC, et al. InterPro in 2019: improving coverage, classification and access to protein sequence annotations. Nucleic Acids Res. 2019;47:D351–D360.
- Zhang Y, Gao X, Zheng Y, et al. Identification of succinic semialdehyde reductases from Geobacter: expression, purification, crystallization, preliminary functional, and crystallographic analysis. Acta Biochim Biophys Sin (Shanghai). 2011;43:996–1002.
- Meyer M, Schweiger P, Deppenmeier U. Succinic semialdehyde reductase Gox1801 from Gluconobacter oxydans in comparison to other succinic semialdehyde-reducing enzymes. Appl Microbiol Biotechnol. 2015;99:3929–3939.
- Kasai T, Suzuki I, Asai T. Glyoxylate oxidation in Acetobacter with reference to the formation of oxalic acid. J Gen Appl Microbiol. 1963;9:49–58.
- Ueshima S, Muramatsu H, Nakajima T, et al. Identification, cloning, and characterization of l-phenylserine dehydrogenase from Pseudomonas syringae NK-15. Enzyme Res. 2010;2010:597010.
- Corpet F. Multiple sequence alignment with hierarchical clustering. Nucleic Acids Res. 1988;16:10881–10890.
- Robert X, Gouet P. Deciphering key features in protein structures with the new ENDscript server. Nucleic Acids Res. 2014;42:W320–324.
- Kumar S, Stecher G, Li M, et al. MEGA X: molecular evolutionary genetics analysis across computing platforms. Mol Biol Evol. 2018;35:1547–1549.
- Duan X, Hu S, Zhou P, et al. Characterization and crystal structure of a first fungal glyoxylate reductase from Paecilomyes thermophila. Enzyme Microb Technol. 2014;60:72–79.
- Ogino H, Nakayama H, China H, et al. Characterization of recombinant glyoxylate reductase from thermophile Thermus thermophilus HB27. Biotechnol Prog. 2008;24:321–325.
- Husic DW, Tolbert NE. NADH:hydroxypyruvate reductase and NADPH: glyoxylatereductase in algae: partial purification and characterization from Chlamydomonas reinhardtii. Arch Biochem Biophys. 1987;252:396–408.
- Hubbard BK, Koch M, Palmer DR, et al. Evolution of enzymatic activities in the enolase superfamily: characterization of the d-glucarate/galactarate catabolic pathway in Escherichia coli. Biochemistry. 1998;37:14369–14375.
- Sigrist CJ, de Castro E, Cerutti L, et al. New and continuing developments at PROSITE. Nucleic Acids Res. 2013;41:D344–347.
- Sakurai K, Arai H, Ishii M, et al. Transcriptome response to different carbon sources in Acetobacter aceti. Microbiology. 2011;157:899–910.
- Prust C, Hoffmeister M, Liesegang H, et al. Complete genome sequence of the acetic acid bacterium Gluconobacter oxydans. Nat Biotechnol. 2005;23:195–200.