ABSTRACT
Selenium (Se) causes oxidative damage to plants. Proline is accumulated as a compatible solute in plants under stress conditions and mitigates stresses. Selenate at 250 µM increased cell death and inhibited the growth of tobacco BY-2 cells while exogenous proline at 10 mM did not mitigate the inhibition by selenate. Selenate increased accumulation of Se and ROS and activities of antioxidant enzymes but not lipid peroxidation in the BY-2 cells. Proline increased Se accumulation and antioxidant enzyme activities but not either ROS accumulation or lipid peroxidation in the selenate-stressed cells. Glutathione (GSH) rather than ascorbic acid (AsA) mitigated the growth inhibition although both reduced the accumulation of ROS induced by selenate. These results indicate that proline increases both antioxidant enzyme activities and Se accumulation, which overall fails to ameliorate the growth inhibition by selenate and that the growth inhibition is not accounted for only by ROS accumulation.
Abbreviations: APX: ascorbate peroxidase; AsA: ascorbic acid; BY-2: Bright Yellow-2; CAT: catalase; DAI: days after inoculation; DW: dry weight; FW: fresh weight; GSH: glutathione; ROS: reactive oxygen species
GRAPHICAL ABSTRACT
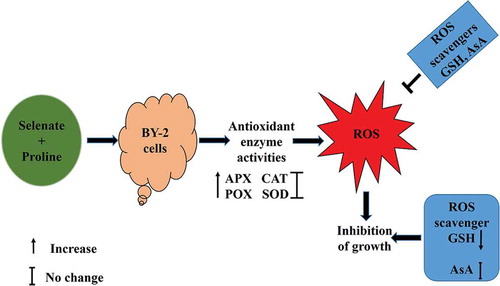
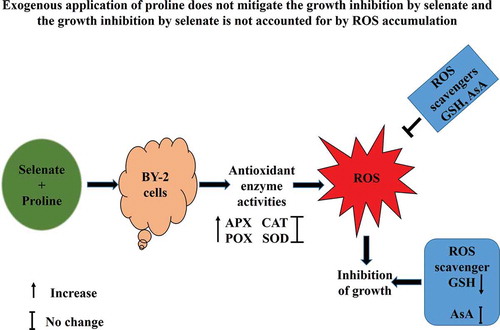
Exogenous application of proline does not mitigate the growth inhibition by selenate and the growth inhibition by selenate is not accounted for by ROS accumulation.
Selenium (Se) is a micro-nutrient for animals but not for most plants [Citation1,Citation2] and Se concentrations at higher than 100 µM can be hazardous for all biotas including plants and animals [Citation3]. In human, adequate level of Se has an important role in antioxidant selenoproteins for protection against oxidative stress while high dose of Se shows alkali diseases and disorders of nervous system [Citation4,Citation5]. In plants, lower concentration of Se has been shown to have positive effects on stress tolerance. For example, exogenous application of selenium alleviates cadmium-induced oxidative stress in tomato (Solanum lycopersicum L.) plants by enhancing antioxidant system [Citation6] and salinity-induced damage in rapeseed (Brassica napus) seedlings [Citation7]. In contrast, high concentration (>100 µM) of Se is toxic for plants as well as animals [Citation3,Citation8].
Selenium toxicity occurs in plants for its chemical similarity with sulfur. Substitution of cysteine residues and methionine residue by selenocystein residue and selenomethionine residue adversely affects the activities of proteins, which result in mimic sulfur starvation such as withering, drying, and premature death of leaves [Citation9–11]. Therefore, it is crucial to understand the mechanisms of growth inhibition by selenium in plants in order to develop approaches to alleviate Se stress.
Proline is a bioactive molecule that functions as an osmoprotectant. It is well known that proline accumulates in plants during various stresses [Citation12–15]. Heavy metal toxicity and plant–pathogen interaction also cause proline accumulation in plants [Citation16,Citation17]. Supplementation of proline can improve tolerance to a variety of environmental stresses in plants [Citation18]. In the case of tobacco Bright Yellow-2 (BY-2) cells, the growth inhibition by salt or cadmium (Cd) was alleviated by supplementation of proline at 10 mM [Citation19–Citation22]. It has been stated that proline plays a role as an enzyme protectant and reactive oxygen species (ROS) scavengers [Citation20,Citation23–25]. However, growth inhibition by arsenate was alleviated by proline only at lower concentrations in BY-2 cells [Citation26].
A variety of toxic metals can induce ROS accumulation in the plant tissues. The ROS accumulation is responsible for damaging biological molecules such as proteins, lipids, DNA, and carbohydrates [Citation27–31]. Selenium functions as a pro-oxidant at high doses, that is, selenium induces ROS production, which triggers oxidative stress in plants. In addition, ROS also acts as a key signaling molecule to mediate many physiological processes in plants such as metabolism, growth, and development, and response to different stress [Citation32,Citation33].
Plants have enzymatic and non-enzymatic antioxidant defense systems, which play an important role to detoxify the produced ROS. In plant cells, ascorbic acid (AsA) is a key non-enzymatic antioxidant that can directly scavenge ROS such as H2O2, O2•−, OH·, and 1O2 and plays an important role in ascorbate-glutathione (AsA-GSH) cycle for scavenging H2O2. Glutathione (GSH) is another important non-enzymatic antioxidant in plant cells that also either directly or indirectly scavenges a range of ROS such as H2O2, OH·, and 1O2 [Citation27,Citation34,Citation35]. In the AsA-GSH cycle, ascorbate peroxidase oxidizes AsA to produce monodehydroascorbate (MDHA) to detoxify H2O2 and monodehydroascorbate reductase (MDAR) mediates regeneration of AsA from MDHA using NADH. The MDHA can disproportionate into ascorbate and dehydroascorbate (DHA) when MDHA is not immediately reduced to AsA. Dehydroascorbate reductase (DHAR) reduces DHA to AsA using reduced GSH. The subsequent oxidized GSH (GSSG) is converted to reduced GSH by NADPH-dependent glutathione reductase (GR).
Tobacco is an annual crop, which is commercially cultivated in many countries and tobacco BY-2 cells have been widely used in many biochemical studies because they are highly homogenous and can grow rapidly in suitable culture medium. Exogenous proline reduces intracellular ROS accumulation and oxidative damage by increasing antioxidant enzyme activities in tobacco BY-2 cells under Na and Cd stress [Citation21,Citation22]. Moreover, in some cases, the exogenously applied proline is toxic to plants [Citation36]. However, it remains to be clarified whether the exogenous application of proline can mitigate inhibition of growth by selenate in BY-2 cells. Therefore, the effects of exogenous proline on growth, antioxidant enzyme activities, intracellular ROS accumulation, and level of oxidative damage in tobacco BY-2 cultured cells under selenate stress were investigated.
Materials and methods
Cell cultures of tobacco BY-2
Tobacco (Nicotiana tabacum L., cv. BY-2) suspension-cultured cells were employed as a cell line that is unadapted to selenium stress [Citation37,Citation38]. The modified LS medium [Citation39] was used as a control medium. Selenium medium was prepared by supplementation with 250 μM Na2SeO4 in the control medium. In order to examine proline effects, the control medium and the selenium medium supplemented with 5 mM or 10 mM proline were used. The cell cultures were performed and managed, as previously described [Citation37,Citation38].
Assay of BY-2 cell growth
Two-gram cells were collected from 7 days old suspension culture and inoculated in fresh medium. The medium was cultured on a rotary shaker (100 rpm, 25°C). The fresh weight (FW) of BY-2 was measured at 0, 4, 6, and 8 days after inoculation as described previously [Citation37,Citation38]. The fresh BY-2 cells were oven-dried at 70°C and the dry weight (DW) was measured after 24 h.
Estimation of cell death of BY-2 cells
Dead cells were estimated by the following procedure of Nahar et al. [Citation26]. Cells were stained with Evans Blue solution (0.05%) for 20 min to load the dye. After that, the excess dye was washed out with distilled water. Dye-bounded dead cells were solubilized in 1 mL of 50% methanol that contained 1% sodium dodecyl sulfate followed by the incubation for 50 min at 50°C. Then the absorbance was measured at 600 nm by a spectrophotometer. For calculation, the cells prepared in the same way were subjected to two cycles of freezing at −20°C and thawing at room temperature. The cells killed in such a way were used to define 100% cell death. The value obtained from 2-day-old and 4-day-old cultured cells was defined as 0% cell death. The death cells were calculated by comparing the absorbance of the samples with the absorbance of 100% cell death.
Intracellular ROS detections
Intracellular ROS accumulation in BY-2 cells was analyzed by using 2´,7´-dichlorodihydrofluorescein diacetate (H2DCF-DA) as described previously [Citation40]. Four-day-old BY-2 cells were collected with brief centrifugation by washing twice with phosphate-buffered saline (PBS). Then the collected cells were incubated in PBS supplemented with 20 μM H2DCF-DA for 30 min at 30°C. After that the incubated cells were washed two times with PBS and fluorescence images were captured using a fluorescence microscope (Biozero, Keyence, Japan) and analyzed using Image-J software (NIH, USA).
Determination of lipid peroxidation
Lipid peroxidation was evaluated by measuring malondialdehyde (MDA) contents, as previously described by Okuma et al. [Citation41]. One-gram of BY-2 cells was grounded in mortar and pestle using liquid nitrogen (N2) and 2 mL of 5% (w/v) trichloroacetic acid was added to homogenize the mixture. Then the homogenized mixture was centrifuged at 12,000 g for 15 min. After that, the supernatant was collected and diluted twice with 20% (w/v) trichloroacetic acid containing 0.5% (w/v) thiobarbituric acid. Then the mixture was heated at 95°C for 25 min. After cooled in an ice bath, the mixture was centrifuged at 7500 g for 5 min. At 532 nm, the absorbance of the samples was measured and nonspecific turbidity was compensated by subtracting absorbance at 600 nm. MDA content was calculated using extinction of the coefficient of 155 mM−1cm−1.
Measurement of antioxidant enzyme activities
Catalase activity
Catalase (CAT; EC: 1.11.1.16) activity was analyzed by the following procedure of Islam et al. [Citation22]. A reaction mixture was prepared which contained 50 mM Tris-HCl buffer (pH 8.0), 0.25 mM EDTA, 20 mM H2O2, and 25 μL of the sample solution. The catalase activity was calculated from the decrease in absorbance at 240 nm for 120 s when the extinction coefficient was 40 M−1 cm−1
APX activity
Ascorbate peroxidase (APX; EC 1.11.1.11) activity was determined by the following procedure of Ishikawa et al. [Citation42]. The reaction buffer solution contained 50 mM KH2PO4 buffer (pH 7.0), 0.1 mM EDTA, 0.1 mM H2O2, and 0.5 mM AsA. The reaction was started by adding the sample solution to the reaction buffer solution. The oxidation of ascorbate was followed by a decrease in absorbance at 290 nm for 60 s, where the extinction coefficient was 2.80 mM−1 cm−1.
SOD activity
Superoxide dismutase (SOD) activity was measured by using a SOD Assay Kit-WST (Dojindo Molecular Technologies, Inc., Kumamoto, Japan). For each SOD activity measurement, 20 µL of the sample solution was added to the wells for sample and blank 2. Twenty microliters of distilled water was added in the wells for blanks 1 and 3. Two hundred microliters of WST working solution was added to each well and mixed properly. Then, 20 µL of dilution buffer was added to the wells for blanks 2 and 3. After that, 20 µL of enzyme working solution was added to the wells for sample and blank 1 and then mixed thoroughly. The absorbance was measured at 450 nm using a microplate reader after incubation at 37ºC for 20 min. The SOD activity was calculated (inhibition rate %) using the following equation: SOD activity (inhibition rate %) = {[(A blank 1-A blank 3)-(A sample-A blank 2)]/(A blank 1-A blank 3)}×100.
POX activity
Peroxidase (POX) activity was analyzed by the following procedure of Hoque et al. [Citation21]. A reaction buffer solution was prepared which contained 50 mM KH2PO4 buffer (pH 7.0), 0.1 mM EDTA, 0.1 mM H2O2 and 10 mM guaiacol. The reaction was started by the addition of the sample solution to the reaction buffer solution. The activity was calculated from change in absorbance at 470 nm for 30 s where an extinction coefficient is 26.6 mM M−1 cm−1.
Determination of Se contents
Selenium contents were determined by the following procedure of Ohno et al. [Citation43] with modifications. Each sample was digested with 5 mL of HNO3 at 130–150ºC and then treated with 1 mL of concentrated HCl. The Se was extracted with distilled water, and the amounts of extracted Se were quantified by fluorometric assay using 2,3-diaminonapthalene (DAN). Concisely, 2 mL of masking reagent (EDTA, NH4OH, NH2OH.HCl, and methyl orange) was added to the cooled extraction. The volume mixture was adjusted to 9 mL, and then freshly prepared 0.1% DAN (in 0.1 N HCl) was added in the mixture and incubate it 30 min at 50ºC. After cooled the mixture, 5 mL of cyclohexane was added to the mixture and the fluorescence of the cyclohexane solution was measured at 520 nm (excitation, 375 nm) using a fluorescence spectrophotometer. Selenium standard solution (Wako, Japan) was used to measure the Se contents.
Measurement of protein content
Protein contents were determined as described previously [Citation44].
Statistical analysis
The significance of differences between the mean values of all parameters was assessed by Tukey’s test. Differences at the level of P ≤ 0.05 were considered as significant.
Results
Exogenous proline did not mitigate growth inhibition of BY-2 cells under selenate stress
In order to investigate effects of selenate and proline on cell growth of BY-2 cells, we tested 250 µM selenate (), which is toxic but not severely to rice plants [Citation8], and 10 mM proline, which improves salt tolerance and cadmium tolerance in BY-2 cells [Citation20–Citation22].
Figure 1. Effects of exogenous proline (Pro) on BY-2 cell growth under selenate stress. Effects of 5 mM and 10 mM proline on BY-2 cell growth at 0, 4, 6, and 8 days after inoculation (DAI) under 250 µM selenate stress based on fresh weight (a) and (b) based on dry weight. Averages of cell growth from three independent experiments (n = 3) are shown. Error bars represent SE. For the same inoculation day, bars with the same letters are not significantly different at P < 0.05.

The effects of selenate on BY-2 cell growth were examined by measuring the fresh weight (FW) and dry weight (DW) of selenate-stressed BY-2 cells at 0, 4, 6, and 8 days after inoculation (DAI). The FW of BY-2 cells cultured in the selenium medium was significantly lower than that in the control medium at 4, 6, and 8 DAI ()). The DW of BY-2 cultured cells in the selenium medium was significantly lower than that in the control medium at 4 and 6 DAI ()). These results indicate that selenate at 250 µM significantly inhibits the growth of BY-2 cells.
In order to investigate whether exogenous proline mitigates the inhibitory effects of selenate on the growth of BY-2 cells, the FW and DW of BY-2 cells at 0, 4, 6, and 8 DAI were measured. Application of proline at 5 mM and 10 mM did not significantly ameliorate the selenate-induced growth inhibition in the BY-2 cells (), suggest that the mechanism of growth inhibition by selenium is different from that by other metals such as Na and Cd.
Exogenously applied proline did not recover selenate-induced cell death
We used Evans Blue to evaluate the cell death of BY-2 induced by selenate in the presence and absence of proline. Under non-stress control condition, the stained cells accounted for 2.8% and 8.8% in the absence of proline at 2 days and 4 days, respectively, and 4.9% and 9.5% in the presence of 10 mM proline at 2 days and 4 days, respectively ()). When treated with selenate, the percentage of stained cells was increased by 10.7% and 28.6% in the absence of proline at 2 days and 4 days, respectively, and by 14.5% and 34.2% in the presence of proline at 2 days and 4 days, respectively ()). These results indicate that selenate drastically induces cell death of BY-2 but that application of proline does not affect the cell death.
Figure 2. Effects of exogenous application of proline on selenate-induced BY-2 cell death. (a) Selenate at 250 µM significantly induced cell death and supplementation of proline at 10 mM did not recover the selenate-induced cell death. Averages of cell death from three independent experiments (n = 3) are shown. The error bars represent SE. Values indicated by the same letter do not differ significantly at 5% level of significance as determined by Tukey’s test. (b) Representative images showing that Evans Blue staining of selenate-treated BY-2 cells in the presence or absence of proline. Scale bar = 20 µm.

Exogenously applied proline did not affect ROS accumulation in BY-2 cells under selenate stress
Intracellular ROS accumulation in the BY-2 cells was evaluated by using 2´,7´-dichlorodihydrofluorescein diacetate (H2DCF-DA). The application of 250 µM selenate significantly induced the accumulation of intracellular ROS in the BY-2 cells (). Exogenous proline at 10 mM did not affect the ROS accumulation induced by selenate stress ().
Figure 3. Effects of exogenous proline (Pro) on intracellular ROS accumulation and lipid peroxidation on BY-2 cells under selenate stress. (a) Intracellular ROS level in 4-day-old Se-unadapted tobacco BY-2 suspension cells under selenate stress in the presence or absence of proline. (b) Representative fluorescence images of 4-day-old Se-unadapted tobacco BY-2 suspension cells under selenate stress in the presence or absence of proline. Scale bar = 20 µm. (c) Malondialdehyde (MDA) content in 4-day-old Se-unadapted tobacco BY-2 suspension cells under selenate stress in the presence or absence of proline. The percentage of fluorescence intensity is shown as a ROS level. Averages from three independent experiments (n = 3) per bar are shown. Error bars represent SE. Bars with the same letters are not significantly different at P < 0.05.

Effects of supplementation of proline on lipid peroxidation of BY-2 cells under selenate stress
Effects of supplementation of proline on lipid peroxidation of selenate-stressed BY-2 cells were investigated using thiobarbituric acid. Selenate at 250 µM did not increase the MDA contents irrespective of the presence or absence of 10 mM proline in the culture medium ()), suggesting that selenate stress does not induce lipid peroxidation in the BY-2 cells.
Antioxidant enzyme activities of BY-2 cells under selenate stress
In order to investigate the mitigation effects of proline on the selenate-stressed plants, activities of antioxidant enzymes, APX, CAT, SOD, and POX in the BY-2 cells under selenate stress were examined. The activities of APX, CAT, SOD, and POX were significantly increased in the BY-2 cells in response to selenate stress (). Exogenous application of 10 mM proline significantly increased the APX and POX activities of the selenate-stressed cells () but did not significantly increase the CAT or SOD activity of the stressed cells (). These results indicate that selenate stress increases antioxidant enzyme activities and that exogenous proline does not increase all antioxidant enzyme activities of BY-2 cells under selenate stress.
Figure 4. Activities of antioxidant enzymes (a) APX, (b) CAT, (c) SOD, and (d) POX in 4-day-old Se-unadapted tobacco BY-2 suspension cells induced by proline and selenate. The activity of APX, CAT, and POX was expressed in units mg−1 protein and the SOD activity was expressed in % inhibition. One unit of activity was defined as the μmol substrate metabolized per min. Averages from three independent experiments (n = 3) per bar are shown. Error bars represent SE. Bars with the same letters are not significantly different at P < 0.05.

Exogenously applied proline enhanced the Se accumulation under selenate stress
To evaluate the effects of exogenous application of proline on Se accumulation, we determined Se contents in BY-2 cells under selenate stress in the presence or absence of proline. Selenate stress significantly increased Se contents in BY-2 cells () and exogenous application of 10 mM proline enhanced the Se accumulation in the selenate-stressed BY-2 cells ().
Figure 5. Effects of exogenous application of proline on Se accumulation. Selenium contents in 4-d-old Se-unadapted tobacco BY-2 suspension cells induced by proline under Se stress. Averages from three independent experiments (n = 3) per bar are shown. “ND” indicates the not detected. Error bars represent SE. Bars with the same letters are not significantly different at P < 0.05.

Effects of ROS scavengers in BY-2 cells under selenate stress
Ascorbic acid (AsA) and glutathione (GSH) are non-enzymatic antioxidants, which are capable of scavenging ROS. To clarify whether these ROS scavengers mitigate the growth inhibition by selenate stress, the effects of GSH and AsA were examined in the BY-2 cells under selenate stress. Glutathione at 0.5 mM significantly mitigated the selenate-induced growth inhibition whereas AsA at 20 mM did not ameliorate the growth inhibition by selenate stress (). Furthermore, the effects of AsA and GSH on intracellular ROS accumulation in the BY-2 cells under selenate stress were examined. Not only AsA at 20 mM but also GSH at 0.5 mM significantly decreased the intracellular ROS accumulation in the BY-2 cells under selenate stress, where neither AsA nor GSH changes the ROS accumulation in the non-stressed BY-2 cells (). These results suggest that the growth inhibition by selenate stress is not accounted for by ROS accumulation.
Figure 6. Effects of ascorbic acid (AsA) and glutathione (GSH) on 4-day-old BY-2 cell growth under selenate stress. (a) Shows the cell growth on the basis of fresh weight and (b) shows the cell growth on the basis dry weight in response to 20 mM AsA, 0.5 mM GSH in presence or absence of selenate stress. Averages of cell growth from three independent experiments (n = 3) are shown. Error bar represents SE. Bars with the same letters are not significantly different at P < 0.05.

Figure 7. Effects of ascorbic acid (AsA) and glutathione (GSH) on intracellular ROS accumulation in BY-2 cells under selenate stress. (a) Intracellular ROS levels in 4-day-old Se-unadapted tobacco BY-2 suspension cells under selenate stress in the presence or absence of AsA and GSH. (b) Representative fluorescence images of 4-day-old Se-unadapted tobacco BY-2 suspension cells under selenate stress in the presence or absence of AsA and GSH. Scale bar = 20 µm. The percentage of fluorescence intensity is shown as a ROS level. Averages from three independent experiments (n = 3) per bar are shown. Bars with the same letters are not significantly different at P < 0.05.

Discussion
Many studies reported that proline plays a defensive role against various stresses in plants and cultured cells. Exogenous proline is known to alleviate Na-and Cd-induced growth inhibition in BY-2 cells [Citation21,Citation22,Citation41] and exogenous proline at lower concentrations suppressed growth inhibition of BY-2 cells by arsenate stress [Citation26]. However, the defensive role of proline against selenate stress in BY-2 cells remains to be understood. Selenate toxicity was also evident from the inhibition of growth in selenate-stressed BY-2 cells () but proline did not mitigate the growth inhibition by selenate (). However, Aggarwal et al. [Citation10] reported that exogenous proline alleviates phytotoxic effects of selenium by reducing oxidative stress and improves growth in Bean (Phaseolus vulgaris L.) seedlings. This difference may come from the difference in endogenous proline contents between BY-2 cells (approximately 3 mM) [Citation26] and bean seedlings (approximately 2 µM) [Citation45]. In addition, selenate stress may affect proline metabolism. Proline content has been known to be involved in salt, drought, and heavy-metal tolerance mechanisms in plants [Citation46–48]. Under stress conditions, the level of proline is maintained by biosynthetic enzyme pyrroline-5-carboxylate synthetase (P5CS) as well as degrading enzyme proline dehydrogenase (ProDH). Proline is catabolized to cytotoxic glutamate-5-semialdehyde (GSA)/pyrroline-5-carboxylate (P5C) by proline dehydrogenase and then GSA/P5C is converted to glutamate by pyrroline-5-carboxylate dehydrogenase (P5CDH) [Citation15,Citation49,Citation50]. Therefore, the reduction of cytotoxic GSA is critical to the stress mitigation by exogenous proline, that is, inhibition of P5CDH activity by the stress can impair the mitigation by proline. The present results in this study suggest that selenate inhibits P5CDH, resulting in accumulation of cytotoxic GSA. However, it is difficult to quantitate GSA and P5C because they are an equilibrated mixture.
Both salt stress and arsenate stress increased the number of Evans Blue-stained BY-2 cells [Citation26,Citation29]. However, the salt-induced cell death was suppressed by supplementation of proline [Citation29] but the arsenate-induced cell death was aggravated by proline [Citation26]. Proline induced cell death by ProDH activation, which leads to GSA accumulation [Citation15]. Hence, the difference in response to proline between salt-induced cell death and arsenate-induced cell death may be due to proline metabolism such as GSA accumulation. In this study, selenate stress induced cell death of BY-2 but the cell death was not affected by exogenous proline (), which may be accounted for the proline metabolism.
Several studies have reported that selenate stress can increase intracellular ROS accumulation in plants [Citation51–53]. Similarly, this study showed that intracellular ROS accumulation was increased by selenate stress ()). In plants, low concentration of Se reduces the toxic effects of heavy metals by alleviating oxidative damages while high dose of Se induces the oxidative stress [Citation54–56]. In this study, selenate stress increased antioxidant activities () and drastically induced selenate uptake (), resulting in growth inhibition and cell death.
Islam et al. [Citation22] reported that Cd stress increased lipid peroxidation and supplementation of proline decreased Cd-induced lipid peroxidation in BY-2 cells. Several studies have reported that salt-induced lipid peroxidation is decreased by the exogenous application of proline [Citation29,Citation41,Citation57]. The present study showed that selenate stress did not affect the MDA content and that exogenous proline also did not show any effect on MDA content under selenate stress ()). These results indicate that the level of oxidative damage was not high enough to induce lipid peroxidation in selenate-treated BY-2 cells and that selenate inhibited BY-2 cell growth not via increasing oxidative damage.
It is well known that proline plays an important role in protecting different enzymes against stresses such as salinity stress [Citation20,Citation23–Citation25]. Some previous studies reported that the antioxidant defense system under salt and Cd stress was increased by exogenous proline application in BY-2 cells [Citation21,Citation29,Citation41]. Hossain et al. [Citation58] reported that the ascorbate peroxidase (APX) activity was increased in response to Cd stress on mung bean and supplementation of proline also increased APX activity under Cd stress. It has been reported that the APX, CAT, and POX activities are increased in response to salt stress and supplementation of proline also increases APX, CAT, and POX activities under salt stress on rice cultivars and suggest that proline detoxifies H2O2 by enhancing APX, CAT, and POX activities under salt stress [Citation59,Citation60]. In this study, selenate stress increased activities of APX, CAT, SOD, and POX (). Exogenous application of proline increased the APX and POX activities () but did not affect either ROS accumulation ()) or the lipid peroxidation ()) in the BY-2 cells under selenate stress. In this study, proline enhanced APX and POX activities ( (a,d)) and also accelerated selenate accumulation (). Taken together with the result that proline did not mitigate selenate stress ( and ), these results suggest that the increment of APX and POX activities by exogenous proline is not sufficient to suppress selenate stress due to selenate accumulation.
Both AsA and GSH are known as a vital component for the detoxification of ROS in plants [Citation61–63]. Exogenous application of AsA improves phytotoxic effects of selenium on rice by reducing oxidative damage [Citation64]. Jung et al. [Citation65] reported that exogenous application of GSH alleviates heavy metal-induced oxidative damage and enhances heavy metal stress resistance in rice plants. In this study, the exogenous application of AsA and GSH significantly decreased the ROS accumulation in the selenate-stressed cells (). However, GSH application significantly mitigated the growth inhibition by selenate () but AsA application did not significantly alleviate the growth inhibition (). It has been reported that interference of GSH synthesis in plants by selenate or other Se compounds may diminish plant defense against hydroxyl radicals and oxidative stress [Citation9]. Hence, in this study, the exogenous application of GSH might increase the GSH level in BY-2 cells and the increased GSH might suppress the selenate toxicity. These results suggest that the application of proline does not always mitigate growth inhibition by heavy metals and suggest that the growth inhibition by selenate is not accounted for by ROS accumulation.
Conclusion
The presented results indicate that selenate induces cell death and inhibits growth in BY-2 cells while selenate elevates antioxidant enzyme activities and that proline enhances antioxidant enzymes such as APX and POX and also selenate accumulation. It is concluded that proline improves antioxidant activities and enhances selenate uptake and that the balance may account for growth inhibition and cell death.
Author’s contribution
D. M., S. M., and Y. M. planned the experiments; M. K. performed the experiments; D. M., M. S. R., and E. O., assisted in experiments; T. N., Y. N., and S. M. contributed to discussions; M. K., S. M., and Y. M. wrote the manuscript.
Disclosure statement
No potential conflict of interest was reported by the authors.
References
- Gupta M, Gupta S. An overview of selenium uptake, metabolism, and toxicity in plants. Front Plant Sci. 2017;11:2074.
- Hamilton SJ. Review of selenium toxicity in the aquatic food chain. Sci Total Environ. 2004;326:1–31.
- Guerrero B, Llugany M, Palacios O, et al. Dual effects of different selenium species on wheat. Plant Physiol Bioch. 2014;83:300–307.
- Rayman MP. Selenium and human health. Lancet. 2012;379:1256–1268.
- Prabhu KS, Lei XG. Selenium. Adv Nutr. 2016;7:415–417.
- Alyemeni MN, Ahanger MA, Wijaya L, et al. Selenium mitigates cadmium-induced oxidative stress in tomato (Solanum lycopersicum L.) plants by modulating chlorophyll fluorescence, osmolyte accumulation, and antioxidant system. Protoplasma. 2018;255:459–469.
- Hasanuzzaman M, Fujita M. Selenium pretreatment upregulates the antioxidant defense and methylglyoxal detoxification system and confers enhanced tolerance to drought stress in rapeseed seedlings. Biol Trace Elem Res. 2011;143:1758–1776.
- Mostofa MG, Hossain MA, Siddiqui MN, et al. Phenotypical, physiological and biochemical analyses provide insight into selenium-induced phytotoxicity in rice plants. Chemosphere. 2017;178:212–223.
- Terry N, Zayed AM, De Souza MP, et al. Selenium in higher plants. Annu Rev Plant Biol. 2000;51:401–432.
- Aggarwal M, Sharma S, Kaur N, et al. Exogenous proline application reduces phytotoxic effects of selenium by minimising oxidative stress and improves growth in bean (Phaseolus vulgaris L.) seedlings. Biol Trace Elem Res. 2011;140:354–367.
- White PJ. Selenium accumulation by plants. Ann Bot. 2016;117:217–235.
- Bohnert HJ, Nelson DE, Jensen RG. Adaptations to environmental stresses. Plant Cell. 1995;7:1099–1111.
- Sleator RD, Hill C. Bacterial osmo adaptation: the role of osmolytes in bacterial stress and virulence. FEMS Microbiol Revi. 2002;26:49–71.
- Verslues PE, Bray EA. Role of abscisic acid (ABA) and Arabidopsis thaliana ABA-insensitive loci in low water potential-induced ABA and proline accumulation. J Exp Bot. 2006;57:201–212.
- Amini S, Ghobadi C, Yamchi A. Proline accumulation and osmotic stress: an overview of P5CS gene in plants. J Plant Mol Breed. 2015;3:44–55.
- Fabro G, Kovacs I, Pavet V, et al. Proline accumulation and AtP5CS2 gene activation are induced by plant-pathogen incompatible interactions in Arabidopsis. Mol Plant Microbe Interact. 2004;17:343–350.
- Sharma SS, Dietz KJ. The relationship between metal toxicity and cellular redox imbalance. Trends Plant Sci. 2009;14:43–50.
- Hayat S, Hayat Q, Alyemeni MN, et al. Role of proline under changing environments: a review. Plant Signal Behav. 2012;7:1456–1466.
- Harinasut P, Tsutsui K, Takabe T, et al. Glycinebetaine accumulation and increased salt-tolerance in rice seedlings. Biosci Biotechnol Biochem. 1996;60:366–368.
- Okuma E, Soeda K, Tada M, et al. Exogenous proline mitigates the inhibition of growth of Nicotiana tabacum cultured cells under saline conditions. Soil Sci Plant Nutr. 2000;46:257–263.
- Hoque MA, Banu MNA, Okuma E, et al. Exogenous proline and glycinebetaine increase NaCl-induced ascorbate–glutathione cycle enzyme activities, and proline improves salt tolerance more than glycinebetaine in tobacco Bright Yellow-2 suspension-cultured cells. J Plant Physiol. 2007;164:1457–1468.
- Islam MM, Hoque MA, Okuma E, et al. Exogenous proline and glycinebetaine increase antioxidant enzyme activities and confer tolerance to cadmium stress in cultured tobacco cells. J Plant Physiol. 2009;166:1587–1597.
- Tsugane K, Kobayashi K, Niwa Y, et al. A recessive Arabidopsis mutant that grows photo autotrophically under salt stress shows enhanced active oxygen detoxification. Plant Cell. 1999;11:1195–1206.
- Hong Z, Lakkineni K, Zhang Z, et al. Removal of feedback inhibition of Δʹ-pyrroline-5-carboxylate synthetase results in increased proline accumulation and protection of plants from osmotic stress. Plant Physiol. 2000;122:1129–1136.
- Okuma E, Soeda K, Fukuda M, et al. Negative correlation between the ratio of K+ to Na+ and proline accumulation in tobacco suspension cells. Soil Sci Plant Nutr. 2002;48:753–757.
- Nahar MNN, Islam MM, Hoque MA, et al. Exogenous proline enhances the sensitivity of tobacco BY-2 cells to arsenate. Biosci Biotechnol Biochem. 2017;81:1726–1731.
- Noctor G, Foyer CH. Ascorbate and glutathione: keeping active oxygen under control. Annu Rev Plant Biol. 1998;49:249–279.
- Apel K, Hirt H. Reactive oxygen species: metabolism, oxidative stress, and signal transduction. Annu Rev Plant Biol. 2004;55:373–399.
- Banu MNA, Hoque MA, Watanabe-sugimoto M, et al. Proline and glycinebetaine induce antioxidant defense gene expression and suppress cell death in cultured tobacco cells under salt stress. J Plant Physiol. 2009;166:146–156.
- Guo H, Kouzuma Y, Yonekura M. Structures and properties of antioxidative peptides derived from royal jelly protein. Food Chem. 2009;113:238–245.
- Sharma P, Jha AB, Dubey RS, et al. Reactive oxygen species, oxidative damage, and antioxidative defense mechanism in plants under stressful conditions. J Bot. 2012. DOI:10.1155/2012/217037
- Foyer CH, Noctor G. Redox signaling in plants. Antioxid Redox Sign. 2013;18:2087–2090.
- Turkan I. ROS and RNS: key signalling molecules in plants. J Exp Bot. 2018;69:3313–3315.
- Asada K. The water-water cycle in chloroplasts: scavenging of active oxygens and dissipation of excess photons. Annu Rev Plant Biol. 1999;50:601–639.
- Polle A. Dissecting the superoxide dismutase-ascorbate-glutathione-pathway in chloroplasts by metabolic modeling. Computer simulations as a step towards flux analysis. Plant Physiol. 2001;126:445–462.
- Hellmann H, Funck D, Rentsch D, et al. Hypersensitivity of an Arabidopsis sugar signaling mutant toward exogenous proline application. Plant Physiol. 2000;123:779–789.
- Murata Y, Obi I, Yoshihashi M, et al. Reduced permeability to K+ and Na+ ions of K+ channels in the plasma membrane of tobacco cells in suspension after adaptation to 50 mM NaCl. Plant Cell Physiol. 1994a;35:87–92.
- Murata Y, Obi I, Yoshihashi M, et al. Salt adaptation of K+ channels in the plasma membrane of tobacco cells in suspension culture. Plant Cell Physiol. 1994b;35:637–644.
- Linsmaier EM, Skoog F. Organic growth factor requirements of tobacco tissue cultures. Physiol Plant. 1965;18:100–127.
- Biswas MS, Mano J. Lipid peroxide-derived short-chain carbonyls mediate hydrogen peroxide-induced and salt-induced programmed cell death in plants. Plant Physiol. 2015;168:885–898.
- Okuma E, Murakami Y, Shimoishi Y, et al. Effects of exogenous application of proline and betaine on the growth of tobacco cultured cells under saline conditions. Soil Sci Plant Nutr. 2004;50:1301–1305.
- Ishikawa T, Morimoto Y, Madhusudhan R, et al. Acclimation to diverse environmental stresses caused by a suppression of cytosolic ascorbate peroxidase in tobacco BY-2 cells. Plant Cell Physiol. 2005;468:1264–1271.
- Ohno M, Uraji M, Shimoishi Y, et al. Mechanisms of the selenium tolerance of the Arabidopsis thaliana knockout mutant of sulfate transporter SULTR1; 2. Biosci Biotechnol Biochem. 2012;76:993–998.
- Bradford MM. A rapid and sensitive method for the quantitation of microgram quantities of protein utilizing the principle of protein-dye binding. Anal Biochem. 1976;72:248–254.
- Zengin F. Exogenous treatment with salicylic acid alleviating copper toxicity in bean seedlings. Proc Natl Acad Sci India Sect B Biol Sci. 2014;84:749–755.
- Iqbal N, Umar S, Khan NA. Nitrogen availability regulates proline and ethylene production and alleviates salinity stress in mustard (Brassica juncea). J Plant Physiol. 2015;178:84–91.
- Choudhary NL, Sairam RK, Tyagi A. Expression of delta1-pyrroline-5-carboxylate synthetase gene during drought in rice (Oryza sativa L.). Indian J Biochem Bio. 2005;42:366–370.
- Chen CT, Chen L, Lin CC, et al. Regulation of proline accumulation in detached rice leaves exposed to excess copper. Plant Sci. 2001;160:283–290.
- Deuschle K, Funck D, Hellmann H, et al. A nuclear gene encoding mitochondrial Δ1-pyrroline-5-carboxylate dehydrogenase and its potential role in protection from proline toxicity. Plant J. 2001;27:345–356.
- Kim GB, Nam YW. A novel Δ1-pyrroline-5-carboxylate synthetase gene of Medicago truncatula plays a predominant role in stress-induced proline accumulation during symbiotic nitrogen fixation. J Plant Physiol. 2013;170:291–302.
- Tamaoki M, Freeman JL, Pilon-Smits EA. Cooperative ethylene and jasmonic acid signaling regulates selenite resistance in Arabidopsis. Plant Physiol. 2008;146:1219–1230.
- Akbulut M, Cakır S. The effects of Se phytotoxicity on the antioxidant systems of leaf tissues in barley (Hordeum vulgare L.) seedlings. Plant Physiol Bioch. 2010;48:160–166.
- Schiavon M, Moro I, Pilon-Smits EA, et al. Accumulation of selenium in Ulva sp. and effects on morphology, ultrastructure and antioxidant enzymes and metabolites. Aquat Toxicol. 2012;122:222–231.
- Malik JA, Goel S, Kaur N, et al. Selenium antagonises the toxic effects of arsenic on mungbean (Phaseolus aureus Roxb.) plants by restricting its uptake and enhancing the antioxidative and detoxification mechanisms. Environ Exp Bot. 2012;77:242–248.
- Jozwiak W, Politycka B. Effect of selenium on alleviating oxidative stress caused by a water deficit in cucumber roots. Plants. 2019;8:217.
- Chen Y, Mo HZ, Hu LB, et al. The endogenous nitric oxide mediates selenium-induced phytotoxicity by promoting ROS generation in Brassica rapa. PloS One. 2014;9:e110901.
- Demiral T, Turkan I. Does exogenous glycinebetaine affect antioxidative system of rice seedlings under NaCl treatment? J Plant Physiol. 2004;161:1089–1100.
- Hossain MA, Hasanuzzaman M, Fujita M. Up-regulation of antioxidant and glyoxalase systems by exogenous glycinebetaine and proline in mung bean confer tolerance to cadmium stress. Physiol Mol Biol Pla. 2010;1:259–272.
- Hasanuzzaman M, Alam M, Rahman A, et al. Exogenous proline and glycine betaine mediated upregulation of antioxidant defense and glyoxalase systems provides better protection against salt-induced oxidative stress in two rice (Oryza sativa L.) varieties. Biomed Res Int. 2014. DOI:10.1155/2014/757219
- Bhusan D, Das DK, Hossain M, et al. Improvement of salt tolerance in rice (Oryza sativa L.) by increasing antioxidant defense systems using exogenous application of proline. Aust J Crop Sci. 2016;10:50.
- Smirnoff N. Ascorbic acid: metabolism and functions of a multifaceted molecule. Curr Opin Plant Biol. 2000;3:229–235.
- Foyer CH, Noctor G. Ascorbate and glutathione: the heart of the redox hub. Plant Physiol. 2011;155(1):2–18.
- Qian HF, Peng XF, Han X, et al. The stress factor, exogenous ascorbic acid, affects plant growth and the antioxidant system in Arabidopsis thaliana. Russ J Plant Physiol. 2014;61:467–475.
- Sharma S, Kaur N, Kaur S, et al. Ascorbic acid reduces the phytotoxic effects of selenium on rice (Oryza Sativa L.) by up-regulation of antioxidative and metal-tolerance mechanisms. J Plant Physiol Pathol. 2014;2:3.
- Jung HI, Kong MS, Lee BR, et al. Exogenous glutathione increases arsenic translocation into shoots and alleviates arsenic-induced oxidative stress by sustaining ascorbate–glutathione homeostasis in rice seedlings. Front Plant Sci. 2019;10. DOI:10.3389/fpls.2019.01089