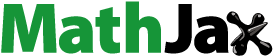
ABSTRACT
Hydroxyl radical (•OH) is considered to be the most damaging among reactive oxygen species. Although a few studies have reported on its effects on growth and stress adaptation of plants, no detailed studies have been performed using •OH in germination and early seedling growth under abiotic stresses. Here we report a single seed treatment with •OH on germination and seedling growth of Arabidopsis and rice under non-stressed (ambient) and various abiotic-stressed conditions (chilling, high temperature, heat, and salinity). The treatment resulted in faster seed germination and early seedling growth under non-stressed conditions, and, interestingly, these effects were more prominent under abiotic stresses. In addition, Arabidopsis seedlings from treated seeds showed faster root growth and developed more lateral roots. These results show a positive and potential practical use for •OH in model and crop plants for direct seeding in the field, as well as improvement of tolerance against emerging stresses.
Abbreviations: AUC: area under curve; MGT: mean germination time; t50: time to reach 50% germination; U7525: time for uniform germination from 25% to 75%; ROS: reactive oxygen species; GSI: germination speed index; SI: stress index; DI: dormancy index
Graphical abstract

A single treatment of Arabidopsis and rice seeds with hydroxy radical (•OH) improves germination and seedling growth under both ambient and stress conditions.
Plants are sessile organisms and are continuously exposed to various biotic and abiotic stresses throughout their lifetimes. Moreover, alterations of the rhythm of seasonal changes by global climate change aggravate the severity of these stresses on plants. Simultaneous exposure to different abiotic and biotic stresses have much more devastating effects on plant growth and development and resulting in yields lower than the plants exposed to an individual stress in the field [Citation1–4]. Different abiotic stresses such as cold/chilling [Citation5–7], salinity [Citation8–11], osmotic [Citation12,Citation13], drought and/or water deprivation, and high temperature [Citation14,Citation15] negatively affect the seed germination, seedling establishment, survival of the young seedlings [Citation16–18]. Multiple abiotic stresses have a significant impact on crop production and food security all over the world [Citation2–4,Citation19,Citation20]. Additionally, climate variation accounts for over 32%–39% of yield variability, and extreme climate events, which are also affecting arable land availability, are exacerbating the loss of global crop yield [Citation21,Citation22]. Numerous approaches have been developed over the last few decades to improve plant performance against multiple abiotic stresses in different climatic conditions around the world [Citation3,Citation23]
In the last couple of decades, several strategies have been developed to fight against biotic and abiotic stresses, including strategies using conventional breeding, genetic engineering, and selection of resistant varieties, some of which are tightly associated with mitigating the impact of stresses arising from global climate changes. However, some of these methods are time-consuming, expensive, and controversial. For example, genetically modified organisms have the potential for increasing tolerance against stresses, but their use is unpopular with growers and consumers. Additionally, the gaps in knowledge on the application between the labs and field, and from model plant to crop plant transitions require extensive studies [Citation24–26].
Alongside the methods mentioned above, a growing number of alternative approaches are emerging to deal with multiple abiotic stresses. For example, priming treatments, in which plants are pre-treated under stressed conditions or with endogenous, natural, or synthetic chemicals to prepare them against upcoming stresses, have gained popularity [Citation25,Citation27,Citation28]. Among the different kinds of priming treatments, the most promising and commonly used chemicals are polyamines, hormones such as salicylic acid, reactive sulfur species such as NaHS, reactive nitrogen species such as nitric oxide, and reactive oxygen species (ROS) such as hydrogen peroxide (H2O2) [Citation29–32]. Exogenous application of these chemicals has been found to be effective against different abiotic stresses [Citation32,Citation33]. However, most of these treatments are focused on a single abiotic stress at a single stage of growth and development.
ROS, including singlet oxygen (1O2), superoxide (O2−), hydrogen peroxide (H2O2), and the hydroxyl radical (•OH), are involved in almost every stage of growth, development, and differentiation [Citation34–38]. Researchers have reported that exogenous application of H2O2 enhances stress tolerance in both model and crop plants by increasing the endogenous concentration to an optimum level [Citation25,Citation39]. Before the discovery of the positive roles of H2O2, ROS were considered toxic byproducts of oxygen metabolism. In particular, •OH is still considered a dangerous compound in the cell that damages DNA and proteins and is responsible for cell death [Citation39,Citation40]. However, similar to H2O2, several positive roles of •OH in cell growth, elongation, germination, and stress signaling processes have been reported [Citation40]. •OH has been found to accelerate the elongation and growth of coleoptiles and roots in maize seedlings [Citation41–43]. It was also found to contribute to the elongation of radicles and the acceleration of germination in cress seeds [Citation44]. However, no papers have reported the application of •OH to improve seed germination and early seedling growth under stressful environments in crop plants.
Recently, we filed a patent #CA2949750 [Citation45] on the catalytic seed treatment phenomenon, which may be operating through the Fenton/Haber Weiss reaction and generating •OH to enhance seed germination and lateral root growth. Because •OH is highly reactive and short-lived, it cannot be added directly in the same manner as H2O2. Therefore, in this study, the Fenton/Haber-Weiss reaction was used for •OH treatment. Furthermore, many physiological and biochemical studies have confirmed that this reaction is equivalent to •OH treatment [Citation46–50]. In this study, Arabidopsis was used as a model system to investigate the mechanism of action, and rice was also studied to validate the breadth of this phenomenon in other crops. The single seed treatment with •OH (with H2O2 as a substrate and Fe or Cu as a catalyst) had multiple positive effects: accelerated germination and early hypocotyl growth in the short term, increased lateral root numbers, and improved tolerance during the germination process to several abiotic stresses in both Arabidopsis and rice. The overall findings from this work will help to improve plant performance against a wide range of abiotic stresses through a simple, single application of •OH based on the Fenton/Haber Weiss reaction in a cost-, time- and labor-effective manner.
Materials and methods
Plant materials
Seeds of Arabidopsis thaliana ecotype Columbia-0 (Col-0) and Oryza sativa cv. Nipponbare were used for experiments. Arabidopsis seeds were purchased from Lehle Seeds (Round Rock, USA) and multiplied in our lab. Rice seeds were purchased from Noken Co. (Kyoto, Japan). Seeds were stored at 4°C in a dry container until use.
Seed treatment with reactive oxygen species
A combination of H2O2 and a metal catalyst, FeSO4.7H2O, or CuSO4.5H2O, was used to generate •OH based on the Fenton reaction/Haber Weiss (see formula below). The metal catalysts were used in a ratio of 1:200 with H2O2. •OH catalyzed by FeSO4.7H2O and CuSO4.5H2O is denoted •OH(Fe) and •OH(Cu), respectively. Seeds were also treated with the same concentration of H2O2 without any metal catalyst to compare against the effect of •OH. All solutions were prepared at pH 4.9 using 0.1 M sodium citrate buffer.
H2O2 + Fe (II) → Fe (III) + •OH + OH−
H2O2 + Cu (I) → Cu (II) + •OH + OH−
After surface sterilization, Arabidopsis seeds were treated with •OH(Fe/Cu) (0, 3, 6, and 25 mM H2O2 in the ratio with Fe/Cu mentioned above) or a similar concentration of H2O2 without any metal catalyst in 1.5 mL microtubes. The tubes were incubated for 1, 4, 8, and 24 h in the dark at room temperature (23°C) before seeding. Rice seeds were submerged in •OH(Fe) (0, 25, 50, 75, and 100 mM H2O2 in the ratio with Fe mentioned above) in 50 mL Falcon tubes and incubated at room temperature on a rotary shaker at 80 rpm for 6 h in the dark. The seeds were then planted in a designated medium without washing off the treatment solution except for one batch of •OH(Fe)-treated Arabidopsis seeds, in which the seeds were washed with ultrapure water after incubation (indicated as •OH(Fe)+W). All seeds were germinated under the ambient condition.
Germination and growth assay in ambient conditions
Arabidopsis seeds were germinated on 0.8% agar (Wako, Japan) medium with a modified Hoagland solution [Citation51]. For each treatment condition, at least 80–100 seeds were sown on the agar medium without clustering in a 9 cm square petri dish. The agar plates were placed vertically inside a growth chamber (CLE-303, Tomy Digital Biology, Tokyo, Japan) at 23°C under dark conditions. For rice, at least 30 seeds were germinated with 5 mL of water in a 9 cm petri dish and kept at 30°C under dark inside a growth chamber (E-8 growth chamber, Conviron, Winnipeg, Manitoba, Canada). All experiments were repeated more than three times in duplicate. A seed was considered germinated when the radicle emerged by protruding the seed coat. Germination data were collected by taking photographs at different time points to determine the time required for 50% germination (t50). The t50 was considered as the speed of germination, which has been used as a determining factor for seed vigor in different studies [Citation52,Citation53]. Final germination numbers for both Arabidopsis and rice plants were counted 48 h after transfer to the growth chamber.
The root growth of Arabidopsis at 23°C was marked every 24 h from day 5 to day 9 after the seeds were placed on agar media. The lateral roots were counted at day 10. All roots, including taproots and lateral roots, were measured for more than 30 plants from at least five replicates. All photographs were taken using high-resolution cameras (Canon 80D, 100D, and M5) and analyzed using the open-source image analysis software ImageJ (version 1.51, https://imagej.nih.gov/ij/). For rice, the radicle length of 48-h-old seedlings was measured.
Seed treatment on germination under different abiotic stresses
For chilling stress, Arabidopsis seeds treated with or without •OH(Fe) solution were planted on agar as described above and placed vertically inside a growth chamber (CLE-303, Tomy Digital Biology, Tokyo, Japan) at 11°C in the dark. Photographs were taken every 12 h after transfer to the chamber.
To study the germination performance under high temperature, Arabidopsis seeds with or without •OH(Fe) treatment were planted on agar plates and kept vertically in a growth chamber at 30°C. Data were taken at several different time points after transfer to the chamber.
To expose seeds to heat stress, a slightly modified version of the basal heat stress method used by Silva-Correia (2014) [Citation54] was applied: treated and untreated Arabidopsis seeds were washed and kept at 50°C in a heat block (ALB-120, Iwaki Asah Techno Glass, Japan) for 65 min and then transferred to 23°C conditions. Photographs were taken every 12 h. Maximum germination was measured at 96 h after transfer to the chamber.
Treated and untreated Arabidopsis seeds were germinated on agar in the presence of 120 mM and 150 mM NaCl and kept vertically in a growth chamber. Photographs were taken every 12 h after transfer to the growth chamber. For rice seeds, treated or untreated seeds were germinated in water containing different concentrations of NaCl ranging from 25 to 200 mM.
Statistical analysis of data using the four parameter hill function (4PHF)
Statistical analysis was carried out using the “Germinator_curve-fitting 1.31.xls” Microsoft Excel solver, a part of the “Germinator” software package developed by Joosen et al. [Citation55]. This solver was developed based on the four parameter Hill function (4PHF), a mathematical model described by El-Kassaby et al. [Citation56] to describe germination properties from curve fitting. The solver can extract valuable information from a germination curve that is biologically relevant to the germination characteristics of a seed population using the following equation:
where y is the cumulative germination percentage at time x (h), y0 is the intercept on the y-axis (≥0), a is the maximum cumulative germination percentage (≤100), b controls the shape and steepness of the germination curve, and c is the time to reach 50% germination by viable seeds (t50). A detailed description of how this solver performs the statistical analysis can be found in the article by Joosen et al. [Citation55] and also on the website (https://www.wur.nl/en/Research-Results/Chair-groups/Plant-Sciences/Laboratory-of-Plant-Physiology/Wageningen-Seed-Lab/Resources/Germinator.htm).
From this 4PHF, germination performance was measured by extracting information such as maximum germination (gMAX), mean germination time (MGT), time for 50% viable seed germination (t50), germination uniformity (Ub-a), and area under the germination curve (AUC). The germination uniformity (U) can be set to different ranges of germination; for example, U75-25 is the uniformity from 25% to 75% germination of the seeds. AUC is the area between the germination curve and the x-axis (the germination time).
El-Kassaby et al. [Citation56] defined the differences between areas under the curve of cold-stratified and unstratified seeds (ΔAUC = AUCstratified seeds − AUCunstratified seeds) as the dormancy index (DI), which can be used as a quantitative measure of changes in seed vigor due to specific treatments. Using a similar idea to DI, we used ΔAUC to quantify the effect of the ROS treatment under ambient and stress conditions. In this study, ΔAUC under ambient conditions (23°C for Arabidopsis and 30°C for rice) was defined as the germination speed index (GSI) and under stress conditions as the stress index (SI) as defined by Joosen et al. [Citation55]. GSI and SI were measured by the averaged differences between the AUC of the ROS-treated seed germination curve (AUCROS) and AUC of the control seed germination curve (AUCControl) under different stress conditions (ΔAUC = ΔAUCROS − ΔAUCControl). In this experiment, AUC was counted from the initial germination time t = 0 to the maximum germination time for all experiments. This germination curve fitting summarized the data with different biologically relevant information regarding seed germination using Student’s t-test and Tukey’s HSD.
Results
Hydroxyl radical/ROS treatment accelerates germination and early seedling growth
In preliminary experiments with different “concentration × incubation period” combinations, the optimum treatment conditions for Arabidopsis were found to be 8 h of incubation in 0.03 mM Fe/Cu and 6 mM H2O2 solution. Under these conditions, it took the shortest time to reach 50% germination in most cases (Figure S1). To nullify the effect of the seed surface-bound treatment solution on germination and other developmental changes, •OH(Fe)-treated seeds were washed after 8 h incubation. The germination of the washed seeds maintained the similar tendency to the •OH(Fe)-treated seeds. Furthermore, to determine the effect of light on germination, seeds were germinated both under a 16-h light and 8-h dark cycle and under continuous dark. Arabidopsis seeds germinated faster under continuous dark than under the light-dark-cycling conditions. In the dark, seeds treated with the optimized treatment solution reached 50% germination 10 + h earlier than seeds germinated under the light and dark conditions (Figure S2). Thus, for all subsequent studies, the seeds were germinated in the dark.
The maximum germination of Arabidopsis seeds at ambient temperature reached over 95% in all treatments and control groups, and the treatment increased the speed of germination significantly ()). To extract more biologically relevant information from the germination data, the 4PHF was applied by inputting the germination data at different time points into the “Germinator curve-fitting 1.31.xls” solver [Citation55]. The solver returned similar results to the manual germination data analysis with a characteristic germination curve (Figure S3). This curve provided significant biological information such as t50, AUC, gMAX, MGT, and U7525 ( (b c) and S4).
Figure 1. Effect of the treatment on germination and early seedling growth of Arabidopsis at the ambient temperature (23ºC). (a) Germination rate at different time points, (b) time for 50% germination (t50) for all the seed groups, (c) area under curve (AUCs) for all the seed groups at 48th hour, (d) germination speed index (GSI) and (e) correlation of germination speed index (GSI) generated by subtracting AUC for the control seeds from AUCs for the •OH(Fe/Cu), •OH(Fe)+W, and H2O2-treated seeds and the germination percentage at different time points. The asterisk indicates a significant P < 0.05) difference between conditions obtained by the Student’s t-test. Letters (a–b) represent statistically different subsets (Tukey HSD, P < 0.05).

Because mean germination time does not provide clear information on the imbibition initiation time and specific germination percentage, the time to reach 50% germination (t50) was used to describe seed vigor [Citation57]. t50 is also a sensitive measure of seed quality and viability under field conditions [Citation53]. Germination speed was significantly (P < 0.05) faster in the •OH(Fe)-treated group than the control. The t50 for the •OH(Fe)-treated seeds was 5 h shorter than the control ()). There was a slight difference in t50 between the •OH(Fe/Cu)- and H2O2-treated seeds, which was not statistically significant ()). The germination speed was further quantified from the differences in the AUCs of the control and all the other ROS treatments (•OH(Fe/Cu), •OH(Fe), and then washing, and H2O2) ( (c d)). This difference was expressed as ΔAUC, where ΔAUC = AUC of any treatment group – AUC of the control group. The ΔAUC of a treatment under the optimum conditions was named the germination speed index, GSI. The GSI of the •OH(Fe)-treated group was correlated with the actual germination percentage of the •OH(Fe)-treated seeds. The GSI of the •OH(Fe) or •OH(Cu)-treated seeds at different time points was higher than that of the H2O2-treated seeds, and the extent of the effect was similar in the Fe and Cu systems ()). Interestingly, in all these data, we observed that the washed seeds, •OH(Fe)+W, showed a similar effect as the •OH(Fe)-treated seeds. When we considered the data from the •OH(Fe)-treated seeds in the statistical analysis, we found a highly significant correlation (r2 = 0.979, )). Based on data, showing that both metal catalysts (Fe, Fe+H2O2+W, and Cu) had similar effects on germination, only Fe-catalyzed data were used for further statistical analysis to determine the •OH treatment effects to avoid redundancy.
Early seedling growth was also positively affected by the •OH(Fe) treatment. The radicles were clearly longer in the •OH(Fe)-treated seeds. The differences were visible at 36 h through to 72 h. The opening and greening of the cotyledon started earlier in the •OH(Fe)-treated seeds than the control (Figure S5a).
The treatment effect is sustained beyond germination
The difference in early seedling growth after 72 h indicated the •OH(Fe) treatment had a relatively long-term effect on seedling growth. Even though we observed the growth in both shoot part (hypocotyl and cotyledon) and the root part, in the early stages the growth was more visible and quantifiable in the roots. Therefore, root growth and lateral root numbers were measured to compare the effect between the control and treated group.
The root growth difference between seedlings from non-treated control and •OH(Fe)-treated seeds was recorded from day 6 to day 9 after transfer into the growth chamber. The difference between the root growth of the control and the treatment group was always significant (P < 0.05) from day 6 to day 9 ()). There was also a significant difference in the total length of roots between the two groups after 9 days of germination ()).
Figure 2. Effect of the treatment on Arabidopsis seedling growth. (a) Daily root growth from day 6 to day 9, (b) total root length after 9 days, and (c) the number of lateral roots per plant after 10 days. The asterisk indicates a significant P < 0.05) difference between conditions obtained by the Student’s t-test.

In addition, we made an interesting observation of lateral root numbers. When the lateral roots per plant were counted on day 10, the •OH(Fe)-treated group had a significantly (P < 0.05) larger number of lateral roots than the control group ()). The treated seedlings had an average number of >12, while the control seedlings had only an average number of 10 lateral roots per plant () and S5b). On day 10, the lateral roots in the •OH(Fe)-treated group also looked longer and denser than the control group (Figure S5b).
The effect of the hydroxyl radical treatment is prominent under abiotic stress
After observing a clear and significant difference between the water treatment (control) and ROS treatments (•OH and H2O2) on Arabidopsis seed and seedling performance under ambient conditions, the treatment effects were tested under various abiotic stresses such as chilling, high temperature, heat, and salinity.
The speed of Arabidopsis seed germination decreased dramatically under the chilling condition at 11°C, but •OH(Fe) or •OH(Cu) treatment had a prominent effect under this condition ()). The treated group germinated significantly (P < 0.05) faster than both the control and H2O2-treated group ()) and took a significantly shorter time (P < 0.05) to reach 50% germination (). The •OH(Fe/Cu)-treated seeds showed a prominent tolerance effect under this condition and showed improved gMAX, U7525, MGT, and AUC compared with the control and H2O2-treated seeds (Figure S6a-d). However, there was no significant difference in U7525 between the Fenton- and H2O2-treated seeds (Figure S6b). The effect of the treatment was also observed in early seedling growth after 6 days with longer radicle and opened cotyledon in the treated group than the controls (Figure S7a).
Table 1. Comparison of the 50% germination time (t50) among four different stresses generated using 4PHF from the Germinator package (asterisk indicates the germination temperate 23ºC).
Figure 3. Germination rate of Arabidopsis under different abiotic stresses. (a) Chilling stress (11°C), (b) high-temperature stress (30°C), (c) heat stress (50°C, 1 h), and (d) salinity stress (150 mM NaCl). The asterisk indicates a significant (P < 0.05) difference between conditions obtained by the Student’s t-test.

Compared with low temperature, incubation of seeds at high temperature (30°C) increased the germination speed, although it was not as fast as the germination at ambient temperature (23°C) ()). Even though the •OH(Fe/Cu)-treated seeds took significantly less time than the control seeds, there was no significant difference between treated seeds with different combinations (•OH(Fe) and H2O2) (). The gMAX for the •OH(Fe/Cu)-treated was higher than the control group, but there was no significant difference between either control and H2O2- treated seeds or •OH(Fe/Cu)-treated and H2O2- treated seeds (Figure S6e). U7525, MGT, and AUC of ROS-treated seeds showed a significant difference with that of the control seeds (Figure S6f-h). Furthermore, there was a clear difference in seedling growth; cotyledon opening and radicle growth were faster in all ROS-treated groups than the control (Figure S7b).
Although the speed of germination was greatly reduced after heat stress at 50°C for 65 min, the ROS treatment improved the germination performance significantly () and S6i). The germination rates of the treated seeds reached 50% more than 10 h earlier than that of the control; 61.5 h and 62.1 h for •OH(Fe) and •OH(Cu), respectively, compared with over 71.6 h for the control (). The difference was only a few hours between the •OH(Fe/Cu)-treated and H2O2-treated seeds (). MGT was also significantly smaller in comparison to the control and H2O2-treated seeds (Figure S6k). There was no significant difference in germination uniformity (U7525) observed in control vs. •OH(Fe/Cu and H2O2 vs. •OH(Fe/Cu) (Figure S6j). Because the experiments were carried out in the dark, the cotyledons were elongated. However, cotyledon-expansion and -greening, hypocotyl, and radical elongation were advanced in all of the treated seeds, and the difference was visible 72 h after transfer to the chamber (Figure S7c).
Similar to chilling and heat stress, salinity stress decreased the germination performance, but the •OH treatment improved seed germination ()). The •OH(Fe/Cu)-treated seeds performed much better than the control and the H2O2-treated seeds under salinity stress, and the extent of the effect was larger at 150 mM than at 120 mM NaCl (Table S1). At 150 mM NaCl, the •OH(Fe/Cu)-treated seeds reached 50% germination earlier than both the control and the H2O2-treated seeds; •OH(Fe/Cu)-treated seeds took around 16 h less than the control and 7 h less than the H2O2-treated seeds (). Except for the germination uniformity where the differences were close to each other among the groups, the gMAX, AUC, and MGT were significantly better in the •OH(Fe/Cu)-treated seeds (Figure S6m-p). Both the germination and the early seedling growth were accelerated by the •OH(Fe/Cu) treatment under salinity stress: opened and green cotyledon, longer radicles are clearly visible in the treated groups (Figure S7d).
The 4PHF can be used to extract biologically relevant information using the surface area under each germination curve, which is expressed as AUC in a short form. As mentioned by Joosen et al. [Citation55], the AUC can be used to quantify the effect of any treatment under different stress conditions as an index of stress. As described in the methods section, by deducting the AUCs extracted from the solver for the control and ROS-treated groups (ΔAUC = ΔAUCROS − ΔAUCControl) under all four abiotic stress conditions, the SI was calculated. The data in ) show clearly that •OH(Fe/Cu)-treated seeds had a larger ΔAUC. There was no difference in the AUCs of the •OH(Fe)- and •OH(Cu)-treated seeds, even though the AUC of the •OH(Cu)-treated seeds was slightly larger than that of the •OH(Fe)-treated seeds ()). SI for the •OH(Fe)-treated seeds was plotted against the original germination percentage under every stress and a significant correlation was found for each stress ()): chilling stress, r2 = 0.983; high temperature stress, r2 = 0.994; heat stress, r2 = 0.972; salinity stress, r2 = 0.981. The SI for the •OH(Cu)-treated seeds also showed a similar correlation with the germination percentage under every stress (data not shown).
Figure 4. Seed germination indices (ΔAUC and SI) for Arabidopsis seeds germinated under abiotic stresses.) ΔAUC and SI were calculated at different time points, as described in the text. (a and b) Chilling stress (11°C), (c and d) High-temperature stress (30°C), (e and f) heat stress (50°C, 1 h), and (g and h) salinity stress (150 mM NaCl). The asterisk indicates a significant P < 0.05) difference between •OH(Fe/Cu) and H2O2 obtained by the Student’s t-test.

The •OH treatment also improves the performance of a crop plant: rice
The promising effect of the •OH(Fe/Cu) treatment on performance in the model plant Arabidopsis seed directed our attention to its use on an important crop plant such as rice. The Fe-based •OH(Fe/Cu) treatment showed a similar effect on rice seeds under non-stressed conditions; it increased the germination rate as well as the speed of germination ()). The time for 50% germination of the •OH(Fe/Cu)-treated seeds was significantly shorter than for the water control ()). Furthermore, early growth, as determined by radicle length was significantly larger in the •OH(Fe/Cu)-treated seeds than in the control seeds ()).
Figure 5. Effect of the •OH(Fe)-treatment in rice seeds. (a) Germination, (b) time for 50% germination t50, and (c) radicle growth at 48th hours. The asterisk indicates a significant P < 0.05, Student’s t-test) difference between control and other treatments.

As a case study to investigate the effect of the •OH(Fe/Cu) treatment on rice seed germination under stressed conditions, we applied salinity stress (25–200 mM NaCl) to seeds germinated at 30°C. The results were similar to what we found in the Arabidopsis seeds; the •OH(Fe/Cu) treatment accelerated rice seed germination under salinity stress. From 25 to 200 mM NaCl, the •OH(Fe/Cu)-treated seeds performed better than the control in every case (Figures S8 and 6(a)). Furthermore, the effect at higher NaCl concentrations (100–200 mM) was more prominent; the seeds germinated faster according to the 48-h germination rate and the time to 50% germination ()). In addition, similar to what we observed in the Arabidopsis seed germination, ΔAUC was also larger at 100 mM and 150 mM NaCl ().
Figure 6. Improvement of salinity stress tolerance by the •OH(Fe)-treatment in rice. (a) Germination rate at different NaCl concentrations, (b) time to reach 50% germination t50, and (c) SI at different time points under different NaCl concentrations. The asterisk indicates a significant P < 0.05) difference between conditions obtained by the Student’s t-test. Seeds were incubated with 100 mM (S100), 150 mM (S150), and 200 mM (S200) NaCl.

Under abiotic stress conditions, in both Arabidopsis and rice, the seeds in the control group could never reach a similar germination percentage to the treated group ( d), S7 and 6). For example, in rice when the stress level was high (200 mM NaCl), the treated seeds reached over 95% germination, where the controls were below 70% at the 48th hour (). Furthermore, by the time the control groups reached their full germination potential, the treated groups already started other growth and developmental stages such as growth in hypocotyl and radicle, and opening and greening in cotyledons (Figure S7).
Discussion
A cost-effective, non-laborious single seed treatment that improves germination, growth performance, and tolerance against multiple abiotic stresses, and could contribute to an increase in crop production, may provide an essential tool for the adaptation to the emerging/ongoing threat of global climate change to agriculture. In this study, we demonstrated that a single seed (Arabidopsis and rice) treatment with H2O2 and a metal catalyst such as Fe or Cu, which quickly produces hydroxyl radicals (•OH) in the system, resulted in faster germination under both ambient and different abiotic stress conditions. It also improved the growth performance of young seedlings (root growth and lateral root numbers). In addition, interestingly, the treatment improved seed and seedling performance under abiotic stress conditions, including chilling, high temperature, heat, and salinity stresses. The positive effects of this treatment on rice seedlings indicate a potential use for this treatment in major crops.
The •OH treatment accelerated germination speed (t50) in both Arabidopsis and rice seeds under ambient temperature (23°C and 30°C, respectively) ()). The ΔAUC or germination speed index (GSI) significantly correlated (r2 > 0.9) with the germination percentage at different time points, indicating that the acceleration of germination was due primarily to the treatment effect of ROS and the metal catalyst in the treatment solution rather than random chance. This correlation fits with the study by El-Kassaby et al [Citation56]., who used a similar approach to quantify the effect of cold stratification on seed germination and dormancy breaking using the term dormancy index (DI). The positive effect of the •OH treatment was further proved by washing the seeds after •OH(Fe)-treatment, where the washed seeds [•OH(Fe)+W] also showed similar germination profile as the unwashed seeds ()). Furthermore, the speed of germination is a critical factor that provides a sensitive measurement of seed vigor [Citation52]. Thus, the shorter t50 and larger GSI determined in this study imply that the treatment increases seed vigor. This is consistent with several previously reported studies, where the exogenous application of •OH on seeds, directly via Fenton reagents [Citation44] and indirectly via nanobubble water containing •OH [Citation58], improved germination.
In addition to the effect observed under ambient temperature conditions (at 23°C for Arabidopsis and at 30°C for rice), one of the most interesting findings of this study was the improvement of seed germination and early growth under different stress conditions. In Arabidopsis, the treatments with ROS (•OH and H2O2) showed superior performance to the controls under all stress conditions employed. The faster germination, larger AUC and SI, and accelerated seedling growth (longer hypocotyl and radical and early cotyledon-expansion and -greening) indicate that the treatment improves stress tolerance in germinating seeds and young seedlings. Although several previous studies have shown that pre-soaking/treating seeds with H2O2 improves germination, growth performance, and stress tolerance by enhancing the performance of the antioxidative machinery, maintaining turgor pressure, inducing stress protein expression, and osmotic readjustment [Citation59–61], there is, to our knowledge, no evidence that exogenous •OH treatment results in similar and in most cases superior improvements under abiotic stress.
The effect also continued beyond the germination stage to the early radicle growth stage in both Arabidopsis and rice. The effect of the treatment at much later stages was shown by an increase in daily root growth and in the number of lateral roots in Arabidopsis. Several reports have shown that exogenous application of •OH accelerates elongation growth in maize coleoptiles [Citation42,Citation43]. These studies reported that the auxin-mediated ROS generation facilitates this extension growth. The final ROS that is generated by the action of auxin is •OH, which acts on the cell wall to breakdown polysaccharide crosslinking and loosens the tightness of the cell wall. Renew et al. also reported that the plasma membrane-bound NADPH oxidase (NOX) enzyme generates apoplastic superoxide radicals (O2•−). O2•− is a precursor of H2O2, and apoplastic metal catalysts (Fe/Cu) can easily convert H2O2 into •OH and then facilitate elongation growth similarly to the exogenous application [Citation62,Citation63]. Exogenous application of H2O2 can also restore the formation of lateral roots in the mutants that do not produce lateral roots [Citation64]. Unlike previous studies, only the seeds were treated in this study, and no other treatment was applied to plants in later stages. Because ROS mediate signal transduction in plants [Citation20,Citation37,Citation39,Citation65,Citation66], it is possible that the treatment with ROS (•OH and H2O2) might affect a signaling mechanism that the plants carry from the germination stage to later stages, such as lateral root formation. In addition, we cannot rule out the possibility that the accelerated seed germination and root growth might partially come from the seed coat and cell wall weakening by the action of exogenous •OH [Citation44].
Moreover, apoplastic •OH is involved in the salt overly sensitive (SOS) signaling pathway for adaptive growth under salinity stress, where •OH is generated by plasma membrane-bound NOX [Citation67]. Under salt stress, Na+ uptake increases the cytosolic Ca2+ level, which activates the membrane-bound NOX to convert O2•− into •OH via H2O2 [Citation68]. As we discussed in the previous sections, •OH can facilitate germination [Citation44] and elongation growth [Citation42,Citation43,Citation63,Citation69]. Thus, it is possible that the seed treatment in this study using ROS (•OH via H2O2) triggered the SOS signaling and elongation growth mechanism to facilitate germination and early seedling growth under salt stress conditions in both Arabidopsis and rice.
In plant cells, H2O2 can react with the cell wall, cell membrane, organellar membrane-bound, or cytosolic soluble metal ions and converts into •OH in a downstream reaction [Citation70–72]. Thus •OH may play an important, positive role that contrasts with its well-established damaging properties. Hence, exogenous application of •OH at an optimum concentration may provide a head start to the supply of •OH in seed germination and early seedling growth processes. Another possible explanation of the effect could be that the treatment provides a cue to seeds to advance their physiological state. This advanced physiological state allows the embryo to start developmental processes, such as germination, earlier than the controls, which might be similar to the priming effect. The same explanation may fit with the improved stress tolerance, with the embryo sensing the exogenous ROS as a stress and activating the stress tolerance machinery in advance. A report showed that, during priming, the initial soaking agents can generate mild to moderate stress, which can enhance tolerance to abiotic stress by activating stress-responsive genes and proteins [Citation73]. A preliminary seed proteomic study under chilling stress (data not shown) showed that the •OH-treated seeds had increased abundance of monodehydroascorbate reductase, catalase, reduced glutathione, glutathione S-transferase, and other ROS-scavenging proteins. This indicates that similar to exogenous H2O2 treatment, •OH activates the ROS-scavenging system under stress conditions. However, further detailed studies are required to elucidate the mechanism.
Decades of research showed that ROS works mostly in a dose-dependent manner; a high concentration of ROS can cause cell death or oxidative stress, low concentration can be cytostatic that can slow down growth and development. On the other hand, a balanced scale of ROS can work as a signaling molecule for growth, development, and stress [Citation20,Citation39]. Although individual abiotic stresses differ significantly in their signaling and response systems, they share a number of common features in signaling pathways and resultant physiological responses via crosstalk. One such common component is ROS [Citation53]. This was further shown by the observations in this study that ROS treatment of seeds improved germination and early growth performance under chilling, high temperature, heat, and salinity stress, possibly via a common mechanism in which ROS plays a central role. We successfully developed a seed treatment method that is easy to apply and non-laborious involving an endogenous rather than a synthetic chemical, which improves seed and seedling performance under both ambient and stress conditions. The accelerated germination, larger roots, increased lateral roots, and enhanced stress tolerance enables plants to establish under various environmental conditions. This study opens up a new application and positive roles of •OH, which is well known as a dangerous byproduct of aerobic respiration. Further technological advancements in imaging, detecting, and tracking •OH inside plant cells will reveal how, when, and where •OH works under different conditions. In addition, a comprehensive molecular study to elucidate which genes and proteins are involved in the improved seed and seedling performance due to •OH treatment will provide a better understanding of the mechanisms to improve crop management. Finally, treatment at the seed stage will save farmers time, labor, and money, and with further field trials, this treatment could be a potential solution to improve crop performance in the face of upcoming unfavorable climate conditions.
Author contribution
Md Mostafa Kamal designed the research, performed the experiments, analyzed data, and took the lead in manuscript writing. Carlos Erazo performed the experiments and discussed on the experiments. Karen K. Tanino, Bernard Laarveld, Andrew Olkowski developed the seed treatment methods and discussed on the experiments. Yukio Kawamura and Jun Kasuga helped supervise the project and discussed on the experiments. Matsuo Uemura designed the research and supervised and took the lead for the project. All authors read and contributed to the manuscript at various stages of the preparation.
Final_Kamal_Supplemental_Data_All.pdf
Download PDF (2.9 MB)Acknowledgments
We are grateful to Dr. Abidur Rahman (Iwate University) for discussion throughout the study, and Yuko Suzuki, Michiko Saito, Nozomi Yokota, Momo Otake, Runa Tamura, Aoi Sawada, and Kei Onodera for their technical help to grow plants. We thank Robbie Lewis, MSc, from Edanz Group (www.edanzediting.com/ac) for editing a draft of this manuscript.
Disclosure statement
The authors have no conflicts of interest to declare.
Supplementary material
Supplemental data for this article can be accessed here.
Additional information
Funding
References
- Perdomo JA, Conesa MA, Medrano H, et al. Effects of long-term individual and combined water and temperature stress on the growth of rice, wheat and maize: relationship with morphological and physiological acclimation. Physiol Plant. 2015;155(2):149–165.
- Silva EN, Vieira SA, Ribeiro RV, et al. Contrasting physiological responses of Jatropha curcas plants to single and combined stresses of salinity and heat. J Plant Growth Regul. 2012;32(1):159–169.
- Suzuki N, Rivero RM, Shulaev V, et al. Abiotic and biotic stress combinations. New Phytol. 2014;203(1):32–43.
- Downing TE. The effects of climate change on agriculture and food security. Renew Energy. 1993;3(4–5):491–497.
- Duke SH, Schrader LE, Miller MG. Low temperature effects on soybean (Glycine max [L.] Merr. cv. Wells) mitochondrial respiration and several dehydrogenases during imbibition and germination. Plant Physiol. 1977;60(5):716–722.
- Hatfield JL, Egli DB. Effect of temperature on the rate of soybean hypocotyl elongation and field emergence. Crop Sci. 1974;14(3):423–426.
- Leopold AC. Temperature effects on soybean imbibition and leakage. Plant Physiol. 1980;65(6):1096–1098.
- Gill PK, Sharma AD, Singh P, et al. Changes in germination, growth and soluble sugar contents of Sorghum bicolor (L.) Moench seeds under various abiotic stresses. Plant Growth Regul. 2003;40(2):157–162.
- Khan MSA, Hamid A, Karim MA. Effect of sodium chloride on germination and seedling characters of different types of rice (Oryza sativa L.). J Agron Crop Sci. 1997;179(3):163–169.
- Zhang WJ, Niu Y, Bu SH, et al. Epistatic association mapping for alkaline and salinity tolerance traits in the soybean germination stage. PLoS One. 2014;9(1):e84750.
- Kan G, Ning L, Li Y, et al. Identification of novel loci for salt stress at the seed germination stage in soybean. Breed Sci. 2016;66(4):530–541.
- Mickky BM, Aldesuquy HS. Impact of osmotic stress on seedling growth observations, membrane characteristics and antioxidant defense system of different wheat genotypes. Egypt J Basic Appl Sci. 2019;4(1):47–54.
- Pratap V, Sharma YK. Impact of osmotic stress on seed germination and seedling growth in black gram (Phaseolus mungo). J Environ Biol. 2010;31(5):721–726.
- Wright E. The effect of high temperatures on seed germination. J For. 1931;29(5):679–687.
- Pagamas P, Nawata E. Effect of high temperature during the seed development on quality and chemical composition of chili pepper seeds. Jpn J Trop Agr. 2007;51(1):22–29.
- Boyer JS, Westgate ME. Grain yields with limited water. J Exp Bot. 2004;55(407):2385–2394.
- Prasad PVV, Pisipati SR, Momčilović I, et al. Independent and combined effects of high temperature and drought stress during grain filling on plant yield and chloroplast EF-Tu expression in spring wheat. J Agron Crop Sci. 2011;197(6):430–441.
- Maraghni M, Gorai M, Neffati M. Seed germination at different temperatures and water stress levels, and seedling emergence from different depths of Ziziphus lotus. S Afr J Bot. 2010;76(3):453–459.
- Lobell DB, Field CB. Global scale climate–crop yield relationships and the impacts of recent warming. Environ Res Lett. 2007;2(1):014002.
- Mittler R. Abiotic stress, the field environment and stress combination. Trends Plant Sci. 2006;11(1):15–19.
- Ray DK, Gerber JS, MacDonald GK, et al. Climate variation explains a third of global crop yield variability. Nat Commun. 2015;6:5989.
- Xiao Z, Ximing C. Climate change impacts on global agricultural land availability. Environ Res Lett. 2011;6(1):014014.
- Pandey P, Irulappan V, Bagavathiannan MV, et al. Impact of combined abiotic and biotic stresses on plant growth and avenues for crop improvement by exploiting physio-morphological traits. Front Plant Sci. 2017;8:537.
- Ahanger MA, Akram NA, Ashraf M, et al. Plant responses to environmental stresses-from gene to biotechnology. AoB Plants. 2017;9(4):plx025.
- Savvides A, Ali S, Tester M, et al. Chemical priming of plants against multiple abiotic stresses: mission possible? Trends Plant Sci. 2016;21(4):329–340.
- Zhang JZ, Creelman RA, Zhu JK. From laboratory to field: using information from Arabidopsis to engineer salt, cold, and drought tolerance in crops. Plant Physiol. 2004;135(2):615–621.
- Chen K, Arora R. Dynamics of the antioxidant system during seed osmopriming, post-priming germination, and seedling establishment in spinach (Spinacia oleracea). Plant Sci. 2011;180(2):212–220.
- Chen K, Fessehaie A, Arora R. Dehydrin metabolism is altered during seed osmopriming and subsequent germination under chilling and desiccation in Spinacia oleracea L. cv. Bloomsdale: possible role in stress tolerance. Plant Sci. 2012;183:27–36.
- Gill SS, Tuteja N. Polyamines and abiotic stress tolerance in plants. Plant Signal Behav. 2010;5(1):26–33.
- Hossain MA, Bhattacharjee S, Armin SM, et al. Hydrogen peroxide priming modulates abiotic oxidative stress tolerance: insights from ROS detoxification and scavenging. Front Plant Sci. 2015;6:420.
- Parra-Lobato MC, Gomez-Jimenez MC. Polyamine-induced modulation of genes involved in ethylene biosynthesis and signalling pathways and nitric oxide production during olive mature fruit abscission. J Exp Bot. 2011;62(13):4447–4465.
- Tanou G, Molassiotis A, Diamantidis G. Hydrogen peroxide- and nitric oxide-induced systemic antioxidant prime-like activity under NaCl-stress and stress-free conditions in citrus plants. J Plant Physiol. 2009;166(17):1904–1913.
- Li L, Wang Y, Shen W. Roles of hydrogen sulfide and nitric oxide in the alleviation of cadmium-induced oxidative damage in alfalfa seedling roots. Biometals. 2012;25(3):617–631.
- Bethke PC, Jones RL. Cell death of barley aleurone protoplasts is mediated by reactive oxygen species. Plant J. 2001;25(1):19–29.
- Kimura S, Kaya H, Kawarazaki T, et al. Protein phosphorylation is a prerequisite for the Ca2+-dependent activation of Arabidopsis NADPH oxidases and may function as a trigger for the positive feedback regulation of Ca2+ and reactive oxygen species. Biochim Biophys Acta. 2012;1823(2):398–405.
- Kobayashi M, Ohura I, Kawakita K, et al. Calcium-dependent protein kinases regulate the production of reactive oxygen species by potato NADPH oxidase. Plant Cell. 2007;19(3):1065–1080.
- Miller G, Schlauch K, Tam R, et al. The plant NADPH oxidase RBOHD mediates rapid systemic signaling in response to diverse stimuli. Sci Signal. 2009;2(84):ra45.
- Queval G, Issakidis-Bourguet E, Hoeberichts FA, et al. Conditional oxidative stress responses in the Arabidopsis photorespiratory mutant cat2 demonstrate that redox state is a key modulator of daylength-dependent gene expression, and define photoperiod as a crucial factor in the regulation of H2O2-induced cell death. Plant J. 2007;52(4):640–657.
- Mittler R. ROS are good. Trends Plant Sci. 2017;22(1):11–19.
- Richards SL, Wilkins KA, Swarbreck SM, et al. The hydroxyl radical in plants: from seed to seed. J Exp Bot. 2015;66(1):37–46.
- Liszkay A, van der Zalm E, Schopfer P. Production of reactive oxygen intermediates (O2·-, H2O2, and ·OH) by maize roots and their role in wall loosening and elongation growth. Plant Physiol. 2004;136(2):3114–3123.
- Schopfer P, Liszkay A, Bechtold M, et al. Evidence that hydroxyl radicals mediate auxin-induced extension growth. Planta. 2002;214(6):821–828.
- Schopfer P. Hydroxyl radical-induced cell-wall loosening in vitro and in vivo: implications for the control of elongation growth. Plant J. 2001;28(6):679–688.
- Muller K, Linkies A, Vreeburg RA, et al. In vivo cell wall loosening by hydroxyl radicals during cress seed germination and elongation growth. Plant Physiol. 2009;150(4):1855–1865.
- Olkowski AA, Laarveld B, Tanino KK, inventors; University of Saskatchewan, assignee. Enhancement and control of seed germination with compositions comprising a transition metal catalyst and an oxidant (CA2949750A1). Canada; 2014.
- Matros A, Peshev D, Peukert M, et al. Sugars as hydroxyl radical scavengers: proof-of-concept by studying the fate of sucralose in Arabidopsis. Plant J. 2015;82(5):822–839.
- Vreeburg RA, Airianah OB, Fry SC. Fingerprinting of hydroxyl radical-attacked polysaccharides by N-isopropyl-2-aminoacridone labelling. Biochem J. 2014;463(2):225–237.
- Khorobrykh SA, Khorobrykh AA, Yanykin DV, et al. Photoproduction of catalase-insensitive peroxides on the donor side of manganese-depleted photosystem II: evidence with a specific fluorescent probe. Biochemistry. 2011;50(49):10658–10665.
- Galano A, Macias-Ruvalcaba NA, Medina Campos ON, et al. Mechanism of the OH radical scavenging activity of nordihydroguaiaretic acid: a combined theoretical and experimental study. J Phys Chem B. 2010;114(19):6625–6635.
- Deng S, Yu M, Wang Y, et al. The antagonistic effect of hydroxyl radical on the development of a hypersensitive response in tobacco. Febs J. 2010;277(24):5097–5111.
- Uemura M, Joseph RA, Steponkus PL. Cold acclimation of Arabidopsis thaliana: effect on plasma membrane lipid composition and freeze-induced lesions. Plant Physiol. 1995;109(1):15–30.
- Hacisalihoglu G, Paine DH, Hilderbrand MB, et al. Embryo elongation and germination rates as sensitive indicators of lettuce seed quality: priming and aging studies. HortScience. 1999;34(7):1240–1243.
- Mastouri F, Bjorkman T, Harman GE. Seed treatment with Trichoderma harzianum alleviates biotic, abiotic, and physiological stresses in germinating seeds and seedlings. Phytopathology. 2010;100(11):1213–1221.
- Silva-Correia J, Freitas S, Tavares RM, et al. Phenotypic analysis of the Arabidopsis heat stress response during germination and early seedling development. Plant Methods. 2014;10(1):7.
- Joosen RV, Kodde J, Willems LA, et al. GERMINATOR: a software package for high-throughput scoring and curve fitting of Arabidopsis seed germination. Plant J. 2010;62(1):148–159.
- El-Kassaby YA, Moss I, Kolotelo D. et al. Seed germination: mathematical representation and parameters extraction. For Sci. 2008;54(2):220–227.
- Soltani E, Ghaderi-Far F, Baskin CC, et al. Problems with using mean germination time to calculate rate of seed germination. Aust J Bot. 2015;63(8):631–635.
- Liu S, Oshita S, Kawabata S, et al. Identification of ROS produced by nanobubbles and their positive and negative effects on vegetable seed germination. Langmuir. 2016;32(43):11295–11302.
- Gondim FA, Gomes-Filho E, Lacerda CF, et al. Pretreatment with H2O2 in maize seeds: effects on germination and seedling acclimation to salt stress. Braz J Plant Physiol. 2010;22:103–112.
- Liheng H, Zhiqiang G, Runzhi L. Pretreatment of seed with H2O2 enhances drought tolerance of wheat (Triticum aestivum L.) seedlings. Afr J Biotechnol. 2009;8(22):6151–6157.
- Wahid A, Perveen M, Gelani S, et al. Pretreatment of seed with H2O2 improves salt tolerance of wheat seedlings by alleviation of oxidative damage and expression of stress proteins. J Plant Physiol. 2007;164(3):283–294.
- Foreman J, Demidchik V, Bothwell JH, et al. Reactive oxygen species produced by NADPH oxidase regulate plant cell growth. Nature. 2003;422(6930):442–446.
- Fry SC. Oxidative scission of plant cell wall polysaccharides by ascorbate-induced hydroxyl radicals. Biochem J. 1998;332((Pt 2)(2)):507–515.
- Orman-Ligeza B, Parizot B, de Rycke R, et al. RBOH-mediated ROS production facilitates lateral root emergence in Arabidopsis. Development. 2016;143(18):3328–3339.
- Baxter A, Mittler R, Suzuki N. ROS as key players in plant stress signalling. J Exp Bot. 2014;65(5):1229–1240.
- Mittler R, Blumwald E. The roles of ROS and ABA in systemic acquired acclimation. Plant Cell. 2015;27(1):64–70.
- Laohavisit A, Richards SL, Shabala L, et al. Salinity-induced calcium signaling and root adaptation in Arabidopsis require the calcium regulatory protein annexin1. Plant Physiol. 2013;163(1):253–262.
- Demidchik V, Cuin TA, Svistunenko D, et al. Arabidopsis root K+-efflux conductance activated by hydroxyl radicals: single-channel properties, genetic basis and involvement in stress-induced cell death. J Cell Sci. 2010;123(Pt 9):1468–1479.
- Renew S, Heyno E, Schopfer P, et al. Sensitive detection and localization of hydroxyl radical production in cucumber roots and Arabidopsis seedlings by spin trapping electron paramagnetic resonance spectroscopy. Plant J. 2005;44(2):342–347.
- Pospisil P. Production of reactive oxygen species by photosystem II. Biochim Biophys Acta. 2009;1787(10):1151–1160.
- Nagasaki-Takeuchi N, Miyano M, Maeshima M. A plasma membrane-associated protein of Arabidopsis thaliana AtPCaP1 binds copper ions and changes its higher order structure. J Biochem. 2008;144(4):487–497.
- Schwarzlander M, Finkemeier I. Mitochondrial energy and redox signaling in plants. Antioxid Redox Signal. 2013;18(16):2122–2144.
- Kubala S, Garnczarska M, Wojtyla L, et al. Deciphering priming-induced improvement of rapeseed (Brassica napus L.) germination through an integrated transcriptomic and proteomic approach. Plant Sci. 2015;231:94–113.