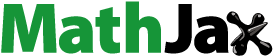
ABSTRACT
Bovine αS1-casein (αS1-CN) is the main allergen in cow's milk, and immunoglobulin E (IgE) epitopes are the material basis to trigger allergic reactions. Thus, IgE epitopes could be as key targets for precise αS1-CN detection and allergenicity assessment. Here, we designed a tandem containing nine IgE linear epitopes of αS1-CN (tαS1-CN), expressed the recombinant tαS1-CN in Escherichia coli, and prepared the rabbit polyclonal antibody against recombinant tαS1-CN (pAb-tαS1-CN). The obtained recombinant tαS1-CN had a purity of 94.43% and retained the antigenicity and potential allergenicity of IgE epitopes. The pAb-tαS1-CN could recognize the nine IgE epitopes of αS1-CN, and also showed high specificity. The ability of pAb-tαS1-CN to evaluate the potential allergenicity of milk in foods was validated by sera from cow's milk allergy patients. These findings indicate that our prepared pAb-tαS1-CN may represents a valuable tool for the evaluation of milk allergenicity in processed foods.
Introduction
Food allergy is a serious food safety issue, affecting up to 10% of the global population (Sicherer & Sampson, Citation2018). Cow's milk allergy (CMA) is one of the most common food allergies in childhood, affecting 0.5%–3% of children under the age of 1 (Flom & Sicherer, Citation2019). Therefore, detection of milk allergen and evaluation of milk allergenicity are of great significance for controlling milk allergy. Immunoglobulin E (IgE) epitopes are the main causes of CMA, and milk allergenicity depends on the amount and stability of IgE epitopes in foods recognized by CMA patients (Huang et al., Citation2023). Thus, targeting IgE epitopes of milk allergen has a dual functionality: (1) detecting the presence of the given allergen in foods and (2) estimating the potential allergenicity of the allergen in food products (Orcajo et al., Citation2019; Tasaniyananda et al., Citation2018). Therefore, preparing an antibody that specifically recognizes IgE epitopes of milk allergen is important to precisely detect the allergen and evaluate its potential allergenicity in the given food products (He et al., Citation2016).
Bovine β-lactoglobulin and αS1-casein (αS1-CN), the most abundant proteins in whey and casein (CN) respectively, are the most common allergens (Giannetti et al., Citation2021). Currently, several antibodies that specifically recognize IgE epitopes of β-lactoglobulin have been prepared and are used to analyze the content of β-lactoglobulin and its allergenic residues in dairy products (He et al., Citation2016; He, Li, Gao et al., Citation2018; Knipping et al., Citation2014; He, Li, Wu et al., Citation2018; Orcajo et al., Citation2019). However, only a few immunoassays have been reported to detect αS1-CN, and the antibodies used did not specifically recognize the IgE epitopes of αS1-CN (Muller-Renaud et al., Citation2005). In addition, CN is widely used in different food products. Therefore, only detecting β-lactoglobulin might not accurately reflect whether the given food contains milk allergens. Thus, a specific antibody against IgE epitopes of αS1-CN should be prepared for detecting αS1-CN and predicting its allergenicity in foods.
Milk allergenicity positively correlates with the content of milk IgE epitopes in food (Xu et al., Citation2020), but the epitopes might be destroyed during processing (Broersen, Citation2020; Zhang et al., Citation2022). A monoclonal antibody only binds one epitope. Therefore, when using monoclonal antibodies to detect allergens, the result may be underestimated and even generate a false negative result (Cai et al., Citation2016). On the other hand, polyclonal antibodies can recognize multiple epitopes, which could overcome this shortcoming (Mariager et al., Citation1994; Plebani et al., Citation1997). Therefore, in order to precisely detect the content of αS1-CN IgE epitopes and predict its potential allergenicity in foods, polyclonal antibodies against multiple IgE epitopes of αS1-CN need to be prepared.
Multiepitope-based peptide vaccine is a recombinant protein consisting of B cell epitope (IgE epitope), T cell epitope, and flexible linker (Aziz et al., Citation2022). Epitope-specific antibodies can be produced by immunizing animals (such as mice and rabbits) with this type of recombinant protein. For example, an artificial chimeric epitope tandem containing three copies of an ϵ-toxin B-cell epitope and universal T-cell epitope was expressed in Escherichia coli (E. coli), and high titres of neutralizing antibodies were obtained in immunized mice and rabbits (Singh, Prabhu et al., Citation2020). Accordingly, polyclonal antibodies against multiple IgE epitopes of αS1-CN can be prepared based on this method. In addition, the epitopes of αS1-CN have been mapped by many studies (http://www.iedb.org), which can provide epitope information to prepare polyclonal antibodies against IgE epitopes of αS1-CN.
The aim of this study is to design a tandem containing nine IgE linear epitopes of αS1-CN (tαS1-CN), produce recombinant tαS1-CN in E. coli, and prepare a specific polyclonal antibody (pAb-tαS1-CN) as a tool for predicting the potential allergenicity of milk in foods. We characterize the selectivity and IgE epitopes-binding ability of the developed pAb-tαS1-CN and validate the capacity of pAb-tαS1-CN to evaluate the potential allergenicity of mike in foods.
Materials and methods
Materials
Kanamycin and isopropyl β-D-1-thiogalactoside (IPTG) were purchased from Sangon Biotech (Shanghai, China). Tryptone and yeast extract were purchased from Oxoid (Hants, UK). Histrap HP affinity chromatography column was obtained from GE Healthcare (Uppsala, Sweden). CN from bovine milk, α-lactalbumin, β-lactoglobulin, complete Freund's adjuvant, incomplete Freund's adjuvant, horseradish peroxidase conjugated goat anti-rabbit IgG (HRP-IgG), and biotin labelled goat anti-human IgE (Bio-IgE) were purchased from Sigma (St. Louis, MO, USA). HRP-streptavidin was acquired from Neobioscience (Shenzhen, China). TMB kit was purchased from Thermo Fisher Scientific (Rockford, USA). Peptides (purity ≥ 95%, high performance liquid chromatography) were synthesized by Chinapeptides (Shanghai, China). All other reagents were of analytical grade and obtained from commercial sources. Ultra heat treated-milk (UHT milk), three kinds of yogurts, a beverage, a cake, and a cookie were purchased from local markets. The egg proteins, soybean proteins, wheat proteins, and oat proteins were prepared according to our previous study (He et al., Citation2017).
The native αS1-CN purified from bovine CN and pAb-αS1-CN were prepared by our group (He et al., Citation2022). CMA patients’ sera were collected from the Department of Dermatology, First Affiliated Hospital of Gannan Medical University (Ganzhou, China) with the informed consent of the patients, and stored at −80°C until use. The information of CMA patients’ sera is shown in .
Table 1. Information of cow's milk allergy patients.
Design of tαS1-CN
The tαS1-CN was designed according to the method used in our previous report (He et al., Citation2016). In short, nine IgE-binding linear epitopes of native αS1-CN, namely, B1, B2, B3, B4, B5, B6, B7, B8, and B9, were selected as part of the tandem according to Chatchatee's work (Chatchatee et al., Citation2001) and a main T cell epitope (AA91–110) was also selected (Ruiter et al., Citation2006). All epitope sequences are shown in . To design the tαS1-CN, the T cell epitope was located in N-terminal, and glycine-glycine-glutamate (GGE) was inserted as a linker between T cell epitope and its adjacent B cell epitope, while glycine-glycine (GG) was inserted between the B cell epitopes (Fang et al., Citation2012). The structure of the epitope tandem is shown in Figure S1. Hydrophilicity, antigenicity, and surface accessibility scores of tαS1-CN were predicted by DNAStar software, and the secondary structure of tαS1-CN was predicted by network server-SOMPA. If the scores of hydrophilicity and antigenicity were more than 0 and the value of surface accessibility was more than 1, the peptides with β-turn or random coil regions were more likely to be epitopes in the tandem.
Table 2. The amino acid sequences of nine B cell epitopes, one T cell epitope of tαS1-CN, and the blocking peptides.
Expression of recombinant tαS1-CN
The tαS1-CN gene was synthesized and cloned into pET-28a(+) vector with 6 × His-tag, and then the pET-28a(+)-tαS1-CN was transformed into E. coli BL21 (DE3) pLysS cells by Chinapeptides (Shanghai, China). The recombinant E. coli was inoculated in 5 mL of Luria–Bertani (LB) medium containing 50 µg/mL kanamycin and grown overnight at 37°C under shaking at 250 rpm. Then, the pre-cultured cells (2 mL) were refreshed in 100 mL of LB medium containing 50 µg/mL kanamycin and grown at 37°C under shaking at 250 rpm until the optical density (OD) at 600 nm reached 0.6−0.7. To optimize the expression parameters, different final concentrations of IPTG (0.1, 0.3, 0.7, 1, and 1.3 mM), incubation temperatures (37, 33, 30, 27, 23, and 20°C), and incubation times (2, 3, 4, 5, 6, 8, and 10 h) were investigated. The incubated cells were harvested by centrifugation at 12,000 × g for 5 min at 4°C, and the pellet was re-suspended in PBS. Then the cells were disrupted by sonication at 200 W for 5 min. After centrifugation at 12,000 × g for 10 min at 4°C, the precipitate was re-suspended in PBS-1 (20 mM PBS, containing 0.5 M NaCl, 2 M urea, and 0.1% Triton-100) and incubated at 25°C for 30 min with rotation. After washing with PBS-1 twice, the precipitate was re-suspended in PBS-2 (20 mM PBS, containing 0.5 M NaCl, 8 M urea, and 20 mM imidazole) and incubated at 25°C for 30 min with rotation. Finally, the supernatant was collected after centrifugation at 12,000 × g for 10 min at 4°C.
Purification of recombinant tαS1-CN
The recombinant tαS1-CN was purified by Histrap HP according to the manufacturer's protocol. First, the column was washed with 5 column volumes of distilled water, then equilibrated with 10 column volumes of binding buffer (20 mM PBS, containing 0.5 M NaCl and 20 mM iminazole, pH 7.4). The sample was loaded onto the column at a flow rate of 1 mL/min. The unbound constituent was washed by 20 column volumes of binding buffer. Then, 10 column volumes of elution buffer (20 mM PBS, containing 0.5 M NaCl and 500 mM iminazole, pH 7.4) was performed, and the eluted proteins (recombinant tαS1-CN) were collected and concentrated using an Amicon Ultra-15 centrifugal filter device (3 kDa) at 4°C with 4,200 × g. All solutions and samples were passed through 0.45 μm filters before loading onto the affinity chromatography column.
Preparation of polyclonal antibody
All animal-use protocols were approved by the Gannan Medical University Animal Care Committee under the guideline of the China Council for Animal Care (SYXK-Gan 2018–0004, China). Two eight-week-old male New Zealand white rabbits were purchased from the Ganzhou Institute of Animal Husbandry and fed with a milk-free diet. After acclimating for one week, each rabbit was subcutaneously immunized with 0.5 mL recombinant tαS1-CN (2 mg/mL) emulsified in 0.5 mL complete Freund's adjuvant. Then, each rabbit was re-immunized with 0.5 mL recombinant tαS1-CN (2 mg/mL) emulsified in 0.5 mL incomplete Freund's adjuvant on day 14, 28, 42, 56, respectively. One week after the last injection, rabbits were bled through the carotid artery under local anesthesia. The blood samples were clotted at 25°C for 1 h and centrifugated at 4,500 × g for 10 min at 4°C to collect serum. The serum (polyclonal antibody) was named pAb-tαS1-CN and stored at −80°C until use.
IgG and IgE binding abilities of recombinant tαS1-CN
The antigenicity and potential allergenicity of IgE epitopes preserved in recombinant tαS1-CN were tested by determining the binding abilities of recombinant tαS1-CN to IgG and IgE, respectively. The 96-well polystyrene microtiter plate (high binding; costar, Kennebunk, USA) was coated with 1 μg/mL of recombinant tαS1-CN or native αS1-CN in 50 mM sodium carbonate buffer (pH 9.6) and incubated overnight at 4°C.
To test the IgG binding ability of recombinant tαS1-CN, the pre-coated plate was washed thrice with PBS containing 0.05% Tween 20 (0.05% PBST). The plate was blocked with 3% gelatin in PBS for 1 h at 37°C. After further washing, 100 μL of pAb-tαS1-CN (diluted 1:20,000 in PBS) or pAb-αS1-CN (diluted 1:100,000 in PBS) was added and incubated for 1 h at 37°C. The wells were washed again, and 100 μL of HRP-IgG (diluted 1:10,000 in PBS) was added and incubated for 1 h at 37°C. After further washing, the plate was incubated with 100 μL TMB for 15 min at 37°C. Finally, 50 μL of 2 M sulfuric acid was added to stop the colour development, and the OD at 450 nm (OD450 nm) was recorded with a microplate reader (Varioskan LUX; Thermo Fisher Scientific, USA).
To test the IgE binding ability of recombinant tαS1-CN, the pre-coated plate was washed three times with 0.1% PBST and blocked with 3% gelatin in PBST for 1 h at 37°C. After being washed three times with PBST, 100 μL of sera from CMA patients (diluted 20 times in blocking buffer) was added to each well, followed by incubation at 37°C for 1 h, and washed again. Then, 100 μL of Bio-IgE (diluted 1:5000 in blocking buffer) was added to each well and incubated at 37°C for 1 h. After further washing, 100 μL of HRP-streptavidin (diluted 1:60 in blocking buffer) was added to each well and incubated at 37°C for 1 h. The subsequent procedures were identical to those used in the IgG binding ability test.
The titre of antisera was defined as the maximum dilution factor that yielded P/N > 2.1, and P > 0.2 (n = 3). P and N represented the OD of positive (antisera) and negative (PBS), respectively.
The pAb-tαS1-CN binding to IgE epitopes of native αS1-CN
Recognition of the IgE epitopes of native αS1-CN by pAb-tαS1-CN is an essential prerequisite for successfully detecting αS1-CN allergic residues and predicting potential milk allergenicity in food products. The binding ability of pAb-tαS1-CN to the IgE epitopes and blocking peptides was evaluated using competitive inhibition ELISA. The microtiter plate (plate-A) was pre-coated with 1 μg/mL of native αS1-CN and incubated overnight at 4°C, and coating buffer was used as blank control. Another plate (plate-B) was blocked with 3% gelatin, and, after washing three times with 0.05% PBST, 70 μL of pAb-tαS1-CN (diluted 1:20,000 in PBS) was mixed with 70 μL of peptide (1, 3, 9, and 27 μg/mL; positive) or PBS (negative) and incubated for 1 h at 37°C. The plate-A was washed three times with PBST, then blocked with 3% gelatin for 1 h at 37°C. After plate-A was washed again, 100 μL solution from plate-B was added to plate-A and incubated for 1 h at 37°C. After further washing, 100 μL of HRP-IgG (diluted 1:10,000 in PBS) was added and incubated for 1 h at 37°C. The subsequent procedures were identical to IgG binding ability test. The percent inhibition (PI) value was expressed as follows:
A0, A1, and A2 represented the OD of blank control, the OD of antisera with peptide (positive), and the OD of antisera with PBS (negative), respectively.
Specificity of pAb-tαS1-CN
The specificity of pAb-tαS1-CN was evaluated by testing the cross-reactivity (CR) with various nontarget food allergens (α-lactalbumin, β-lactoglobulin, egg proteins, wheat proteins, oat proteins, and soybean proteins) using competitive inhibition ELISA with the ELISA procedures as described above. The 50% inhibition concentration (IC50%) was used to determine the CR as follows: CR% = IC50 (αS1-CN)/IC50 (inhibitor) × 100%.
Validation of the ability of pAb-tαS1-CN to evaluate the potential allergenicity of dairy foods
The ability of pAb-tαS1-CN to evaluate the potential allergenicity of milk protein in food was validated by sera pool from seventeen CMA patients using indirect ELISA. The samples were pretreated according to our previous study (He, Li, Wu et al., Citation2018). In short, fat was removed from the UHT milk, yogurts, and beverage by centrifugation at 12,000 × g for 10 min at 4°C. Cake and cookie were ground into a fine powder, and 1 g of powder was dissolved in 20 mL Tris-HCl (20 mM, pH 8.0, containing 2% Tween-20). The mixture was agitated overnight at 4°C, followed by centrifugation at 12,000 × g for 10 min at 4°C, after which the supernatant was collected. Then the samples were diluted with coating buffer and coated in a microtiter plate. After blocking and washing, 100 μL of pAb-tαS1-CN (diluted 1:20,000 in PBS) or sera pool (diluted 1:1,600 in blocking buffer) was added, and the subsequent procedures were identical to the IgG or IgE binding ability test described above, respectively.
Statistical analysis
Data were analyzed using the software OriginPro 8.5.0 (OriginLab Inc., Northampton, MA, USA), tested using descriptive statistical analysis, and results were reported as the average and standard deviation (SD). Statistical significance was carried out using SPSS 17.0 (SPSS Inc., Chicago, IL, USA), and differences were performed by the Tukey’s pairwise comparisons of ANOVA; statistically significant was considered at ∗p < 0.05 and ∗∗p < 0.01.
Results and discussion
Design and construction of tαS1-CN expression strain
DNAStar software and network server-SOMPA were applied to optimize the portfolio epitope combination of tαS1-CN. The optimal portfolio epitope combination of tαS1-CN was as follows: T-GGE-B7-GG-B1-GG-B6-GG-B2-GG-B9-GG-B4-GG-B8-GG-B5-GG-B3. The amino acid and gene sequences of the designed tαS1-CN are shown in Figure S2. The DNAStar software predicted results showed that B1–B9 in the designed tαS1-CN had good hydrophilicity, antigenicity, and surface accessibility (Figure S3) and contained β-turn or random coil regions, as predicted by network server-SOMPA (Figure S4). These results indicated that the designed tαS1-CN was reasonable. Then, the tαS1-CN expression strain was constructed by Chinapeptides (Shanghai, China). The sequencing results showed that the recombinant plasmid pET28a(+)-tαS1-CN contained the full gene of tαS1-CN in expression strain E. coli BL21 (DE3) pLysS (Figure S5, located between 162 and 683 bp), indicating that the expression strain had been successfully constructed.
Expression of recombinant tαS1-CN
First, the expression of recombinant tαS1-CN was investigated at 37°C by induction with 1 mM IPTG. A distinct band with molecular weight of approximately 30 kDa was observed after induction with IPTG, and the content of recombinant protein increased with increasing IPTG induction time ((A)). The band corresponding to recombinant tαS1-CN appeared at a greater apparent molecular mass than its natural molecular weight (containing 174 AAs and a 6 × His-tag, about 20.09 kDa). This phenomenon was similar to αS1-CN, which had a larger hydrodynamic size and led to it having lower mobility on SDS–PAGE gels than anticipated, considering molecular mass (Treweek et al., Citation2011). In addition, other reports also showed that the observed molecular weight of His-tag fusion protein in SDS-PAGE was larger than their predicted size (Bierig et al., Citation2020; Dickey et al., Citation2017). Therefore, these results suggest that the recombinant tαS1-CN was successfully expressed. Subsequently, different IPTG concentrations, incubation temperatures, and incubation times were chosen to optimize the yield of recombinant tαS1-CN. The content of recombinant protein did not noticeably change with increasing IPTG concentration ((B)). Thus, 0.1 mM IPTG was selected as the optimal concentration for induction. The recombinant protein content increased with increasing induction temperature (20–37°C, (C)) and incubation time (2–10 h, (D)). Therefore, the optimum condition was induced with 0.1 mM IPTG at 37°C for 10 h.
Figure 1. SDS-PAGE analysis of the recombinant tαS1-CN under different induction conditions. (A) With or without IPTG. M: markers; lanes 1, 3, 5, 7, and 9: incubation without IPTG for 0, 2, 3, 4, and 5 h, respectively; lanes 2, 4, 6, and 8: induction with IPTG for 2, 3, 4, and 5 h, respectively. (B) Induction with different IPTG concentrations for 4 h at 37°C. M: markers; lanes 1–6: induction with 0.1, 0.3 0.5, 0.7, 1, and 1.3 mM IPTG, respectively. (C) Induction with 0.1 mM IPTG for 4 h at different temperatures. M: markers; lanes 1–6: induction at 37, 34, 30, 27, 23, and 20°C, respectively. (D) Induction with 0.1 mM IPTG at 37°C for different times. M: markers; lanes 1–6: induction for 2, 3, 4, 5, 6, 8, and 10 h, respectively. The recombinant tαS1-CN band is indicated by arrow.

Purification of recombinant tαS1-CN
Before being purified by Histrap HP, the E. coli cells were lysed by sonication, and the proteins were extracted using PBS containing urea. As shown in (A) (lanes 1–2), only a trace amount of recombinant tαS1-CN was found in the supernatant after sonication. Subsequently, the precipitate was further extracted with PBS-1 (20 mM PBS, containing 0.5 M NaCl, 2 M urea, and 0.1% Triton-100), and the recombinant tαS1-CN was mostly in the precipitate ((A), lanes 3–6). These results indicate that the recombinant tαS1-CN was expressed as inclusion body. This might be because: (1) although high induction temperature (37°C) can improve the expression level, this always results in the formation of inclusion body (Singh, Upadhyay et al., Citation2020); (2) the recombinant tαS1-CN contains about 70% AAs of native αS1-CN, resulting in the recombinant tαS1-CN similar to native αS1-CN with poor water solubility (Villa et al., Citation2018). Therefore, the recombinant protein in the precipitate was further extracted with 8 M urea in PBS ((A), lane 7), followed by purification by Histrap HP affinity chromatography column. As shown in (B), the nonspecific proteins were eluted by binding buffer (lane 1), and there was no protein after washing with 20 column volumes of binding buffer (lane 2). Then, the tagged protein bound to the Histrap HP affinity column was eluted with 5 column volumes of elution buffer ((B), lane 3), and 94.43% high purity recombinant tαS1-CN was obtained, which was imaged by ImageJ software.
Figure 2. SDS-PAGE analysis of recombinant tαS1-CN purified with Histrap HP. (A) The proteins extracted from cell lysates with different buffers. M: markers; lane 1: the cells before sonication; lane 2: the supernatant after sonication; lanes 3/4 and 5/6: the supernatant/precipitate after treatment with PBS-1 twice, respectively; lane 7: the supernatant after treatment with PBS-2. (B) Recombinant tαS1-CN purified with Histrap HP. M: markers; lane 1: flow-through protein of the column; lanes 2 and 3: eluted with 20 and 500 mM imidazole, respectively. The recombinant protein band is indicated by arrow.

IgG and IgE binding abilities of recombinant tαS1-CN
The titres of sera from two rabbits were determined by indirect ELISA using recombinant tαS1-CN (1 μg/mL) as coating antigen. After inoculation with purified recombinant tαS1-CN five times, the titres of sera from rabbits A and B reached 1:160,000 and 1:80,000 ((A)), respectively. Thus, the sera from rabbit A were chosen for the subsequent research.
Figure 3. IgG and IgE-binding abilities of recombinant tαS1-CN. (A) The titres of antisera against recombinant tαS1-CN. (B) Cross-reactivity between recombinant tαS1-CN and native αS1-CN was defined by indirect ELISA by using pAb-tαS1-CN or pAb-αS1-CN as primary antibody. (C) IgE-binding abilities of recombinant tαS1-CN and native αS1-CN were defined by indirect ELISA with sera from CMA patients. P1 to P4 represent the sera from four CMA patients. Data are represented as mean ± SD (n = 3).

Whether the antigenicity and allergenicity of IgE epitopes of native αS1-CN are preserved in recombinant tαS1-CN directly relates to the preparation of IgE epitopes-specific antibody. In order to clarify the antigenicity and allergenicity of the recombinant tαS1-CN, the IgG and IgE binding abilities of recombinant tαS1-CN were analyzed, and the native αS1-CN was used as positive control. As shown in (B), the IgG binding ability of pAb-αS1-CN to the native αS1-CN was about 1.8-folds higher than its binding ability to the recombinant tαS1-CN, which may be due to the fact that the native αS1-CN contains more epitopes than recombinant tαS1-CN (Chatchatee et al., Citation2001; Jarvinen et al., Citation2002; Lisson et al., Citation2014). This result is similar to our previous report (He et al., Citation2016). In contrast, the IgG binding ability of pAb-tαS1-CN to recombinant tαS1-CN was almost the same as its binding ability to native αS1-CN. These results strongly indicate that the antigenicity of the IgE epitopes of native αS1-CN is preserved well in the recombinant tαS1-CN, and the pAb-tαS1-CN can efficiently recognize the IgE epitopes on native αS1-CN.
To determine whether the allergenicity of IgE epitopes was preserved in the recombinant tαS1-CN, the IgE binding to recombinant tαS1-CN was estimated by indirect ELISA with sera from CMA patients. As shown in (C), both the recombinant tαS1-CN and native αS1-CN were recognized by all four CMA patients’ sera. Due to the native αS1-CN containing more IgE epitopes than recombinant tαS1-CN (Chatchatee et al., Citation2001; Jarvinen et al., Citation2002; Lisson et al., Citation2014), all four sera responded more strongly to native αS1-CN than to recombinant tαS1-CN ((C)). Moreover, individual differences caused the four patients’ sera to have different responsive intensities to native αS1-CN or to recombinant tαS1-CN (Azdad et al., Citation2018). Nevertheless, the ratios of ODαS1-CN/ODtαS1-CN were relatively stable (1.56 ± 0.21) ((C), red and broken black lines). These results indicate that the allergenicity of IgE epitopes of native αS1-CN is preserved well in the recombinant tαS1-CN.
Characteristics of pAb-tαS1-CN binding to epitopes
In order to further confirm that the prepared pAb-tαS1-CN could recognize the IgE epitopes of native αS1-CN, we analyzed the binding ability of pAb-tαS1-CN to the nine IgE epitopes and blocking peptides by competitive inhibition ELISA (). The results show that the pAb-tαS1-CN recognizes all the IgE epitopes and blocking peptides, the IgE epitope and blocking peptide have a similar binding ability to pAb-tαS1-CN, and the inhibition increases with the increasing peptide concentration. The inhibition of B4 was significantly higher than the others, which might be because the synthetic score of hydrophilicity, antigenicity, and surface accessibility of B4 was relatively higher than the others (Figure S2, AA111–130), which caused the rabbits to produce higher levels of antibodies in response to B4. The other eight epitopes had a similar inhibition. In addition, the mixture of B1–B9 and b1–b9 also had a similar binding ability to pAb-tαS1-CN. Also, the inhibition ratio reached 65.75% when the concentration of the mixture of B1–B9 was 27 μg/mL, which was similar to the total inhibition ratio (66.98%) of each IgE epitope at 3 μg/mL, and the inhibition ratio (71.24%) of the mixture of b1–b9 at 27 μg/mL was higher than the total inhibition ratio (54.81%) of each blocking epitope at 3 μg/mL (). Therefore, these results suggest that the prepared pAb-tαS1-CN can recognize all nine IgE epitopes and blocking peptides, and the peptides do not inhibit each other when binding to pAb-tαS1-CN, further indicating that pAb-tαS1-CN can precisely detect the content of αS1-CN IgE epitopes in foods.
Figure 4. Binding characteristics of pAb-tαS1-CN to IgE epitopes and blocking peptides at different concentrations (1, 3, 9, and 27 μg/mL) were defined by indirect competition ELISA. B1 to B9 represent nine IgE epitopes, and b1 to b9 represent nine blocking peptides. Data are represented as mean ± SD (n = 3).

The specificity of pAb-tαS1-CN
The specificity of pAb-tαS1-CN was evaluated by competitive inhibition ELISA and defined as the CR with other allergens. The results of IC50 and CR are shown in . The IC50 of αS1-CN was 6.83 µg/mL, and no CR was found with α-lactalbumin, β-lactoglobulin, egg proteins, wheat proteins, and oat proteins (). However, there was slight CR with soybean proteins. This finding is in accordance with the results from MALDI-TOF MS, mice model, and sera (from CMA patients) tests, which demonstrated that CR exists between αS1-CN and soybean proteins (such as Gly m 5 and P34) (Candreva et al., Citation2015, Citation2016, Citation2017). In the current study, the inhibition rate was 7.15% at a soybean proteins concentration of 128 µg/mL and this rate was much lower (p < 0.01) than 12.71% when the αS1-CN concentration was 0.125 µg/mL (), suggesting that the CR with soybean proteins was lower than 0.098%. These results indicate that the pAb-tαS1-CN is highly specific.
Evaluating potential allergenicity of milk in foods
The ability of pAb-tαS1-CN to evaluate the potential allergenicity of milk in foods was validated by sera from CMA patients. Seven samples were detected by both pAb-tαS1-CN and sera pool from CMA patients (n = 17, ). UHT milk and PBS were used as positive and blank control, respectively. Noticeably, the immunoreactivity of these seven samples tested by pAb-tαS1-CN and CMA patients’ sera had a similar varying tendency. The samples of UHT milk, yogurts, beverage, and cake contained cow's milk (); hence they could be detected by both pAb-tαS1-CN and CMA patients’ sera (). Overall, the immunoreactivity intensity decreased with decreasing concentration of milk protein in samples, while there was a contrary phenomenon in yogurts (, ), which might be due to the different fermenting degrees that resulted in the IgE epitopes being damaged to different degrees (Kordesedehi et al., Citation2018; Wroblewska et al., Citation2020). In addition, raw milk was the only source of protein in UHT milk, yogurt-1 and yogurt-2 (); thus, the ratios of OD(yogurt-1 and yogurt-2) to ODUHT milk tested by pAb-tαS1-CN had no significant difference from those tested by CMA patients’ sera (). However, the samples of yogurt-3, beverage, and cake contained milk powder and whey powder (). Therefore, the whey protein could be recognized by CMA patients’ sera but could not react with pAb-tαS1-CN (, α-lactalbumin and β-lactoglobulin), resulting in the ratios of OD(yogurt-3, beverage, and cake) to ODUHT milk tested by CMA patients’ sera being significantly higher than those tested by pAb-tαS1-CN (). In addition, the cookie lacked milk protein (); thus, it could not be recognized by both pAb-tαS1-CN and CMA patients’ sera (). Therefore, these results strongly suggest that the pAb-tαS1-CN can predict the potential allergenicity of milk in foods without extra added whey protein.
Figure 6. The ability of pAb-tαS1-CN to evaluate the potential allergenicity of milk in foods was validated by indirect ELISA with sera pool from CMA patients (n = 17). The samples of UHT milk, yogurts and beverage were diluted 1 × 105 times in coating buffer; the samples of cake and cookie were diluted 1 × 103 times and 1 × 102 times in coating buffer, respectively. Data are represented as mean ± SD (n = 3). Significant at ∗p < 0.05 and ∗∗p < 0.01. ODS, ODM, and ODB represent the OD of samples (yogurts, beverage, cake, and cookie), the OD of UHT milk, and the OD of blank (PBS), respectively. ND: Not detected.

Table 3. Information of protein source and protein concentration in food samples.
The advantages of pAb-tαS1-CN for predicting potential allergenicity of milk in foods
Currently, a double-blind placebo-controlled food challenge is the gold standard to evaluate the allergenicity of food allergens, but this test is rarely performed routinely in practice because of various practical issues, such as safety risks and lack of a standardized matrix preparation (Alexiou et al., Citation2022). Additionally, IgE-binding capacity, animal sensitization model, and basophils/mast cells degranulation tests are the principal methods to assess the allergenicity of food allergens. Among these, the use of an animal model is preferable to evaluate the allergenicity of food allergens, but this involves a heavy workload, a long experiment period, and high costs (Yang et al., Citation2019). Moreover, it needs a large amount of allergen to stimulate the animal (Van Esch et al., Citation2020). On the other hand, the IgE-binding capacity test is the main method used to rapidly evaluate the potential allergenicity of food allergens, but this method is massively dependent on sera from food allergy patients (Chakrapani et al., Citation2022; Martin-Pedraza et al., Citation2021). Furthermore, patients’ sera are very limited, and individual differences exist. Therefore, the practical application of this approach is limited. Finally, the basophils/mast cells degranulation test not only needs patients’ sera to sensitize the cells but is also expensive, time-consuming, and dependent on highly technical requirements (Chang et al., Citation2022). To overcome the limitations of using patients’ sera, sera from animals (such as mice and rabbits) are widely used to estimate the security of food allergens. However, the traditional antisera from animals cannot specifically recognize IgE-binding epitopes of allergens, resulting in the potential allergenicity not being predicted (Vencia et al., Citation2019).
The results of the current study facilitate a new method of assessing the allergenicity of food allergen which overcomes the limitations of the traditional methods. In the current study, the prepared pAb-tαS1-CN could specifically recognize nine IgE epitopes of native αS1-CN (). Our findings primarily validate that pAb-tαS1-CN can predict the potential allergenicity of milk in foods without extra added whey protein (). Therefore, the prepared pAb-tαS1-CN can rapidly predict milk-allergenic behaviour in processed foods and overcome the shortcomings of the traditional methods.
Conclusions
In this study, we designed, expressed and purified a tandem containing nine IgE epitopes of native αS1-CN and developed a high-specificity antibody for the first time. Indirect ELISA indicated that the recombinant tαS1-CN preserved the antigenicity and allergenicity of the IgE epitopes. The pAb-tαS1-CN could recognize nine IgE epitopes of native αS1-CN and showed excellent specificity. The potential allergenicity of milk in seven samples was evaluated by pAb-tαS1-CN, and the results correlated with those tested by sera pool from CMA patients. These results suggest that our prepared pAb-tαS1-CN can be used as a novel tool instead of sera from CMA patients for rapid preliminary prediction of milk-allergenic behaviour in foods, with high sensitivity and strong specificity. Moreover, this kind of IgE epitope-specific antibody can now easily be extended to evaluate the allergenicity of other food allergens and can overcome the limitations of conventional methods.
cfai_a_2222932_sm0612.docx
Download MS Word (3.4 MB)Disclosure statement
No potential conflict of interest was reported by the author(s).
Additional information
Funding
References
- Alexiou, A., Höfer, V., Dölle-Bierke, S., Grünhagen, J., Zuberbier, T., & Worm, M. (2022). Elicitors and phenotypes of adult patients with proven IgE-mediated food allergy and non-immune-mediated food hypersensitivity to food additives. Clinical and Experimental Allergy, 52(11), 1302–1310. https://doi.org/10.1111/cea.14203
- Azdad, O., Mejrhit, N., & Aarab, L. (2018). Reduction of the allergenicity of cow's milk alpha-lactalbumin under heat-treatment and enzymatic hydrolysis in Moroccan population. European Annals of Allergy and Clinical Immunology, 50(4), 177–183. https://doi.org/10.23822/EurAnnACI.1764-1489.60
- Aziz, S., Almajhdi, F. N., Waqas, M., Ullah, I., Salim, M. A., Khan, N. A., & Ali, A. (2022). Contriving multi-epitope vaccine ensemble for monkeypox disease using an immunoinformatics approach. Frontiers in Immunology, 13, 1004804. https://doi.org/10.3389/fimmu.2022.1004804
- Bierig, T., Collu, G., Blanc, A., Poghosyan, E., & Benoit, R. M. (2020). Design, expression, purification, and characterization of a YFP-tagged 2019-nCoV spike receptor-binding domain construct. Frontiers in Bioengineering and Biotechnology, 8, 618615. https://doi.org/10.3389/fbioe.2020.618615
- Broersen, K. (2020). Milk processing affects structure, bioavailability and immunogenicity of β-lactoglobulin. Foods, 9(7), 874. https://doi.org/10.3390/foods9070874
- Cai, Q., Zhang, W. J., Zhu, Q. Q., & Chen, Q. (2016). Influence of heat treatment on the structure and core IgE-binding epitopes of rAra h 2.02. Food Chemistry, 202, 404–408. https://doi.org/10.1016/j.foodchem.2016.02.004
- Candreva, A. M., Ferrer-Navarro, M., Bronsoms, S., Quiroga, A., Curciarello, R., Cauerhff, A., Petruccelli, S., Docena, G. H., & Trejo, S. A. (2017). Identification of cross-reactive B-cell epitopes between Bos d 9.0101(Bos Taurus) and Gly m 5.0101 (Glycine max) by epitope mapping MALDI-TOF MS. Proteomics, 17(15–16), 1700069. https://doi.org/10.1002/pmic.201700069
- Candreva, A. M., Smaldini, P. L., Curciarello, R., Cauerhff, A., Fossati, C. A., Docena, G. H., & Petruccelli, S. (2015). Cross-reactivity between the soybean protein p34 and bovine caseins. Allergy Asthma & Immunology Research, 7(1), 60–68. https://doi.org/10.4168/aair.2015.7.1.60
- Candreva, A. M., Smaldini, P. L., Curciarello, R., Fossati, C. A., Docena, G. H., & Petruccelli, S. (2016). The major soybean allergen Gly m Bd 28 K induces hypersensitivity reactions in mice sensitized to cow's milk proteins. Journal of Agricultural and Food Chemistry, 64(7), 1590–1599. https://doi.org/10.1021/acs.jafc.5b05623
- Chakrapani, N., Fischer, J., Swiontek, K., Codreanu-Morel, F., Hannachi, F., Morisset, M., Mugemana, C., Bulaev, D., Blank, S., Bindslev-Jensen, C., Biedermann, T., Ollert, M., & Hilger, C. (2022). α-Gal present on both glycolipids and glycoproteins contributes to immune response in meat-allergic patients. Journal of Allergy and Clinical Immunology, 150(2), 396–405.e11. https://doi.org/10.1016/j.jaci.2022.02.030
- Chang, X. J., Zhou, X. Y., Tang, Y., Zhang, Y., Yuan, J. L., Li, X., Yang, A. S., Tong, P., Wu, Z. H., & Chen, H. B. (2022). Effect of processing on the structure and allergenicity of peanut allergen Ara h 2 roasted in a matrix. Journal of Agricultural and Food Chemistry, 70(2), 626–633. https://doi.org/10.1021/acs.jafc.1c06828
- Chatchatee, P., Jarvinen, K. M., Bardina, L., Beyer, K., & Sampson, H. A. (2001). Identification of IgE- and IgG-binding epitopes on alpha(s1)-casein: Differences in patients with persistent and transient cow's milk allergy. Journal of Allergy and Clinical Immunology, 107(2), 379–383. https://doi.org/10.1067/mai.2001.112372
- Dickey, A., Wang, N., Cooper, E., Tull, L., Breedlove, D., Mason, H., Liu, D., & Wang, K. Y. J. (2017). Transient expression of lumbrokinase (PI239) in tobacco (Nicotiana tabacum) using a geminivirus-based single replicon system dissolves fibrin and blood clots. Evidence-based Complementary and Alternative Medicine, 2017, 6093017. https://doi.org/10.1155/2017/6093017
- Fang, M. L., Li, J. L., Wang, H., Yang, M., Zhang, Y. S., Zhou, L., Wei, H. F., Yang, G., Yu, Y., Wei, X. F., Yu, Y. L., Wang, L. Y., & Wan, M. (2012). Correlation between efficacy and structure of recombinant epitope vaccines against bovine type O foot and mouth disease virus. Biotechnology Letters, 34(5), 839–847. https://doi.org/10.1007/s10529-012-0856-0
- Flom, J. D., & Sicherer, S. H. (2019). Epidemiology of cow's milk allergy. Nutrients, 11(5), 1051. https://doi.org/10.3390/nu11051051
- Giannetti, A., Vespasiani, G. T., Ricci, G., Miniaci, A., di Palmo, E., & Pession, A. (2021). Cow's milk protein allergy as a model of food allergies. Nutrients, 13(5), 1525. https://doi.org/10.3390/nu13051525
- He, S. F., Li, X., Gao, J. Y., Tong, P., & Chen, H. B. (2017). Development of sandwich ELISA for testing bovine beta-lactoglobulin allergenic residues by specific polyclonal antibody against human IgE binding epitopes. Food Chemistry, 227, 33–40. https://doi.org/10.1016/j.foodchem.2017.01.060
- He, S. F., Li, X., Gao, J. Y., Tong, P., & Chen, H. B. (2018a). Development of a H2O2-sensitive quantum dots-based fluorescent sandwich ELISA for sensitive detection of bovine beta-lactoglobulin by monoclonal antibody. Journal of the Science of Food and Agriculture, 98(2), 519–526. https://doi.org/10.1002/jsfa.8489
- He, S. F., Li, X., Gao, J. Y., Tong, P., Lu, J., & Chen, H. B. (2016). Preparation, immunological characterization and polyclonal antibody development for recombinant epitope tandem derived from bovine β-lactoglobulin. Food and Agricultural Immunology, 27(6), 806–819. https://doi.org/10.1080/09540105.2016.1183596
- He, S. F., Li, X., Wu, Y., Wu, S. D., Wu, Z. H., Yang, A. S., Tong, P., Yuan, J. L., Gao, J. Y., & Chen, H. B. (2018). Highly sensitive detection of bovine beta-lactoglobulin with wide linear dynamic range based on platinum nanoparticles probe. Journal of Agricultural and Food Chemistry, 66(44), 11830–11838. https://doi.org/10.1021/acs.jafc.8b04086
- He, S. F., Long, C. Y., Wang, J., Xiong, M., Zhao, J. J., Yan, Y., Dong, Y. P., Li, X., & Chen, H. B. (2022). Purification, identification and polyclonal antibody development for cow’s milk major allergen αS1-casein. Science and Technology of Food Industry (in Chinese with English Abstract), 43(15), 106–114. https://doi.org/10.13386/j.issn1002-0306.2021110034.
- Huang, M. J., Yang, F., Wu, Y., Meng, X. Y., Shi, L. B., Chen, H. B., & Li, X. (2023). Identification of peptides sequence and conformation contributed to potential allergenicity of main allergens in yogurts. Frontiers in Nutrition, 9, 1038466. https://doi.org/10.3389/fnut.2022.1038466
- Jarvinen, K. M., Beyer, K., Vila, L., Chatchatee, P., Busse, P. J., & Sampson, H. A. (2002). B-cell epitopes as a screening instrument for persistent cow's milk allergy. Journal of Allergy and Clinical Immunology, 110(2), 293–297. https://doi.org/10.1067/mai.2002.126080
- Knipping, K., Simons, P. J., Buelens-Sleumer, L. S., Cox, L., den Hartog, M., de Jong, N., Teshima, R., Garssen, J., Boon, L., & Knippels, L. M. J. (2014). Development of β-lactoglobulin-specific chimeric human IgEκ monoclonal antibodies for in vitro safety assessment of whey hydrolysates. PLoS One, 9(8), e106025. https://doi.org/10.1371/journal.pone.0106025
- Kordesedehi, R., Taheri-Kafrani, A., Rabbani-Khorasgani, M., Kazemi, R., Mutangadura, D., & Haertle, T. (2018). Modification of IgE binding to αS1-casein by proteolytic activity of enterococcus faecium isolated from Iranian camel milk samples. Journal of Biotechnology, 276–277, 10–14. https://doi.org/10.1016/j.jbiotec.2018.04.005
- Lisson, M., Novak, N., & Erhardt, G. (2014). Immunoglobulin E epitope mapping by microarray immunoassay reveals differences in immune response to genetic variants of caseins from different ruminant species. Journal of Dairy Science, 97(4), 1939–1954. https://doi.org/10.3168/jds.2013-7355
- Mariager, B., Sølve, M., Eriksen, H., & Brogren, C. H. (1994). Bovine β-lactoglobulin in hypoallergenic and ordinary infant formulars measured by an indirect competitive ELISA using monoclonal and polyclonal antibodies. Food and Agricultural Immunology, 6(1), 73–78. https://doi.org/10.1080/09540109409354815
- Martin-Pedraza, L., Mayorga, C., Gomez, F., Bueno-Díaz, C., Blanca-Lopez, N., González, M., Martínez-Blanco, M., Cuesta-Herranz, J., Molina, E., Villalba, M., & Benedé, S. (2021). IgE-Reactivity pattern of tomato seed and peel nonspecific lipid-transfer proteins after in vitro gastrointestinal digestion. Journal of Agricultural and Food Chemistry, 69(11), 3511–3518. https://doi.org/10.1021/acs.jafc.0c06949
- Muller-Renaud, S., Dupont, D., & Dulieu, P. (2005). Development of a biosensor immunoassay for the quantification of aS1-casein in milk. Journal of Dairy Research, 72(1), 57–64. https://doi.org/10.1017/s0022029904000664
- Orcajo, J., Lavilla, M., & Martínez-de-Marañón, I. (2019). Specific and sensitive ELISA for measurement of IgE-binding variations of milk allergen β-lactoglobulin in processed foods. Analytica Chimica Acta, 1052, 163–169. https://doi.org/10.1016/j.aca.2018.11.048
- Plebani, A., Restani, P., Naselli, A., Galli, C. L., Meini, A., Cavagni, G., Ugazio, A. G., & Poiesi, C. (1997). Monoclonal and polyclonal antibodies against casein components of cow milk for evaluation of residual antigenic activity in ‘hypoallergenic’ infant formulas. Clinical and Exjyerimcnial Allergy, 27(8), 949–956. PMID: 9291294. https://doi.org/10.1111/j.1365-2222.1997.tb01238.x
- Ruiter, B., Tregoat, V., M'rabet, L., Garssen, J., Bruijnzeel-Koomen, C. A. F. M., Knol, E. F., & Hoffen, E. (2006). Characterization of T cell epitopes in αS1-casein in cow's milk allergic, atopic and non-atopic children. Clinical and Experimental Allergy, 36(3), 303–310. https://doi.org/10.1111/j.1365-2222.2006.02436.x
- Sicherer, S. H., & Sampson, H. A. (2018). Food allergy: A review and update on epidemiology, pathogenesis, diagnosis, prevention, and management. Journal of Allergy and Clinical Immunology, 141(1), 41–58. https://doi.org/10.1016/j.jaci.2017.11.003
- Singh, A., Upadhyay, V., Singh, A., & Panda, A. K. (2020). Structure-function relationship of inclusion bodies of a multimeric protein. Frontiers in Microbiology, 11, 876. https://doi.org/10.3389/fmicb.2020.00876
- Singh, A. P., Prabhu, S. N., Nagaleekar, V. K., Dangi, S. K., Prakash, C., & Singh, V. P. (2020a). Immunogenicity assessment of clostridium perfringens type D epsilon toxin epitope-based chimeric construct in mice and rabbit. 3 Biotech, 10(9), 406. https://doi.org/10.1007/s13205-020-02400-4
- Tasaniyananda, N., Tungtrongchitr, A., Seesuay, W., Sakolvaree, Y., Aiumurai, P., Indrawattana, N., Chaicumpa, W., & Sookrung, N. (2018). Quantification of Fel d 1 in house dust samples of cat allergic patients by using monoclonal antibody specific to a novel IgE-binding epitope. Asian Pacific Journal of Allergy and Immunology, 36(1), 8–15. https://doi.org/10.12932/AP0876
- Treweek, T. M., Thorn, D. C., Price, W. E., Price, W. E., & Carver, J. A. (2011). The chaperone action of bovine milk αS1- and αS2-caseins and their associated form αS-casein. Archives of Biochemistry and Biophysics, 510(1), 42–52. https://doi.org/10.1016/j.abb.2011.03.012
- Van Esch, B. C. A. M., Van Bilsen, J. H. M., Gros-Van Hest, M., Kleinjans, L., Belzer, C., Jeurink, P. V., Garssen, J., Smit, J. J., Pieters, R. H. H., & Knippels, L. M. J. (2020). A multi-center assessment to compare residual allergenicity of partial hydrolyzed whey proteins in a murine model for cow's milk allergy - comparison to the single parameter Guinea pig model. Toxicology Letters, 333, 312–321. https://doi.org/10.1016/j.toxlet.2020.05.020
- Vencia, W., Minale, P., Migone, L., Lazzara, F., Vito, G., Ferrari, A., & Razzuoli, E. (2019). Effects of thermal treatment on walnut detection and allergenicity. Journal of the Science of Food and Agriculture, 99(5), 2636–2640. https://doi.org/10.1002/jsfa.9428
- Villa, C., Costa, J., Oliveira, M. B. P. P., & Mafra, I. (2018). Bovine milk allergens: A comprehensive review. Comprehensive Reviews in Food Science and Food Safety, 17(1), 137–164. https://doi.org/10.1111/1541-4337.12318
- Wroblewska, B., Kaliszewska-Suchodola, A., Fuc, E., Markiewicz, L. H., Ogrodowczyk, A. M., Złotkowska, D., & Wasilewska, E. (2020). Effect of low-immunogenic yogurt drinks and probiotic bacteria on immunoreactivity of cow's milk proteins and tolerance induction-In vitro and in vivo studies. Nutrients, 12(11), 3390. https://doi.org/10.3390/nu12113390
- Xu, L., Gong, Y. S., Gern, J. E., & Lucey, J. A. (2020). Influence of whey protein hydrolysis in combination with dextran glycation on immunoglobulin E binding capacity with blood sera obtained from patients with a cow milk protein allergy. Journal of Dairy Science, 103(2), 1141–1150. https://doi.org/10.3168/jds.2019-17187
- Yang, X., Liu, Y., Guo, X. D., Bai, Q., Zhu, X. F., Ren, H., Chen, Q. W., Yue, T. L., & Long, F. Y. (2019). Antiallergic activity of Lactobacillus plantarum against peanut allergy in a Balb/c mouse model. Food and Agricultural Immunology, 30(1), 762–772. https://doi.org/10.1080/09540105.2019.1631261
- Zhang, Z., Ma, R. D., Xu, Y. P., Chi, L., Li, Y., Mu, G. Q., & Zhu, X. M. (2022). Investigation of the structure and allergic potential of whey protein by both heating sterilization and simulation with molecular dynamics. Foods, 11(24), 4050. https://doi.org/10.3390/foods11244050