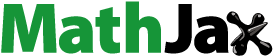
ABSTRACT
This work deals with a physically based creep model of the martensitic 9% Cr-steel P91. In particular, we quantitatively study the effect of precipitates located in the subgrain interior on the creep curve and the lifetime of the material. Our creep model is capable of simulating the microstructural evolution parallel to creep curves and has been further developed towards predicting time-to-rupture (TTR) diagrams. We demonstrate how the number density, size and shape of the precipitates in the subgrain interior affect the creep curve and thus the lifetime of the material. Three according parameter studies on the material P91 compare microstructures with/without precipitates and/or assuming specific shapes by introducing aspect ratios. These simulations can be used as a basis for further development of materials with regard to the precipitation phases.
Introduction
Modern thermal power plants with high efficiency contain components exposed to elevated temperatures and stresses. These components deform plastically by a slow rate due to the phenomenon of creep, which is limiting their service time significantly. In order to increase the service time, one has to understand the underlying physical processes causing these deformations. In our case, the material in question is P91, which belongs to the group of martensitic 9–12% Cr steels.
Martensitic 9–12% Cr-steels are state of the art materials for power plants due to their high creep strength and oxidation resistance [Citation1]. In order to increase the lifetime of the material (lower effective costs per operation year) and/or the service temperature (higher efficiency of the plant, lower CO2 output), one has to further improve the creep strength [Citation1,Citation2]. Unfortunately, experimental approaches to this task are extremely costly and time-consuming, since the critical degradations take place after 10+ operating years. Modelling approaches can assist in better understanding the results and shorten the experimental times. For this reason, we focus on improving modelling approaches.
Phenomenological models can mimic the creep deformation of materials (see e.g. the detailed summary of those model type by Holdsworth et al. [Citation3]). This type of models describes the creep deformation by a set of analytical functions with fit parameters and can be applied quickly. Unfortunately, this type of model has two disadvantages:
(i) the fit parameters can vary with applied temperature, stress, the material type and even the material heat and (ii) the model will not give insight into the very reasons for a good/bad creep behaviour of a specific material and thus cannot guide towards improving an existing material.
In contrast, physical models can provide this information; however, they are generally more challenging to be set up. These models consider the underlying creep mechanisms including the microstructure, interactions within the microstructure, the microstructural evolution during the creep process and, finally, the manifestation of these processes in terms of creep deformation (rate). These models also provide the opportunity to rate different starting microstructures and their effect on creep life, which is presumably the reason for different creep behaviour of various material badges of the same material type (apart from unintended variations of experimental parameters).
This work deals with an extension of such a physically based creep model, which has previously shown good results for the martensitic 9% Cr-steel P91 [Citation4]. The extension of the model includes a physically more accurate representation of the effect of precipitates in the subgrain interior that impede mobile dislocations in their gliding processes. We show the effect of the improvements in the model, the effect of the precipitates, as well as their influence on the lifetime of the material, and suggest potential further improvements for future models.
Creep model of the IMK research group
In order to account for the microstructural changes of the material, a microstructurally based physical dislocation creep model has been developed by our working group [Citation4]. Since the creep strain rate can be linked to the current mobile dislocation density and -velocity, creep curves and TTR diagrams can be deducted from the evolution of the microstructure and the specific interactions therein.
The core of our model has been developed by Riedlsperger [Citation4], based on pre-works of Ghoniem [Citation5], Yadav [Citation6] and Basirat [Citation7]. Starting point of a simulation is the initial microstructure and precipitation data (e.g. from the software MatCalc [Citation8]). The model considers various aspects of the microstructural evolution and interactions of mobile dislocations (density ρm), static dislocations (density ρs), boundary dislocations (density ρb), and subgrains (radius Rsbg). The creep strain rate is calculated based on the Orowan equation. Orowan’s law [Citation9] has been modified with respect to the velocity of mobile dislocations by now using an effective velocity veff instead of the pure sliding velocity vg. Thus, the dislocation climb across precipitates in the subgrain interior can also be taken into account.
The potential microstructural interactions within a subgrain are depicted in . Here, X denotes an annihilation of dislocations and arrows denote transformations.
Figure 1. Microstructural interactions within a subgrain [Citation10].
![Figure 1. Microstructural interactions within a subgrain [Citation10].](/cms/asset/ae0d17ed-7b66-49c2-98aa-1ccceaebe345/ymht_a_2310389_f0001_c.jpg)
In the creep model, the following interaction, generation, annihilation, and/or transformation processes are modelled [Citation10]:
Frank-Read sources (generation of mobile dislocations ρm)
Emission of mobile dislocations ρm from static dislocations ρs in the subgrain wall
Immobilisation of mobile dislocations ρm - generation of static dislocations ρs
Recovery by climbing processes (=static recovery) for mobile and static dislocations.
Spontaneous annihilation (=dynamic recovery) of mobile and static dislocations
Subgrain boundaries are formed by static dislocations
Subgrain coarsening, subgrain nucleation and the Zener pinning effect of precipitates
Most of these mechanisms correspond to the original concept of Ghoniem [Citation5]. In addition, a number of terms have been adapted, refined and/or modified in the creep models [Citation4,Citation6,Citation10]. The current, most comprehensive version of the creep model is described in the book chapter [Citation10]. Compared to the previous fundamental model published in 2020 [Citation4], the damage model has been significantly improved in the 2022 work [Citation10] considering two aspects:
the description of cavitation damage was optimised (better tertiary creep region), and
the model for damage due to precipitate coarsening was removed, since potential ‘damage effects’ due to coarsening are already implicitly included due to the effect of precipitates on the motion of mobile dislocations as well as on subgrain coarsening in point (g) above.
This adaptation of the model resulted in a better transition region between primary and secondary creep. The creep strain rate , Equationeq. (1)
(1)
(1) , and the effective dislocation velocity veff, Equationeq. (2)
(2)
(2) , can now be calculated as follows:
In Equationequation (1)(1)
(1) b denotes the Burgers vector, ρm represents the mobile dislocation density, veff is the effective dislocation velocity, M is the Taylor factor and Dcav denotes the cavitation damage factor. Meanwhile, in Equationequation (2)
(2)
(2) , vg represents the glide velocity and vc represents the climb velocity of dislocations. EquationEquation (2)
(2)
(2) has been modified by Witzmann et al. [Citation11] compared to sources [Citation4] and [Citation10] to take into account the impact of needle and plate-shaped particles. Instead of using precipitation radii rp,i and densities NV,i, the ‘effective’ precipitation radii rp,i,eff and densities NV,i,eff have been introduced. More details for this will be presented in the next section.
Morphology of the precipitates
Since precipitates can have different morphologies, a specific shape factor C(h) was introduced by Sonderegger et al. [Citation12] in order to include different impacts of spherical, plate-shaped, as well as needle-shaped precipitates with one single factor, leading to the calculation of an effective radius rp,eff and an effective precipitate density Nv,eff [Citation11]. It should be noted here that this factor must be derived specifically for each crystal system of a matrix and the factor mentioned in this paper refers to bcc.
In the extension of the creep model in reference [Citation11], the spherical precipitates in the subgrain interior are replaced by rotational ellipsoids, since these can also be used to describe needle- or plate-shaped precipitates. For rotational ellipsoids, the shape can be defined with the help of two parameters: with the semi-axes ai (bi=ai) and ci. With these semi-axes, an aspect ratio hi can be defined according to Equationeq. (3)(3)
(3) :
An aspect ratio of h > 1 corresponds to a needle-shaped precipitate, an aspect ratio of h < 1 to a plate-shaped precipitate, and h = 1 describes a spherical precipitate.
The equations for the effective precipitate density Nv,eff and the effective radius of precipitates rp,eff are given by Equationeq. (4)(4)
(4) and (Equation5
(5)
(5) ), and the associated parameter C(h) is provided in Equationeq. (6)
(6)
(6) [Citation12].
It should be noted that Equationeq. (6)(6)
(6) already considers all main variants of relative orientations between precipitates’ rotational axes and the slip systems of the bcc crystal in a statistical manner. It should also be noted that Equationeq. (4)
(4)
(4) -(Equation6
(6)
(6) ) and also the effective dislocation velocity, Equationeq. (2)
(2)
(2) , only apply to precipitates within subgrains. The precipitates located at the subgrain boundaries are considered within the subgrain mobility and the Zener pinning effect.
A cross-section of an ellipsoidal precipitate (h = 4) within a glide plane is visualised in . In a spherical precipitate with the same volume as the spheroidal precipitate is shown, and the multiplication of the radius from (b) with the specific shape factor C(h) to obtain the radius rp,eff is shown in .
Figure 2. Cross section of (a) an ellipsoidal precipitate with h=4; (b) a spherical precipitate with the same volume; (c) application of the parameter C(h) on the radius of the precipitate.

demonstrates the need of the parameter C(h): lengthy precipitates are more likely to intersect a slip plane compared to spherical particles of the same volume. In the case of an aspect ratio of h = 4, the probability for a particle to intersect a slip plane is increased by 43% (statistical mean value). We consider this effect by changing the number density NV into an effective number density NV,eff. In addition, the mobile dislocations have to climb higher distances for overcoming those particles (both statements do not necessarily hold for each individual interaction, but for statistically considering all potential relative orientations). For details of the derivation, the reader is referred to the original paper by Sonderegger et al. [Citation12].
Material and experimental details
In the following simulations, the 9% Cr-steel P91 was used as material, since there is a large amount of literature and experimental data available for this material. In order to obtain the precipitate data in as-received state as well during creep, simulations of the precipitation kinetics were carried out with the software package MatCalc 6.02 (based on the SFFK model [Citation13]). For this, the thermodynamic database ‘mc_fe_v2.060.tdb’ and the mobility database ‘mc_fe_v2.012.ddb’ were used, as well as the chemical composition summarised in .
Table 1. Chemical compositions of simulated P91 (wt.%) [Citation14].
Microstructural input parameters for the simulation were taken from various literature sources, whereby more detailed information can be found in reference [Citation4]. shows the starting values selected for the simulation of the material P91 at 650°C/70 MPa.
Table 2. Microstructural input parameters (start values) for P91 in as-received (normalised + tempered) condition before creep [Citation16].
Since not all parameters of the creep model are directly accessible experimentally, some have to be slightly optimised with the help of one single master creep curve. These are: the damage parameter A, the prefactor of the glide velocity a1, the source density β of mobile dislocations emitted from statics in the subgrain boundary walls, the pipe diffusion coefficient Dvp, the Holt constant Kc and the activation volume Vr. In order to obtain optimal values for these parameters, an optimisation algorithm based on a simple gradient method was added into the existing Matlab code from Mergl [Citation17]. Nevertheless, these values should be viewed critically, although Krenmayr [Citation18] provided some guidelines for the range of these parameters in P91. Furthermore, the activation volume Vr can be roughly estimated with the help of relaxation data [Citation4].
In Witzmann et al. [Citation11], two different parameter studies have been carried out. In parameter study 1, the modelled creep curve according to the previous model [Citation4] (with spherical precipitates in the subgrain interior) is tested against an experimental creep curve. Parameter study 2 tests the experimental curve against the improved model considering aspect ratios of precipitates. In order to simulate a realistic microstructure of P91, NbC and AlN were assumed to be spherical (see [Citation19] for NbC in P91). No morphology data on AlN were available for the authors. However, their phase fraction is negligible. TEM images of P91 from Carrizo et al. ( in reference [Citation20]) show that VN are needle-shaped. This morphology for VN was also found by Hättestrand et al. ( in reference [Citation21]) in EFTEM images for P92. Another source – Aydogan et al. [Citation22] - describes acicular vanadium carbo-nitrides for 12% chromium steels, which were investigated by STEM. VN is therefore considered to be acicular. For the sake of completeness, it should be mentioned that VN can also be plate-shaped [Citation19,Citation23].
Therefore, an aspect ratio of h = 4 was chosen for a needle-like VN and NbC & AlN were accordingly simulated as spherical precipitates with h = 1.
Results and discussion
In the following, three parameter studies are presented. Studies 1 and 2 demonstrate the effect of precipitates on individual creep curves and microstructural evolution, whereas study 3 demonstrates the impact of precipitates on TTR diagrams. It should be noted that some parts of the results of studies 1 and 2 have already been presented by Witzmann et al. [Citation11].
The final set of simulation parameters for both parameter studies 1 & 2 is shown in (Ω represents the atomic volume). It should be noted here that if the morphology of the precipitates is changed, only the parameters a1 and A have to be adjusted [Citation11].
Table 3. Final set of remaining simulation parameters of parameter study 1 & 2.
The simulated creep curves for the two parameter studies, the experimental creep curve and the ± 19% scatter band (according to EN ISO 204) are shown in . The experimental curve in and also in the following images represent an averaged curve from over 20 experiments taken from Krenmayr [Citation18].
Figure 3. Experimental and simulated creep curves of P91, 650°C, 70 MPa; (a) parameter study 1 (spherical VN) h=1; (b) parameter study 2 (needle-shaped VN) h=4 (modified from [Citation11]).
![Figure 3. Experimental and simulated creep curves of P91, 650°C, 70 MPa; (a) parameter study 1 (spherical VN) h=1; (b) parameter study 2 (needle-shaped VN) h=4 (modified from [Citation11]).](/cms/asset/cab6a894-1c55-4e99-b47e-e6717e1dfb63/ymht_a_2310389_f0003_b.gif)
It can be seen that in parameter study 1 - - especially the primary and secondary creep regions for spherical precipitates in the subgrain interior are excellently reproduced. The simulation of the tertiary region, on the other hand, deviates slightly from the experimental creep curve. In - parameter study 2 - all three regions of the simulated creep curve are almost identical with the experimental creep curve, which means on the one hand that a simulation with elongated VN maps the creep curve more realistically and furthermore, that a very good parameter choice was made.
General strategy
In order to demonstrate the influence of the precipitates inside the subgrain on the creep curve, the two parameter studies are compared using various different simulations. Both parameter studies include:
Simulations without precipitates (VN, NbC & AlN) in the subgrain interior.
Simulations with different variations of the aspect ratio h for VN. NbC and AlN are again considered to be spherical. It should be noted that h = 1 corresponds to a spherical precipitate, h < 1 to a plate-like precipitate, and h > 1 to a needle-like precipitate.
In each case, spherical chromium carbides M23C6 are assumed to be located at the subgrain boundaries.
In addition to the results presented in [Citation11], we present the microstructural evolution of the matrix depending on different morphologies of the precipitates in the subgrain interior and also the effect of these precipitate shapes on the TTR diagram, which are shown in the following sections.
Parameter study 1
shows the simulated creep curves of parameter study 1 (see for parameters), the experimental creep curve and the ± 19% scatter band (according to EN ISO 204).
Figure 4. Creep curves for different variations of the precipitates inside subgrains with the fitting parameters of the parameter study 1; *without precipitates = without VN, NbC & AlN inside subgrains (modified from [Citation11]).
![Figure 4. Creep curves for different variations of the precipitates inside subgrains with the fitting parameters of the parameter study 1; *without precipitates = without VN, NbC & AlN inside subgrains (modified from [Citation11]).](/cms/asset/7b3494a9-d28e-4f21-95df-63bef0381d03/ymht_a_2310389_f0004_c.jpg)
As shown in , the calculated creep curve for h = 1 (spherical) reproduces the primary and secondary creep stage excellently. The variant of the creep curve without precipitates in the subgrain interior is closer to the experimental creep curve in terms of the rupture time, but shows stronger deviations in the secondary creep stage compared to experimental data.
If the lifetimes are compared, the lifetime of the experimental averaged creep curve [Citation18] with 8503 h (at 6% elongation) is similar to the lifetime of the fitted curve for h = 1 with 9096 h and also to the creep curve, which has no precipitates in the subgrain interior (8706 h). Simulations of needle-shaped precipitates of the VN with h = 4 and h = 6, show a significantly higher lifetime compared to the result for spherical precipitates with 10,113 h and 12,030 h, respectively. For the creep curve simulations with plate-shaped VNs, the increase in lifetime is less pronounced than for needle-shaped precipitates. An aspect ratio of h=¼ corresponds to a lifetime of 9670 h and h=⅙ corresponds to a lifetime of 10,275 h.
Remarkable is the minimal difference in lifetime of spherical precipitates in contrast to the lifetime without precipitates in the subgrain-interior of only 4%. If we assume that subgrain-interior precipitates have significant impact on the creep lifetime, this difference appears too low and suggests improper model assumptions (spherical particles).
Parameter study 2
shows the simulated creep curves of parameter study 2 (see for parameters), the experimental creep curve and the ± 19% scatter band (according to EN ISO 204).
Figure 5. Creep curves for different variations of the precipitates inside subgrains with the fitting parameters of the parameter study 2; *without precipitates = without VN, NbC & AlN inside subgrains (modified from [Citation11]).
![Figure 5. Creep curves for different variations of the precipitates inside subgrains with the fitting parameters of the parameter study 2; *without precipitates = without VN, NbC & AlN inside subgrains (modified from [Citation11]).](/cms/asset/b01907dc-9eb6-474c-a88a-9df9f6417141/ymht_a_2310389_f0005_c.jpg)
For parameter study 2, the deviation of the lifetimes (in %) of several different aspect ratios is shown in . The master creep curve with needle-shaped VN (h = 4) served as reference point.
Table 4. Deviation in % depending on the aspect ratio of VN [Citation11].
As mentioned earlier, the master creep curve (h = 4) for parameter study 2 achieved an excellent agreement with the experimental creep curve. If no precipitates in the subgrain interior are considered, the creep curve of this simulation shows the lowest lifetime of all variants. The second lowest lifetime is obtained by a simulation with spherical precipitates. As in parameter study 1, needle-shaped VN precipitates lead to a stronger increase in creep life than plate-shaped precipitates – corresponding values can be found in . The differences between the individual simulations now appear much more realistic. For example, taking VN into account with an aspect ratio of h = 4 increases the service life (compared to the same simulation without VN) by 16%. An aspect ratio of h = 6 even results in a 38% longer creep life compared to the VN-free simulation.
Microstructural evolution for different morphologies of precipitates inside subgrains
In the following section, the influence of the morphologies of precipitates in the subgrain interior on the microstructural evolution is studied. shows the evolution of (a) mobile dislocations (density ρm), (b) static dislocations (density ρs), (c) boundary dislocations (density ρb), and (d) subgrains (radius Rsbg) over time. For the study of the microstructural evolution, the simulation parameters from parameter study 2 were used.
Figure 6. Microstructural evolution of (a) the mobile dislocation density; (b) the static dislocation density; (c) the boundary dislocation density; (d) the subgrain radius; *without precipitates = without VN, NbC & AlN inside subgrains.

As shown in and (b), precipitates in the subgrain interior have a significant influence on the mobile and static dislocation density. Up to a creep time of 250 h, only minimal differences are evident for the different variants. Differences start to become evident between 250 and 1000 h of creep. After 1000 h, the curves increase parallel in the same slope.
The boundary dislocation density () is also very similar at the beginning for all variants of the creep simulation. The difference between the different variants increases more with ongoing creep time. It is worth to mention, that the creep curve without precipitates in the subgrain interior already seems to converge towards a value at 7000 h, whereas the curve with the lowest boundary dislocation density (h = 6) still continues to drop after 8000 h.
is particularly remarkable – here almost no deviation is visible for all variants. This confirms the new model that precipitates in the subgrain interior have hardly any influence on the subgrain radius.
Parameter study 3 - time-to-rupture diagram (TTR-Diagram)
Since the complete set of parameters is known for the parameter study 2, a TTR diagram can be reconstructed from the calculated creep curves. For this purpose, creep curves for the stresses from 60 MPa to 120 MPa were simulated without any additional alterations. All input parameter remained the same, only the stresses were varied in intervals of 10 MPa. The variation of applied stress changes the effective velocity in Equationeq. (2)(2)
(2) , since both glide and climb velocity contain a stress-dependent activation term – see Riedlsperger et al. [Citation4]. By contrast, a1, the pre-factor of the glide velocity in the modified Orowan’s law, is regarded as stress-independent, however impacting the modelled rupture times most significantly amongst all the non-fixed parameters stated in , see also [Citation16,Citation18]. The TTR diagram is obtained, when plotting the fracture times logarithmically against the stress, as it can be seen in . Furthermore, it should be noticed that in the TTR diagram different shapes of VN (in the subgrain interior) were distinguished, as it was already done in the variations of the creep curves and the microstructural evolution.
Figure 7. Time-to-rupture diagram for different morphologies of the precipitates inside subgrains; *without precipitates = without VN, NbC & AlN inside subgrains; reference data from ECCC [Citation24].
![Figure 7. Time-to-rupture diagram for different morphologies of the precipitates inside subgrains; *without precipitates = without VN, NbC & AlN inside subgrains; reference data from ECCC [Citation24].](/cms/asset/04bf7a89-8d96-46b6-9cbf-9f111ff7a980/ymht_a_2310389_f0007_c.jpg)
To compare the simulated data with experimental fracture times, the ECCC datasheet for P91 [Citation24] was used. For this purpose, the equation, which was obtained by the Manson-Haferd model from 2195 experimental points, was used to determine the rupture times for 650°C and the corresponding stresses.
As can be seen in , the reference curve (with h = 4 for VN) shows perfect agreement with the experimentally determined data of ECCC. The shape variations of the precipitates in the subgrain interior run parallel to the reference line.
Simulated rupture times react most sensitively to changes of the VN morphology from spherical to needle shape, whereas the effect of plate shapes on the TTR seems to be much less pronounced. It is particularly noteworthy that there is only a minimal difference for no precipitates in the subgrain interior or spherical precipitates in the subgrain interior.
Conclusion
The present work refines the existing dislocation creep model [Citation4] and emphasises the special importance of the morphology of precipitates in the subgrain interior. Until 2022 – [Citation11] - precipitates were always assumed to be spherical in the creep model of our working group. Since they can also be needle- or plate-shaped in alloys, an existing side-model for the impact of various particle shapes [Citation12] was implemented to better simulate the microstructure evolution. This is of particular relevance because the distance to be covered during climbing changes depending on the orientation and size of the precipitate.
In order to visualise the influence of spherical, needle-shaped or plate-shaped precipitates in the subgrain interior, parameter studies with different aspect ratios and simulations without precipitates in the subgrain interior were performed. Here it is evident, that not only the size of the precipitates or the number density but also the morphology (spherical, needle-shaped or plate-shaped) have a considerable influence on the creep curve and the fracture time. According to the original model [Citation4] without any shape effects (parameter study 1), VNs in the subgrain interior extend the service life by only 4%, whereas according to the new model (parameter study 2) by 16%. Accordingly, the new model with the assumption of acicular VN provides an improved simulation of the creep curve and also of the microstructure.
To reasonably validate the results of the creep curves, direct experiments regarding precipitates in the subgrain interior would be needed. Nevertheless, these simulations appear to provide quite realistic values.
The findings obtained are summarised below.
The existing dislocation creep model is refined & the particular importance of the morphology of the precipitates became apparent from the parameter studies
A more realistic microstructure can be simulated
Not only the size and number density of precipitates but also the morphology (needle-shaped, plate-shaped) affects the creep curve
Partial explanation of different creep behaviour, when different batches of material are compared with each other (±20% scatter band related to stress)
The different morphologies of the precipitates in the subgrain interior affect the evolution of the mobile and static dislocation density
Creep curve and TTR – diagram can be reproduced very accurately
Our creep curve and TTR simulation demonstrated that needle-like precipitates in the subgrain interior lead to the most significant extensions of creep life
Almost no difference in the TTR diagram was visible whether spherical precipitates or no precipitates were assumed in the subgrain interior
A possible further improvement is the implementation of lattice stresses into the model. Lattice stresses form around particles, as soon as the Youngs modulus of the particles differs from the matrix, and external forces are applied [Citation25]. Such stresses can potentially impact the glide and climb processes of dislocations near particles. This effect can increase the effective radius of particles and thus increase their impact on dislocation motion. Furthermore, the shape of the precipitates at the subgrain boundaries is currently not taken into account in the creep model. An extended model based on these precipitates located on subgrain boundaries would have an effect on the subgrain coarsening and thus improve the representation of the creep behaviour.
The current model for needle and plate-shaped precipitates is planned to be implemented in the software CreeSo [Citation26].
Contributor Roles Taxonomy (CRediT) author contributions
L. Witzmann (L.W.): conceptualisation, methodology, validation, formal analysis, data curation, writing – original draft, visualisation; J. Mergl (J.M.): methodology, software, data curation; F. Riedlsperger (F.R.): conceptualisation, data curation, writing – original draft, writing – review & editing; B. Krenmayr (B.K.): investigation, visualisation; G. Zuderstorfer (G.Z.): software; B. Sonderegger (B.S.): conceptualisation, methodology, formal analysis, resources, writing – original draft, writing – review & editing, supervision, project administration, funding acquisition
Acknowledgments
L.W., F.R, G.Z & B.S. gratefully acknowledge funding from Austrian Science Fund (FWF) within the project P-31374 “Software Development on Dislocation Creep in Alloys”. Furthermore, L.W. would like to thank the Linz Institute of Technology (LIT), the province of Upper Austria and the Federal Ministry of Austria – Education, Science and Research (BMBWF) for financial support within the project LIT-2021-10-SEED-115,01/899978 “MLSC - Model Development for Lifetime Assessment of Steels under Various Creep Loads”. J.M. gratefully acknowledge student funding from Johannes Kepler University (JKU) Linz. Financial support from Siemens Energy Global GmbH & Co. KG is gratefully acknowledged. The authors want to thank Dr Torsten Neddemeyer & Dr Torsten-Ulf Kern from Siemens Energy Global GmbH & Co. KG and Prof. Dr John Hald from DTU (Technical University of Denmark) for fruitful discussions.
Disclosure statement
No potential conflict of interest was reported by the author(s).
Additional information
Funding
References
- Zeiler G. Martensitic steels for rotors in ultra-supercritical power plants. In: editor Di Gianfrancesco A. Materials for ultra-supercritical and advance ultra-supercritical power plants. Woodhead Publishing Series in Energy; 2017, Vol. 104, pp. 143–174. doi: 10.1016/B978-0-08-100552-1.00006-3
- Hald J. Microstructure and long-term creep properties of 9–12% Cr steels. Int J Pres Ves Pip. 2008;85(1–2):30–37. doi: 10.1016/j.ijpvp.2007.06.010
- Holdsworth SR, Askins M, Baker A, et al. Factors influencing creep model equation selection. Int J Pres Ves Pip. 2008;85:80–88. doi: 10.1016/j.ijpvp.2007.06.009
- Riedlsperger F, Krenmayr B, Zuderstorfer G, et al. Application of an advanced mean-field dislocation creep model to P91 for calculation of creep curves and time-to-rupture diagrams. Materialia. 2020;12:100760. doi: 10.1016/j.mtla.2020.100760
- Ghoniem NM, Matthews JR, Amodeo RJ. A dislocation Model for creep in engineering materials. Res Mech. 1990;29:197–219.
- Yadav SD, Creep study of 9-12% Cr steels for power plants, PhD Thesis, Graz University of Technology, Austria, 2016.
- Basirat M, Shrestha T, Potirniche GP, et al. A study of the creep behavior of modified 9Cr-1Mo steel using continuum-damage modeling. Int J Plast. 2012;37:95–107. doi: 10.1016/j.ijplas.2012.04.004
- Kozeschnik E. Mean-field microstructure kinetics modeling. In: Caballero FG editor. Encyclopedia of materials: metals and alloys, 4. Oxford: Elsevier; 2022. pp. 521–526. doi:10.1016/B978-0-12-819726-4.00055-7
- Orowan E. Problems of plastic gliding. P Phys Soc. 1940;52(1):8–22. doi: 10.1088/0959-5309/52/1/303
- Sommitsch C, Sonderegger B, Ahmadi M, et al. Microstructurally based modeling of creep deformation and damage in Martensitic Steels. in: Creep Deformation, IntechOpen; 2022. doi: 10.5772/intechopen.104381.
- Witzmann L, Mergl J, Riedlsperger F, et al. Quantitative physikalische Modellierung der Auswirkung von Ausscheidungen im Subkorninneren auf die Kriechkurve und die Lebensdauer von martensitischen 9-12% Cr-Stählen, in Proceedings of 45th FVWHT Conference; November 2022; Düsseldorf, Germany. p. 57–68.
- Sonderegger B, Kozeschnik E, Sommitsch C. Modeling particle distances of coherent prolate- and oblate-shaped precipitates in bcc systems. Mater Sci Forum. 2012;706–709:1521–1526. doi: 10.4028/www.scientific.net/MSF.706-709.1521
- Svoboda J, Fischer FD, Fratzl P, et al. Modelling of kinetics in multi-component multi-phase systems with spherical precipitates I: theory. Mat Sci Eng A Struct. 2004;385:166–174. doi: 10.1016/j.msea.2004.06.018
- Hahn B, Bendick W. Pipe steels for modern high-output power plants. Pt1. Metallurgical principles - long-term properties, recommendations for use, 3R. Int. 2008;47:398–407.
- Panait CG, Zielińska-Lipiec A, Koziel T, et al. Evolution of dislocation density, size of subgrains and MX-type precipitates in a P91 steel during creep and during thermal ageing at 600°C for more than 100,000h. Mat Sci Eng A Struct. 2010;527(16–17):4062–4069. doi: 10.1016/j.msea.2010.03.010
- Riedlsperger F, Krenmayr B, Zuderstorfer G, et al., Mikrostrukturbasierte Modellierung des Versetzungskriechens von martensitischen 9-12% Cr-Stählen. Anwendung, Extrapolation, Tests und Verifikation, in Proceedings of 43rd FVWHT Conference, November 2020, Online Event, pp. 51–62.
- Mergl J, Numerical simulation and optimization of microstructure-based creep models, Diploma Thesis, Johannes Kepler University Linz, Austria, 2022.
- Krenmayr B, Mikrostrukturbasierte Kriechmodellierung von martensitischen Stählen und Untersuchung möglicher Abweichungen beim Kriechexperiment, PhD Thesis, Graz University of Technology, Austria, 2020.
- Czyrska-Filemonowicz A, Zielińska-Lipiec A, Ennis PJ. Modified 9% Cr steels for advanced power generation: microstructure and properties. J Achiev Mater Manuf Eng. 2006;19:43–48.
- Carrizo DA, Besoky JI, Luppo M, et al. Characterization of an ASTM A335 P91 ferritic-martensitic steel after continuous cooling cycles at moderate rates. J Mater Res Technol. 2019;8(1):923–934. doi: 10.1016/j.jmrt.2018.07.004
- Hättestrand M, Andrén H-O. Evaluation of particle size distributions of precipitates in a 9% chromium steel using energy filtered transmission electron microscopy. Micron. 2001;32:789–797. doi: 10.1016/S0968-4328(00)00086-X
- Aydogan E, Gigax JG, Parker SS, et al. Nitrogen effects on radiation response in 12Cr ferritic/martensitic alloys. Scr Mater. 2020;189:145–150. doi: 10.1016/j.scriptamat.2020.08.005
- Maruyama K, Sawada K, Koike J. Strengthening mechanisms of creep resistant tempered Martensitic Steel. ISIJ Int. 2001;41(6):641–653. doi: 10.2355/isijinternational.41.641
- Bendick W, Cipolla L, Gabrel J, et al. New ECCC assessment of creep rupture strength for steel grade X10CrMoVNb9-1 (grade 91. Int J Pres Ves Pip. 2010;87(6):304–309. doi: 10.1016/j.ijpvp.2010.03.010
- Weyer R, The Modelling of Damage due to Diffusional Creep in High Chromium Steels, Master Thesis, University of Cape Town, South Africa, 2016.
- Zuderstorfer G, Riedlsperger F, Sonderegger B. CreeSo – software for creep simulation of complex alloys. Mater High Temp. 2022;39:596–602. doi: 10.1080/09603409.2022.2058237