ABSTRACT
Objectives: Both iron and omega-3 (n-3) fatty acids (FA) play important roles in the development and functioning of the brain. We investigated the effects of n-3 FA and iron deficiencies, alone and in combination, during early development on behaviour and brain monoamines in rats. Methods: Using a 2-factorial design, female Wistar rats were randomly allocated to one of four diet groups: Control, n-3 FA deficient (n-3 FAD), iron deficient (ID), or n-3 FAD + ID. Females received these diets throughout mating, pregnancy and lactation. Offspring (n = 24/group; male:female = 1:1) continued on the same diet until post-natal day 42–45, and underwent a sucrose preference test (SPT), novel object recognition test, elevated plus maze (EPM) and social interaction test (SIT). Results: ID offspring consumed less sucrose in the SPT and spent more time in closed arms and less time in open arms of the EPM than non-ID offspring. In female offspring only, ID and n-3 FAD reduced time approaching and together in the SIT, with an additive effect of ID and n-3 FAD for even less time approaching and spent together in the n-3 FAD + ID group compared to controls. ID offspring had higher striatal dopamine and norepinephrine and lower frontal cortex dopamine concentrations. N-3 FAD and ID affected frontal cortex serotonin concentrations in a sex-specific manner. Conclusions: Our results suggest that ID and n-3 FAD during early development provoke anhedonia, anxiety and social dysfunction in rats, with potential additive and attenuating effects when combined. These effects may in part be attributed to disturbances in brain neurochemistry and may be sex-specific.
Introduction
Adequate nutrition during early life is critical for optimal neurodevelopment and may modulate the risk of developing neuropsychiatric disorders later in life [Citation1]. It has been estimated that one-third of children younger than five years residing in low- and middle-income countries (LIMC) fail to reach basic milestones in cognitive or psycho-social development, with malnutrition being a main contributing factor [Citation2]. Especially in LMIC, it is likely that multiple nutrient deficiencies co-exist during pre- and early postnatal life with potentially compounding effects on neurodevelopmental outcomes. Both iron and omega-3 fatty acids (n-3 FA) play crucial roles in brain development.
Iron deficiency (ID), the most common micronutrient deficiency in the world, is highly prevalent in women of childbearing age and children [Citation3]. It plays a central role in neuronal growth, differentiation and myelination, being a cofactor for enzymes involved in cell division, synthesis of neurotransmitters, and myelination [Citation4]. High concentrations of iron are found in cortico-limbic brain regions with high dopaminergic activity and in regions that receive inputs from gamma-aminobutyric acid (GABA) fibres, suggesting a functional relationship between iron and neurotransmission [Citation5].
Inadequate n-3 long-chain polyunsaturated fatty acid (LCPUFA) status, on the other hand, has been observed in many populations, especially in countries adopting a more Westernised diet [Citation6]. Approximately 35% of the adult brain dry weight consists of lipids in the form of LCPUFA, with docosahexaenoic acid (DHA; 22:6n-3) comprising 10–20% of the brain’s fatty acid (FA) composition [Citation7]. Like iron, DHA and eicosapentaenoic acid (EPA; 20:5n-3) play important roles in neurogenesis, myelination and neurotransmission [Citation8]. In rats, maternal DHA deficiency was shown to result in impaired offspring neurogenesis and behavioural alterations associated with altered dopaminergic function [Citation9].
Data on potential interactions between iron and n-3 FA deficiencies, particularly in relation to neurodevelopmental outcomes, are scarce. An earlier depletion study in post-weaning male rats by Baumgartner et al. [Citation10] indicated that combined iron and n-3 FA deficiency disrupts monoaminergic neurotransmission to a greater extent and produces greater cognitive deficits than a single deficiency.
Besides effects on cognitive development, deficiencies in iron and n-3 FA have also been associated with anxiety and depressive disorders [Citation11–13]. It has been suggested that depression and anxiety are directly caused by either a dysfunction of the monoaminergic neurotransmitter systems within the amygdala, prefrontal cortex and hippocampus, or a dysfunction within these brain areas itself [Citation14]. Both prenatal ID and n-3 FAD alone have been shown to affect monoaminergic neurotransmission in the offspring. For example, offspring born to ID anaemic dams showed aberrations in monoamine concentrations in several brain regions, even after receiving iron treatment postnatally [Citation15]. On the other hand, n-3 FAD diets were shown to cause increased synaptic neurotransmitter levels and decreased storage pools through two proposed processes: (1) alterations in cerebral membrane fluidity and permeability, and (2) changes in specific gene regulation [Citation16].
Therefore, the aim of this study was to investigate the effects of iron and n-3 FA deficiencies, alone and in combination, during early development on behaviour related to cognition, anhedonia, anxiety and social dysfunction, and cortico-limbic brain monoamines in rats. We further explored whether effects are sex-specific. We hypothesised that a double-deficiency during early development affects offspring cognition, behavioural outcomes and cortico-limbic brain monoamine levels to a greater extent than a single deficiency, and potentially in a sex-specific manner.
Materials and methods
Animals and housing
All experiments were approved by the AnimCare Ethics Committee (National Health Research Ethics Council registration number AREC-130913-015) of the Faculty of Health Sciences of the North-West University (NWU), South Africa (project number NWU-00335-15-S5; approval date: 13 November 2015). The experiments were conducted following the 4R principles for animal research [Citation17], and reported according to the Animals in Research: Reporting In Vivo Experiments (ARRIVE) guidelines [Citation18]. The experiments were conducted in Wistar rats, which were bred and housed in a temperature and humidity-controlled (22 ± 2°C and 55 ± 15% relative humidity) animal facility (Vivarium of the Preclinical Drug Development Platform of the NWU) with a reversed 12 h dark–light cycle (lights off at 06:00am). Rats were pair-housed in standard, individually ventilated, solid floor cages (Techniplast GR900 SealSafe® Plus; 300 × 300 × 189 mm) with low trace-element (<2.00 ppm Fe) alpha-cellulose bedding (Alpha-Dri®; LBS Serving Biotechnology Ltd, Horley, UK), and had ad libitum access to food and water (two bottles nanopure water,18MΩ). Cages were enriched with a plastic tube to provide a resting and nesting area, trace-element free nesting material (Kimtech Science, Precision Wipes™), and a chewing device (Bio-Serv® Nylon Bone; 101 × 25 mm) to avoid incisor malocclusion and overgrowth. Rats were handled regularly, and were monitored by means of recording body weights and completing study-specific checklists three times/wk.
Study design and diets
A total of 48 female Wistar rats aged 21 ± 3 days (postnatal day [PND] 21) with a minimum weight of 25 g were randomly allocated to one of four diet groups: (1) control (n = 8); (2) iron deficient (ID; n = 14); (3) n-3 FA deficient (n-3 FAD; n = 14); or (4) ID + n-3 FAD (n = 12) ().
Figure 1. Flow diagram of the experimental design; n indicates the numbers in each group; * indicates an equal number of male and female rats. GD: Gestational day; ID: Iron deficient; n-3 FAD: n-3 fatty acid deficient; ID + n3 FAD: iron and n-3 fatty acid deficient.
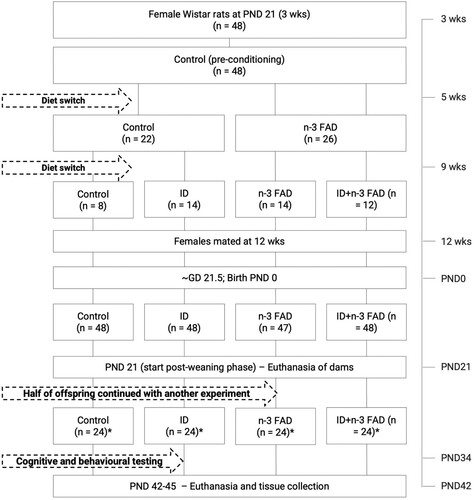
At PND 21, all female rats were placed on the control diet for a two-week period of preconditioning. After the preconditioning period, rats randomly allocated to an n-3 FAD diet group (n-3 FAD or ID + n-3 FAD) were placed on the n-3 FAD diet for six weeks, imitating a chronic n-3 FAD diet [Citation19], while the remaining rats continued to receive the control diet. Two weeks before mating, rats that were randomly allocated to an ID diet group were placed on their respective ID or ID + n-3 FAD diets [Citation20].
After 8 weeks on the experimental diets (11 weeks old), the female rats were placed in individual cages and mated 1:1 with approximately 12-week-old male breeders (habituated with the AIN-93G diet) of the same strain. During the mating period, animal handling was kept to a minimum and took place only during the light phase to not disturb mating. Conception was confirmed by the presence of a vaginal plug and set as gestational day (GD) 0. Male breeders were removed after conception, and females maintained their pre-pregnancy diet throughout pregnancy. During the gestational period, rats were monitored daily, and maternal body weights were recorded three times/wk. Dams were allowed to deliver spontaneously at approximately GD 22 (PND 0). At birth, litter size was recorded. Litters were culled to eight pups to maintain nutritional adequacy at PND 3–5, with ideally four males and four females per litter [Citation21]. Dams were maintained on their respective experimental diet throughout lactation.
At weaning (PND 21), offspring were randomly selected (n = 24 per experimental group, 1:1 sex ratio; 1–4 from each litter) and maintained on the same experimental diet (housed in same-sex pairs) as allocated to their dams until PND 42–45. Behavioural tests were conducted from PND 31–41, and offspring were euthanised for tissue collection at PND 42–45.
The purified experimental diets (Supplemental Table 1) were commercially obtained (Dyets, Bethlehem, PA, USA) and based on the AIN-93G [Citation22] formulation with modifications in iron content and fat source. All diets contained 10% fat. The basal AIN-93G formulation contained 40 mg iron, 70 g soybean oil and 30 g hydrogenated coconut oil/kg diet [Citation22]. The n-3 FAD diets contained 81 g hydrogenated coconut oil and 19 g safflower oil/kg diet [Citation10]. The ID diets contained 15–18 mg iron/kg diet [Citation20]. Iron content and FA composition of the diets were analysed in spot samples from each batch by Covance Laboratory Services (Madison, WI, USA) before shipping (Supplemental Table 2). All diets were custom prepared, vacuum packed in 2 kg bags and stored at −20°C until use. Diets were thawed in batches in a refrigerator (4°C). Once the bags were opened, diets were kept in an airtight container.
Tissue collection and analysis
Tissue collection and processing. Offspring at PND 42–45 were decapitated, and trunk blood collected into pre-chilled, 4 ml EDTA-coated and trace element free tubes (BD Vacutainer®). Haemoglobin (Hb) concentrations were measured in whole blood with a Coulter Analyzer (Vivarium, NWU Potchefstroom). After decapitation, brain tissue was rapidly removed and separated into two hemispheres along the midline on an ice-cool glass Petri dish. The frontal cortex, striatum and hippocampus were isolated from both hemispheres by freehand dissection [Citation23]. Brain regions were snap-frozen in liquid nitrogen and stored at −80°C until analysis. Snap frozen samples were homogenised and aliquoted for biochemical analysis as described by Erikson et al. [Citation24].
Brain iron analysis. Crude homogenates of the specific brain regions were digested with nitric acid according to Erikson et al. [Citation24] and total iron concentrations were measured by using the hydrogen reaction mode on an Agilent 7900 quadrupole ICP-MS at the Central Analytical Facilities, Stellenbosch University, South Africa. Samples were introduced via a 0.4 ml/min micromist nebuliser into a peltier-cooled spray chamber at a temperature of 2°C. NIST-traceable quality control standards at high and low concentration levels (De Bruyn Spectroscopic Solutions, Bryanston, South Africa) were analysed to verify the accuracy of the calibration before sample analysis commenced and this was repeated for every 12 samples to monitor drift. A germanium (Ge) internal standard was introduced online to monitor instrument drift and correct for matrix differences between samples and standards. During the analysis, internal standard recovery was between 90 and 110% for all samples, and recovery for drift monitor standards between 95 and 105%. Oxide formation was less than 0.3%. Three replicate measurements were completed for each sample.
Total phospholipid fatty acid analysis. Total phospholipid FA analyses were performed in crude homogenates of the specific brain regions as described previously by Baumgartner et al. [Citation10]. Lipids were extracted from brain tissue samples with chloroform:methanol (2:1, v:v; containing 0.01% butylated hydroxytoluene) using a modification of the Folch method [Citation25]. The lipid extracts were concentrated and the neutral lipids separated from the phospholipids by thin layer chromatography (TLC) (Silica gel 60 plates, 10 × 3x20 cm, Merck), trans-methylated to yield fatty acid methyl esters, and analysed by quadrupole (QP) gas chromatography electron ionisation mass spectrometry (GC-EI-MS) on an Agilent Technologies 7890A GC system equipped with an Agilent Technologies 5975C VL mass selective detector. Data analysis was performed using Agilent Quantitative Analyses software (Version B.05.02/Build 5.2.365.0). Relative percentages of FA were calculated by taking the concentration of a given FA derivative as a percentage of the total concentration of all FA identified in the sample.
Brain monoamine analysis. Monoamines and monoamine derivates (3,4-dihydroxyphenylacetic acid, DOPAC; dopamine, DA; norepinephrine, NE; serotonin, 5HT) were measured in crude hippocampal, striatal and frontal cortex homogenates. Monoamine concentrations were measured following a modified version of the method described by Zheng et al. [Citation26]. 50 µl homogenate containing 5 mg tissue were used for analysis. Caffeic acid (Sigma, CAS-331-39-5) was used as internal standard (IS). 5 µl of 50 µg/ml caffeic acid was added to 95 µL ascorbic H2O (Pure molecular grade H2O containing 2 mM ascorbic acid (Sigma, A92902) and 2.5 µg/ml caffeic acid IS). Samples were vortexed for 10s and sonicated in an ice bath for 15 min. 500 µL ice-cold methanol was added and the solution was bead-beaten (QIAGEN TissueLyser) at 20 Hz for 10 min, followed by centrifugation at 13’000 rpm for 2 min. The supernatant was carefully transferred to a 2 ml round bottom tube. The methanol supernatant was evaporated by using nitrogen. Completely dry samples were derivatised by adding 50 µL benzoyl-chloride and 50 µL tetra-borate, vortexed for 5 min and the re-suspended pellet incubated at 37°C for 10 min. The derivatised sample was centrifuged at 13,000 rpm for one minute and a 50 µL aliquot was transferred to a glass vial for LC-MS/MS analysis. The standard curves were prepared in parallel, following the same sample preparation methodology as described above. In short, 200 µL of a 10 µg/mL neurotransmitter stock solution containing a mix of dopamine (DA; Sigma-Aldrich H8502), 3,4-dihydroxyphenylacetic acid (DOPAC; Sigma-Aldrich D9128), norepinephrine (NE; Sigma-Aldrich A7256), and serotonin (5HT; Sigma-Aldrich 14927), was dried under nitrogen. The dried pellet was derivatised by adding 100 µL benzoyl-chloride and 100 µL tetra-borate, vortexed for 5 min and incubated at 37°C for 10 min. A 50/50 benzoyl-chloride and tetra-borate mix was used as diluent and 100 µL was added to each vial. 100 µL of the derivatised 10 µg/mL neurotransmitter stock solution was removed, and serial dilution from a 5 µg/mL concentration to 0.001 µg/mL, by means of serial dilution 10, 5, 2.5, 1.25, 0.624, 0.312, 0.156, 0.078, 0.039, 0.019, 0.009, 0.004, 0.002, 0.001 µg/mL.
Samples were analysed by using LC-MS/MS (Agilent 6410 Triple Quad LC/MS). Each sample was maintained at 4°C in a thermostatic auto sampler. The injection volume was 2.5 µL. The chromatographic separation was achieved on an Agilent ZORBAX Eclipse Plus C18, Narrow Bore 2.1mm × 150 mm column, 5-µ with the column temperature set at 30°C. The mobile phase consisted of solvent A (0.1% formic acid and 2.0 mM ammonium acetate in water) and solvent B (100% acetonitrile). The mobile phases were eluted at 0.3 mL/min according to the following gradient: 30% B maintained for 1.5 min, increased to 75% at 3.5 min and held for 11.5 min, decreased to 40% at 18.0 min, and then decreased to 5% at 22.0 min followed by 2.0 min for equilibration. The flow was diverted to the waste in the initial 4.5 min. The mass spectrometer operated at: 300°C gas temperature, 10L/min gas flow, nebuliser pressure of 25psi and capillary voltage of 3500 V. The electrospray ionisation source was operated in the positive mode with a 30 ms dwell time for each ion transition. Instrument control, data acquisition, and qualitative data analysis was done with MassHunter software for LC/MS systems.
Behavioural tests
Sucrose preference test. The sucrose preference test (SPT) is a measure of anhedonia, the reduced ability to experience pleasure from rewarding or enjoyable activities, by comparing the behavioural preference for drinking a sucrose solution in water, compared to the preference of water only, evident in many neuropsychiatric manifestations, especially depression [Citation27]. The SPT was performed as previously described [Citation28]. Briefly, rats remained housed in same-sex pairs to control for confounding by social isolation, with free access to food and two drinking bottles per cage. Total 24 h liquid intake was calculated by weighing the water bottles at the same time each day. On test day 1 (PND 34), both bottles contained nanopure water and 24 h consumption data were used to determine side preference. On days 2 and 3 (PND 35–36), one of the nanopure water bottles were replaced with a bottle containing a 2% sucrose solution in nanopure water. The sucrose bottle was randomly positioned on either the left or right-hand side of the cage on day 2 and swapped to the other side on day 3. Sucrose preference was calculated as the 2-day average percentage sucrose over total liquid intake.
Novel object recognition test. The novel object recognition test (NORT) measures cognitive ability through recognising previously encountered events and relies on the animal’s natural inclination to explore novel environments or objects [Citation29]. Cognitive deficits are a common comorbid feature of many psychiatric disorders, e.g. depression, schizophrenia etc. [Citation30]. The test was performed during the dark (active) phase as described previously [Citation31]. Prior to the experiment, rats were randomly placed in a noise isolated and poorly lit (60lux) experimental room, for 30-minutes of acclimation. The rats were exposed to a 10 min habituation session for two consecutive days (PND 37–38) in an empty Plexiglas open field box (70 × 70 × 40 cm). On the third day (PND 39), two identical plastic objects (familiar object) were placed in opposite corners of the box, 10 cm away from the walls. Each rat was allowed to explore the objects for 5 min (acquisition trial) before returning to their home cage for 90 min. The box and objects were thoroughly cleaned, both objects removed, and one familiar object was replaced with a novel unfamiliar object. Following the 90 min inter-trial interval, rats were returned to the box and allowed to explore the familiar and novel object (5 min retention trial). Total time spent in active exploration was measured with Noldus Ethovision XT (2018) by tracking the nose, centre and tail point of each rat [Citation32], using footage recorded with a centrally mounted video camera. Active exploration was defined as the rat either touching the object with its nose or directing its nose at the object at a distance ≤2 cm. Data were expressed as the percentage of time spent exploring the familiar or novel object.
Elevated plus-maze test. The elevated plus-maze (EPM) measures anxiety-related behaviour in rats, which is a common comorbid feature of many psychiatric disorders, especially depression, schizophrenia [Citation30]. This test is based on their innate fear of open and elevated spaces. The test was performed on PND 40 between 07h00 and 12h00 as described previously [Citation31]. Briefly, the plus sign-shaped-maze consists of two open (50 × 10 cm) and two closed arms (5 cm walls; 5 × 10 × 50 cm) connected by a central platform (10 × 10 cm). The closed arms are arranged to be across from one another. The maze was elevated to 50 cm, and a video camera was mounted above its centre. After testing room habituation (≥30 min), the rats were placed in the central platform, facing an open arm, and allowed to explore the maze for 5 min while being videotaped. Relative time spent in the open and closed arms, and total number of times each arm was entered was measured with Noldus Ethovision XT (2018) and expressed as percentages.
Social interaction test. The social interaction test (SIT) was used to measure deficits in social behaviour [Citation33], which has been associated with psychiatric disorders and the dysfunction of serotonin and dopamine neurotransmission [Citation34]. The social interaction test was performed on PND 40 between 13h00–17h00 as described previously [Citation31]. During the test, two rats of the same sex, diet group, and approximate weight (±10 g), that were unfamiliar with each other, were placed in the centre of an opaque Perspex® container (100 × 100 × 40 cm) that was illuminated with red light (40lux) and had a video camera mounted above its centre. After providing 30s for adaptation, the percentage time spent in active social interactive (i.e. rearing, anogenital sniffing, approaching and staying with the partner) and self-directed (i.e. self-grooming and total distance moved) behaviours were scored over 10 min. While experiments were observed in real-time to detect aggressive behaviour and to stop the experiment accordingly, recorded video footage was used for behavioural analysis (Noldus Ethovision XT, 2018).
Statistical analyses
Data were analysed and expressed using IBM SPSS software (version 25) and Microsoft Excel (Office 365). Data were examined for normality of distribution and the presence of outliers (boxplots and ±3 SD from the mean). Homogeneity of variance was examined with the Levene’s test. Variables that deviated significantly from normality and/or variance homogeneity were transformed prior to inferential statistical analysis. The effects of pre- and post-natal diets and their interactions with sex were analysed using 2 × 2x2 analysis of variance (ANOVA) (Fe[sufficient/deficient] × n-3 FA[sufficient/deficient] × sex[male/female]). If a sex-diet interaction was observed, ID and n-3 FAD effects and potential interactions were assessed by 2 × 2 ANOVA (Fe[sufficient/deficient] × n-3 FA[sufficient/deficient]); otherwise sex was adjusted for in subsequent 2 × 2 analysis of covariance (ANCOVA). When a significant diet effect or interaction was observed, a one-way ANCOVA with diet group as a between-subject variable and sex as a covariate was performed, followed by a Bonferroni test for multiple pair-wise comparisons. Effects of diet group without an interaction are interpreted as additive effects of deficient diets, whereas an interactive effect implies synergism or antagonism. Data are expressed as mean ± SEM. A P-value <0.05 was considered statistically significant.
Results
Offspring litter size, body weight and weight gain
The effects of pre- and postnatal ID and n-3 FAD, and ID × n-3 FAD interactions, on offspring litter size, body weight and weight gain are shown in . Litter size was not affected by diet. There were significant ID x n-3 FAD interactions for body weight at birth (PND 0) and at weaning (PND 21); the ID + n-3 FAD offspring had significantly lower body weight at these time points compared to the n-3 FAD group, but not compared to controls. Consequently, body weight gain from PND 0 until PND 21 was also significantly lower in the ID + n-3 FAD group compared to offspring in the n-3 FAD group.
Table 1. Litter sizes, body weights and weight gain in offspring.
An ID x sex interaction was observed for weight gain from PND 21 to PND 45 (P = 0.001). In male offspring, there were effects of both ID and n-3 FAD for lower body weight gain (ID, P < 0.001; n-3 FAD, P = 0.006), resulting in additively lowered body weight gain in the ID + n-3 FAD group compared to controls. In female offspring, there was only an effect of ID for lower body weight gain (ID, P = 0.012). No diet x sex interactions were observed for total body weight at PND 45, but there were effects for both ID and n-3 FAD for lower body weight (ID, P < 0.001; n-3 FAD, P = 0.010), resulting in additively lowered body weights in the offspring in the ID + n-3 FAD group compared to controls.
Haemoglobin and brain tissue iron concentrations
No diet x sex interactions were observed for Hb and brain tissue iron concentrations. There was a significant effect of ID for lower Hb concentrations at PND 45 (), with a trend for an effect of n-3 FAD (P = 0.057) for lower Hb. ID offspring also showed lower hippocampal (P = 0.001), striatal (P < 0.001) and frontal cortex (P = 0.001) iron concentrations. In the striatum, iron concentrations were lower in the ID (P < 0.001) and ID + n-3 FAD (P < 0.001) groups compared to controls. In the hippocampus and frontal cortex, only the ID + n-3 FAD group (P = 0.030; P = 0.046) had significantly lower tissue iron concentrations compared to controls.
Table 2. Haemoglobin, and tissue iron concentrations and fatty acid composition of the different brain regions in offspring at PND 45.
N-3 and n-6 fatty acids in brain tissue
The n-3 FAD diets significantly altered the n-3 and omega-6 (n-6) total phospholipid FA composition of different brain region tissue homogenates from offspring at PND 45 (), with no significant diet x sex interactions.
N-3 FAD resulted in lower alpha-linolenic acid (ALA; 18:3n-3), DHA and total n-3 FA in hippocampal, striatal and frontal cortex tissue (P < 0.001, all regions and FA). N-3 FAD resulted in lower hippocampal and striatal EPA (P < 0.001, both brain areas) but had no effect on frontal cortex EPA (P = 0.180).
N-3 FAD further resulted in lower linoleic acid (LA; 18:2 n-6), and higher arachidonic acid (AA; 20:4 n-6) and total n-6 FA in hippocampal, striatal and frontal cortex tissue. There was an effect of ID for higher LA in all three brain regions (P < 0.001, all regions). N-3 FAD increased the total n-6:n-3 ratio in hippocampal, striatal and frontal cortex tissue (P < 0.001, all regions).
Sucrose preference test
There was a significant effect of ID (P = 0.001) for lower relative intake of sucrose solution compared to water in the SPT ((A)), with no sex x diet interaction. While there was no significant effect of n-3 FAD on sucrose intake, between-group analysis revealed that only the ID + n-3 FAD offspring consumed significantly less sucrose compared to the control group (P = 0.028).
Figure 2. Sucrose preference test (SPT), elevated plus maze test (EPM) and novel object recognition test (NORT) results. (A) Percentage of sucrose consumed in the SPT by male and female rats (ID P = 0.001). (B) Percentage of time spent in the open (ID P = 0.020) and closed arms (ID P = 0.008) of the EPM by male and female rats. (C) Percentage time spent exploring familiar (n-3 FAD P = 0.034) and novel objects during the NORT. Two-way ANCOVA was used to test effects of ID, n-3 FAD, and ID x n-3 FAD interactions, adjusted for sex. The results that were not normal distributed were log transformed to perform ANCOVA. Between-group differences were determined using one-way ANCOVA followed by Bonferroni’s post-hoc test (adjusted for sex). For the EPM, independent t-test were performed to determine significant difference between time spent in open vs closed arms. Values are means ± SEM, n = 22–24. Labelled means without a common letter differ significantly, ID, iron deficient; n-3 FAD; n-3 fatty acid deficient.

Elevated plus maze test
In the EPM test, there were no diet x sex interactions. There was an effect of ID for less time spent exploring the open arms (P = 0.020) and more time spent exploring the closed arms (P = 0.008) of the EPM compared to the controls ((B)). Consequently, offspring in the ID and ID + n-3 FAD groups spent an equal amount of time exploring the open and closed arms, while the control and n-3 FAD rats spent notably more time exploring the open than the closed arms.
Novel object recognition test
In the NORT, there were no diet x sex interactions. There was an effect of n-3 FAD for less time spent exploring the familiar object (P = 0.034) ((C)), but there were no significant differences between groups. Furthermore, there was no effect of ID or n-3 FAD on time spent exploring the novel object (ID, P = 0.243; n-3 FAD, P = 0.890).
Social interaction test
In the SIT, a diet x sex interaction occurred for time spent approaching (n-3 FAD × sex; P = 0.020), together (ID × sex; P = 0.039; n-3 FAD × sex; P = 0.004) and moving away (n-3 FAD × sex; P = 0.039), as well as for anogenital sniffing (ID × n-3 FAD × sex; P = 0.002). In female offspring only, there was an effect of ID and n-3 FAD for less time spent approaching ((A)), together ((B)), and moving away ((C)), with an additive effect of ID and n-3 FAD for significantly less time spent approaching, together or moving away in female offspring that received the combined ID + n-3 FAD diet compared to controls. Females receiving an n-3 FAD diet spent more time anogenital sniffing (P = 0.011), however, this effect was attenuated by ID (ID × n-3 FAD, P < 0.001; (D)).
Figure 3. Social interaction test result. (A) The percentage of time female and male rats spent approaching one another (ID P < 0.001; n-3 FAD P = 0.001), (B) together (ID P < 0.001; n-3 FAD P < 0.001) and (C) moving away from each other (ID P < 0.001; n-3 FAD P = 0.001) during the SIT. (D) The percentage of time female and male rats spent anogenital sniffing (ID P = 0.033; n-3 FAD P = 0.011; IDxn-3FAD P < 0.001) during the SIT. Panels E and F show the amount of time the rats spent in self-directed behaviour: (E) Rearing (n-3 FAD P = 0.018) and (F) self-grooming (ID P = 0.011; IDxn-3 FAD P = 0.022). Two-way ANCOVA was used to test effects of ID, n-3 FAD, and ID x n-3 FAD interactions, adjusted for sex. The results that were not normal distributed were log transformed to perform ANCOVA. Between-group differences were determined using one-way ANCOVA followed by Bonferroni’s post-hoc test (adjusted for sex). Values are means ± SEM, n = 7–12, where split for sex (A, B, C, D) and n = 22–24 where results for male and female rats were combined (E, F). Labelled means without a common letter differ significantly. ID: iron deficient; n-3 FAD: n-3 fatty acid deficient.
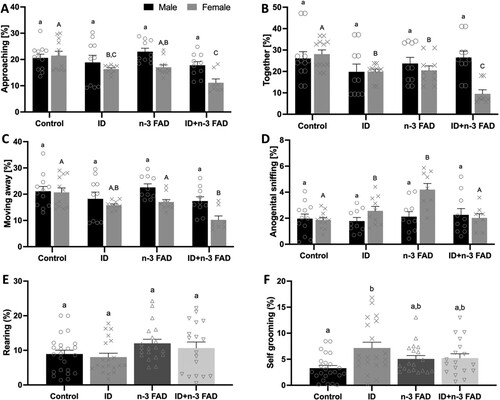
Male and female offspring receiving an n-3 FAD diet spent more time rearing (P = 0.018, (E)). ID increased the percentage of time spent self-grooming (P = 0.011, (F)), but this effect was attenuated when ID and n-3 FAD were combined (ID × n-3 FAD, P = 0.022).
Monoamines
The effects of ID and n-3 FAD, as well as ID × n-3 FAD interactions, on monoamine concentrations in the hippocampal, striatal and frontal cortex tissue homogenates are presented in Supplemental Table 3.
In the hippocampus, there was no effect of ID or n-3 FAD on any of the monoamines measured. In the striatum (), there was an effect of ID for higher NE (P = 0.028), DA (P = 0.004) and DOPAC (P = 0.004) concentrations, with no effect of n-3 FAD. In the frontal cortex (), there was an ID x n-3 FAD interaction on NE concentrations (P < 0.001); NE concentrations were significantly higher in the ID and n-3 FAD diet groups compared to controls, but these increases were attenuated in the combined ID + n-3 FAD group. In the frontal cortex, there was an ID effect for lower DA concentrations (P = 0.006). However, only the combined ID + n-3 FAD group showed lower DA concentrations compared to controls (P = 0.022). There was an ID x n-3 FAD interaction (P = 0.034) on frontal cortex DOPAC concentrations; the offspring in the n-3 FAD group had significantly lower DOPAC concentrations compared to controls, but this decrease was attenuated in the combined ID + n-3 FAD group.
Figure 4. Monoamine results for the striatum (NE, ID P = 0.012; DA, ID P = 0.006; DOPAC, ID P = 0.001). Two-way ANCOVA was used to test effects of ID, n-3 FAD, and ID x n-3 FAD interactions, adjusted for sex. The values were log transformed to perform ANCOVA. Between-group differences were determined using one-way ANCOVA followed by Bonferroni’s post-hoc test (adjusted for sex). Means with superscripts without a common letter differ (P < 0.05). Values are means ± SEM, n = 16–24 per group (n = 12 females, n = 12 males). ID: iron deficient; n-3 FAD: n-3 fatty acid deficient.
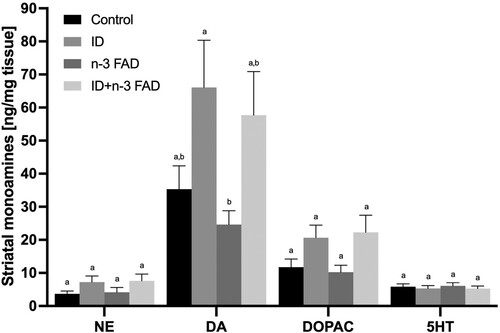
Figure 5. Monoamine results for the frontal cortex (NE, IDxn-3FAD P < 0.001; DA, ID P = 0.018; IDxn-3FAD P = 0.033; 5HT, ID P = 0.001; IDxn-3FAD P = 0.027). Two-way ANCOVA was used to test effects of ID, n-3 FAD, and ID x n-3 FAD interactions, adjusted for sex. The values were log transformed to perform ANCOVA. Between-group differences were determined using one-way ANCOVA followed by Bonferroni’s post-hoc test (adjusted for sex). Means with superscripts without a common letter differ (P < 0.05). Values are means ± SEM, n = 16–24 per group (n = 12 females, n = 12 males). ID: iron deficient; n-3 FAD: n-3 fatty acid deficient.
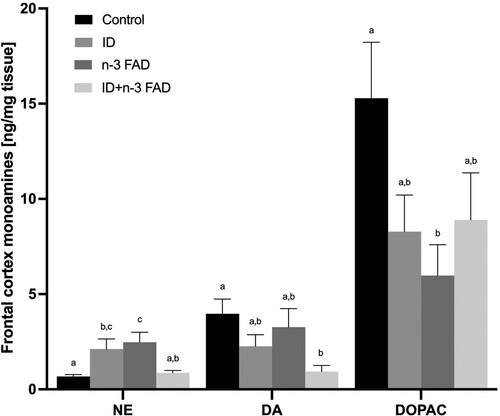
Furthermore, there were diet × sex interactions on frontal cortex 5HT concentrations (ID × sex, P = 0.018; n-3 FAD × sex, P = 0.012; ). In male offspring, there was an effect of n-3 FAD for lower 5HT concentrations (P = 0.005), with significantly lower 5HT concentrations in both the n-3 FAD and ID + n-3 FAD groups compared to controls. In female offspring, there was an ID x n-3 FAD interaction (P = 0.027) and ID effect (P < 0.001); the female offspring receiving the ID diet had significantly lower 5HT concentrations compared to controls, but this decrease was attenuated in the combined ID + n-3 FAD group. No significant diet × sex interactions on the measured monoamine concentrations were observed in any other brain regions.
Figure 6. Serotonin results for the frontal cortex split by sex (female, ID P = 0.001; IDxn-3FAD P = 0.027; male, n-3FAD P = 0.005). Two-way ANCOVA was used to test effects of ID, n-3 FAD, and ID × n-3 FAD interactions, adjusted for sex. The values were log transformed to perform ANCOVA. Between-group differences were determined using one-way ANCOVA followed by Bonferroni’s post-hoc test (adjusted for sex). Means with superscripts without a common letter differ (P < 0.05). Values are means ± SEM, n = 16–24 per group (n = 12 females, n = 12 males). ID: iron deficient; n-3 FAD: n-3 fatty acid deficient.
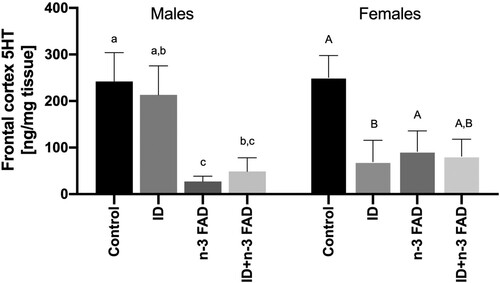
Discussion
This study in male and female Wistar rats demonstrates that both ID and n-3 FAD during early development provoke deficits in behaviour related to anhedonia, anxiety, and social dysfunction with potential additive and attenuating effects when combined. Interestingly, the effects of ID and n-3 FAD on social behaviour in the SIT were observed in female offspring only. Both ID and n-3 FAD during early development resulted in altered cortico-striatal monoamine concentrations. ID increased striatal DA and NE concentrations and lowered frontal cortex DA. Both ID and n-3 FAD alone increased frontal cortex NE concentrations but these increases were attenuated in the combined ID + n-3 FAD group. Furthermore, n-3 FAD and ID affected frontal cortex 5-HT concentrations in a sex-specific manner. The results from this study support our hypothesis that a combined iron and n-3 FA deficiency during early development in rats significantly affects striatal and frontal cortex neurochemistry that in turn may underlie the observed deficits in offspring behaviour. Moreover, combined deficiency has greater impact than a single deficiency. In contrast to our hypothesis, we found no clear effect of ID or n-3 FAD on cognition, specifically object recognition memory in the NORT. Sex-specific effects were observed for social behaviour and frontal cortex 5HT concentrations.
ID during early development significantly reduced sucrose preference in the SPT, implying that, at least in rodents, ID leads to the inability to experience pleasure in response to a rewarding stimuli (anhedonia) [Citation35]. Dysfunction of the reward system is closely related to alterations in the dopaminergic system [Citation36], with low DA typically associated with anhedonia in patients with depression [Citation37]. In the current study, ID offspring had significantly lower DA concentrations in the frontal cortex, albeit significantly higher concentrations of DA and its metabolite DOPAC in the striatum. This is consistent with previous studies in mice, which showed ID to disrupt dopamine pathways, especially when occurring during periods of rapid neural development [Citation38,Citation39]. It has also been shown in human studies that psychiatric disorders that present with mood, anhedonia, and psychotic manifestations such as schizophrenia had lower DA in the frontal cortex but elevated levels in the striatum [Citation40]. The mechanisms by which ID induces alterations in the dopaminergic system are not fully understood, but may be linked to the role of iron as co-factor for the enzyme tyrosine hydroxylase, which is responsible for dopamine production [Citation39]. Furthermore, studies in mice and Sprague–Dawley rats have shown ID to reduce the expression of the dopamine transporter and the dopamine D2 receptor [Citation39,Citation41]. Anhedonia may also be caused by noradrenergic dysregulation [Citation14]. We found that ID significantly increased striatal NE concentrations, which in turn may modulate DA release via action on α2A or α2C adrenergic receptors [Citation42]. Furthermore, offspring receiving an ID diet during early development had significantly higher frontal cortex NE concentrations, but this increase was attenuated in the offspring that received a combined ID + n-3 FAD diet. Increased NE concentration in ID rodents requires further study, but may be explained through the role of iron in the activity of the monoamine oxidase enzyme, critical in neurotransmitter degradation, as well as the activity of the norepinephrine transporter [Citation43]. Decreased NE concentrations have previously been observed in Wistar-Imanichi rats consuming an n-3 FA deficient diet [Citation44]. Whilst this may be a strain-related effect, it hints of a possible counterbalancing when these two deficiencies are combined.
In the EPM, ID offspring spent significantly less time exploring the open arms and significantly more time in the closed arms compared to their non-ID counterparts, implying increased anxiety-like behaviour. This agrees with previous studies in both Wistar and Sprague–Dawley rats that showed ID to be associated with anxiety-driven behaviour [Citation11,Citation45]. In contrast, Nishikura et al. [Citation46] found postweaning ID to have no effect on anxiety in juvenile Fischer rats when assessed using the EPM, although again a possible strain effect being involved. That said, we also observed ID-induced anxiety related behaviour during the SIT, with ID offspring spending significantly more time self-grooming [Citation47], but this effect was attenuated when combined with n-3 FAD. Increased anxiety has been linked to alterations in dopaminergic neurotransmission [Citation45], possibly involving n-3 FAD associated reversal of ID-mediated effects on NE and/or DA as noted above. Previous studies in Long-Evans rats further showed that females were less anxious during the EPM than male rats [Citation48], possibly involving the influence of oestrogen on the dopaminergic system [Citation49]. In our study, the effect of ID-induced anxiety-related behaviour assessed in the EPM was not sex-specific. Moreover, we did not find an n-3 FAD effect on anxiety-related behaviour in the EPM, which is in disagreement with previous neurodevelopmental studies in rodents [Citation44,Citation50]. Singh et al. [Citation50] reported 15-week-old n-3 FAD Sprague–Dawley males born to n-3 FAD dams (deficiency induced on second day of pregnancy) were significantly anxious, spending less time in the open arms than their n-3 FA sufficient counterparts. Similarly, Takeuchi et al. [Citation44] showed that 8-week-old n-3 FAD Wistar offspring born to n-3 FAD dams (deficiency induced during gestation) spent less time in the open arms. Overall, our results indicate that ID during early life may increase the risk of anxiety.
In the SIT, ID and n-3 FAD female offspring spent significantly less time approaching, together and moving away, with a significant additive effect of ID and n-3 FAD in the combined group. The deficiencies did not affect males. Thus, females may be more susceptible to develop psychosocial dysfunction induced by ID and n-3 FAD during early life. This is aligned with research in humans showing that social anxiety disorder is more prevalent in females than males, with increased symptoms observed in females particularly during puberty [Citation51]. Thus, the observed sex-specific differences in social and anxious behaviour are likely linked to interactions with sex-hormones [Citation52]. In a previous study in Sprague–Dawley rats, where ID was induced during pregnancy (embryonic day 3; ID diet containing 2–6 g/kg iron) and reversed at PND 7, the ID diet resulted in reduced social approach in male offspring (no females included in behavioural testing) [Citation53]. Similarly, various studies in humans have shown that early life ID can lead to changes in socio-emotional behaviour, such as a lack of engagement and increased apathy during social interactions in infancy and childhood [Citation54]. While it is not clear how prenatal and childhood n-3 FAD may impact social behaviour, n-3 FAD is closely associated with an increased risk for developing conditions such as depression, anxiety, bipolar disorder and attention-deficit/hyperactivity disorder which may indirectly impact social behaviour [Citation12]. Importantly, deficits in social and self-directed behaviour have been associated with a dysfunction in serotonergic and dopaminergic neurotransmission [Citation34,Citation55]. While we did not observe changes in striatal 5HT concentrations, n-3 FAD significantly lowered 5HT concentrations in the frontal cortex, which agrees with previous rodent studies showing increased frontal cortex 5HT receptor density, likely as an adaptation to reduced serotonergic function [Citation56,Citation57]. Indeed, various mechanisms may underlie how n-3 FAD potentially alters serotonergic neurotransmission, such as affecting the physical properties of neuronal membranes and membrane-bound proteins, by regulating gene expression, and/or promoting neuroinflammatory processes [Citation58,Citation59]. Of note, both striatal 5HT and DA have previously been shown to play a key role in social interaction deficits observed in rodent models of depression [Citation60], obsessive compulsive disorder [Citation61] and psychosis [Citation62].
The additive nature of the effects of ID and n-3 FAD on social behaviour indicates that the effects of ID and n-3 FAD are mediated by complementary but independent pathways. Thus, it can be speculated that ID affects social behaviour by affecting dopaminergic neurotransmission, while n-3 FAD may affect social behaviour by disrupting the serotonergic system. In the SIT, we further observed that n-3 FAD offspring spent more time rearing and anogenital sniffing. However, the effect for exaggerated anogenital sniffing, which may be associated with dominance-related behaviour, was attenuated when n-3 FAD was combined with ID. While excessive rearing can be associated with boxing, an aggressive and anti-social behaviour, based on our observations in the current study, excessive rearing was more likely a repetitive behaviour associated with a deficit in serotonin-modulated inhibitory control [Citation63,Citation64].
While n-3 FAD did have a significant effect to reduce time spent exploring the familiar object in the NORT, there were no significant differences between groups or an effect of ID or n-3 FAD on novel object recognition. Furthermore, we found no effect on exploration time (data not shown), which could have masked the results [Citation65]. Thus, neither diet significantly affected object recognition memory. This is in contrast with the results of the previous study by Baumgartner et al. [Citation10], which showed in male Wistar rats that ID and n-3 FAD significantly impair working and reference memory in the Morris Water Maze, with an additive effect on reference memory when combined.
A particular strength of this study is the inclusion of both male and female offspring that allowed us to explore sex-specificity of the applied dietary effects. Furthermore, the co-assessment of behaviour and brain neurochemistry draws an important parallel between cause and effect. To improve clinical translation to the human situation, we set about to induce moderate rather than severe ID anaemia, which is highly prevalent in women and children living in LMIC. Thus, the ID diets in the current model contained 15–18 mg iron/kg diet as opposed to 3 mg iron/kg diet used in most previous iron depletion rodent studies. The Hb results from this study confirmed that the ID diet induced moderate ID anaemia in the offspring (Hb of 9.45 ± 0.33 g/dL and 8.63 ± 0.28 g/dL in the ID and ID + n-3 FAD groups, respectively), and significantly reduced brain iron concentrations by 12.5–36.2%. In contrast, the n-3 FAD diets did not contain an n-3 FA source and reduced brain DHA levels in the Hip, Str and FC by 66.7–70.0%, 65.9–68.5% and 73.4–74.2%, respectively, compared to n-3 FA sufficient counterparts.
On the other hand, we acknowledge that the chronicity of exposure to a deficient diet spanning the prenatal to early adolescence period represents a relatively severe model. That said, the current rat model has relevance for public health translation, being designed to mimic the human situation where both male and female offspring are born to mothers with inadequate iron and/or n-3 FA intake and consequently status during pregnancy and lactation. Dietary habits are shaped in childhood and are mainly based on the parental dietary behaviour, influenced by their food environment [Citation66]. It can therefore be assumed that infants would be weaned to a diet similar to that of their mothers, therefore equally inadequate in iron and/or n-3 FA, and will continue to consume a deficient diet throughout childhood up to adolescence. Late adolescence and thereafter is when they are likely to make their own dietary choices. Thus, we chose postnatal day 42–45 as study endpoint, which corresponds to early adolescence in humans [Citation67].
The main limitation of this study is that the timespan across which the deficient diets stretched (prenatal period right through early adolescence) prevented us from identifying a critical window when the diets likely induced the observed effects, which should be explored in future studies. Furthermore, besides the analysis of monoaminergic neurotransmitters and their metabolites, it would have been valuable to investigate other mechanisms that may underlay the observed effects of ID and n-3 FAD, such as their impact on myelination, neurogenesis, oxidative stress, neuroinflammation, and brain energy metabolism. The observed effects of ID on body weight at birth and weaning could imply that some of the observed effects may be mediated by ID causing foetal growth restriction through changes in placental function and/or postnatal growth faltering [Citation68], which may be sex-specific and warrants further investigation. Furthermore, we observed large within-group variability for some of the brain monoamine measures, which may have masked some important changes. Also, the addition of another behavioural test, specifically the forced swim test as a measure for depression, to an already large test battery could have been of value.
In conclusion, this study in rats shows that ID and n-3 FAD, alone and in combination, during early life provoke deficits in behaviour, specifically anhedonia, anxiety and social dysfunction, potentially brought about by shifts in cortico-striatal neurotransmitter concentrations. Furthermore, ID and n-3 FAD may increase the risk for aberrant social behaviour in a sex-specific manner. Therefore, our results indicate that both dietary deficiencies during early life may be involved in the aetiology of neuropsychiatric manifestations, especially when present in combination. Future research is necessary to determine the mechanisms underlying the sex-specific effects of ID and n-3 FAD on social behaviour, and whether effects of ID and n-3 FAD induced during early development are reversible with the introduction of iron and n-3 FA after critical periods of neurodevelopment.
Supplemental Material
Download PDF (310.3 KB)Acknowledgements
We would like to thank the personnel of the North-West University Vivarium (Mr Cor Bester, Mr Kobus Venter and Mrs Antoinette Fick) for their assistance with executing the animal study. Furthermore, we would like to thank Dr Estelle Venter, Ms Mamokete Pule, Ms PJ Alley and Mrs Laetitia Jordaan for their help during the execution of the animal study. We thank Mrs Cecile Cooke and Dr Adriaan Jacobs for their assistance with the laboratory analyses. The authors would also like to acknowledge Prof DeWet Wolmarans and Dr Marisa Möller-Wolmarans for their valuable advice and training in the setting up and refinement of the Noldus Software and related behavioural protocols. C.M.S., J.B. and B.H.H. conceptualised and designed the study; E.T.K., J.B. and L.Z. supervised and executed the animal experimental procedures; E.T.K. and L.Z. supervised and performed the laboratory analyses; E.T.K., L.Z. and J.B. analysed the data; B.H.H advised on the behavioural tests used; all authors assisted in the interpretation of results; E.T.K., L.Z. and J.B. wrote the paper. All authors reviewed and approved the final manuscript.
Disclosure statement
No potential conflict of interest was reported by the author(s).
Data availability statement
The authors declare that all data associated with the current study are available from the corresponding author upon reasonable request.
Additional information
Funding
Notes on contributors
Erna T. Kemp
Erna T. Kemp is a Doctor of Philosophy (Ph.D.) candidate at the Centre of Excellence for Nutrition of the North-West University in Potchefstroom, South Africa. Her Ph.D. focuses on the neurodevelopmental effects of maternal iron and omega-3 fatty acid deficiency and depletion, alone and in combination, in the offspring.
Lizelle Zandberg
Lizelle Zandberg is a Senior Lecturer at the Centre of Excellence for Nutrition of the North-West University in Potchefstroom, South Africa. Her research is in the field of molecular nutrition where she studies the underlying molecular mechanisms of fatty acids and micronutrients as role players in neuro- and immune competence, using rodent models, and human intervention and observational studies.
Brian H. Harvey
Brian H. Harvey is a Professor at the Centre of Excellence for Pharmaceutical Sciences of the North-West University in Potchefstroom, South Africa, and programme leader in preclinical research at the South African Medical Research Council’s Unit on Risk and Resilience in Mental Disorders at the Department of Psychiatry and the Neuroscience Institute of the University of Cape Town in Cape Town, South Africa, and an Honorary Professor at the Institute for Mental and Physical Health and Clinical Translation, School of Medicine, Deakin University, Geelong, Australia. Brian employs translational rodent and zebra fish models to study the neurobiological basis of risk and resilience in human psychiatric disorders and uses these models as research platforms for novel drug development.
Cornelius M. Smuts
Cornelius M. Smuts is a Professor in Nutrition and the Director of the Centre of Excellence for Nutrition of the North-West University in Potchefstroom, South Africa. His research focuses on the effects of omega-3 fatty acids on maternal outcomes, the interactions between omega-3 fatty acids and micronutrients on cognition, behaviour and immune function, as well as different nutritional intervention strategies to improve infant growth and development.
Jeannine Baumgartner
Jeannine Baumgartner is a Lecturer in Nutritional Sciences at King’s College London and holds an honorary appointment as Extraordinary Associate Professor in Nutrition at the Centre of Excellence for Nutrition of the North-West University in Potchefstroom, South Africa. Currently, her research focuses on studying the role of micronutrients and omega-3 fatty acids in the development and functioning of the brain, bones, immune system, and gut microbiome in animal models, and human intervention and observational studies.
References
- Adan RAH, van der Beek EM, Buitelaar JK, Cryan JF, Hebebrand J, Higgs S, et al. Nutritional psychiatry: towards improving mental health by what you eat. Eur Neuropsychopharmacol. 2019;29(12):1321–32. doi:10.1016/j.euroneuro.2019.10.011. Eng.
- McCoy DC, Peet ED, Ezzati M, Danaei G, Black MM, Sudfeld CR, et al. Early childhood developmental status in low- and middle-income countries: national, regional, and global prevalence estimates using predictive modeling. PLoS Med. 2016;13(6):e1002034. doi:10.1371/journal.pmed.1002034.
- Wang M, Gao H, Wang J, Cao C, Ying X, Wei Y, et al. Global burden and inequality of iron deficiency: findings from the global burden of disease datasets 1990–2017. Nutr J. 2022;21(1):16. doi:10.1186/s12937-022-00771-3. Eng.
- Moos T, Skjørringe T, Thomsen LL. Iron deficiency and iron treatment in the fetal developing brain – a pilot study introducing an experimental rat model. Reprod Health. 2018;15(1):117–20. doi:10.1186/s12978-018-0562-z.
- Lozoff B. Early iron deficiency has brain and behavior effects consistent with dopaminergic dysfunction. J Nutr. 2011;141(4):740S–6. doi:10.3945/jn.110.131169.
- Stark KD, Van Elswyk ME, Higgins MR, Weatherford CA, Salem N. Global survey of the omega-3 fatty acids, docosahexaenoic acid and eicosapentaenoic acid in the blood stream of healthy adults. Prog Lipid Res. 2016;63:132–52. doi:10.1016/j.plipres.2016.05.001.
- McNamara RK, Carlson SE. Role of omega-3 fatty acids in brain development and function: potential implications for the pathogenesis and prevention of psychopathology. Prostaglandins Leukot Essent Fatty Acids. 2006;75(4–5):329–49. doi:10.1016/j.plefa.2006.07.010.
- Lauritzen L, Brambilla P, Mazzocchi A, Harsløf LBS, Ciappolino V, Agostoni C. DHA effects in brain development and function. Nutrients. 2016;8(1). doi:10.3390/nu8010006.
- Basak S, Mallick R, Banerjee A, Pathak S, Duttaroy AK. Maternal supply of both arachidonic and docosahexaenoic acids is required for optimal neurodevelopment. Nutrients. 2021;13(6):2061. doi:10.3390/nu13062061.
- Baumgartner J, Smuts CM, Malan L, Arnold M, Yee BK, Bianco LE, et al. Combined deficiency of iron and (n-3) fatty acids in male rats disrupts brain monoamine metabolism and produces greater memory deficits than iron deficiency or (n-3) fatty acid deficiency alone. J Nutr. 2012;142(8):1463–71. doi:10.3945/jn.111.156281. Eng.
- Bonuti R, Horiquini-Barbosa E, Morato S. Late effects of iron deficiency during gestation and lactation followed by iron replacement on anxiety-related behaviors and brain morphology of young adult rats. Psychol Neurosci. 2020;13(4):516–30. doi:10.1037/pne0000239.
- Lange KW. Omega-3 fatty acids and mental health. Global Health Journal. 2020;4(1):18–30. doi:10.1016/j.glohj.2020.01.004.
- Berthou C, Iliou JP, Barba D. Iron, neuro-bioavailability and depression. eJHaem. 2022;3(1):263–75. doi:10.1002/jha2.321.
- Liu Y, Zhao J, Guo W. Emotional roles of mono-aminergic neurotransmitters in major depressive disorder and anxiety disorders. Front Psychol. 2018;9. doi:10.3389/fpsyg.2018.02201.
- Unger EL, Hurst AR, Georgieff MK, Schallert T, Rao R, Connor JR, et al. Behavior and monoamine deficits in prenatal and perinatal iron deficiency are not corrected by early postnatal moderate-iron or high-iron diets in rats. J Nutr. 2012;142(11):2040–9. doi:10.3945/jn.112.162198. Eng.
- Tanaka K, Farooqui AA, Siddiqi NJ, Alhomida AS, Ong WY. Effects of docosahexaenoic acid on neurotransmission. Biomol Ther. 2012;20(2):152–7. doi:10.4062/biomolther.2012.20.2.152. Eng.
- du Sert NP, Hurst V, Ahluwalia A, Alam S, Avey MT, Baker M, et al. The ARRIVE guidelines 2.0: updated guidelines for reporting animal research. Br J Pharmacol. 2020;177(16):3617–24. doi:10.1111/bph.15193. Eng.
- Kilkenny C, Browne WJ, Cuthill IC, Emerson M, Altman DG. Improving bioscience research reporting: the ARRIVE guidelines for reporting animal research. PLoS Biol. 2010;8(6):e1000412. doi:10.1371/journal.pbio.1000412. Eng.
- Mathieu G, Oualian C, Denis I, Lavialle M, Gisquet-Verrier P, Vancassel S. Dietary n-3 polyunsaturated fatty acid deprivation together with early maternal separation increases anxiety and vulnerability to stress in adult rats. Prostaglandins Leukot Essent Fat Acids. 2011;85(3–4):129–36. doi:10.1016/j.plefa.2011.07.001. Eng.
- Greminger AR, Mayer-Pröschel M. Identifying the threshold of iron deficiency in the central nervous system of the rat by the auditory brainstem response. ASN Neuro. 2015;7(1). doi:10.1177/1759091415569911.
- Chahoud I, Paumgartten FJ. Influence of litter size on the postnatal growth of rat pups: is there a rationale for litter-size standardization in toxicity studies? Environ Res. 2009;109(8):1021–7. doi:10.1016/j.envres.2009.07.015. Eng.
- Reeves PG. Components of the AIN-93 diets as improvements in the AIN-76A diet. J Nutr. 1997;127(5 Suppl):838s–41s. doi:10.1093/jn/127.5.838S. Eng.
- Chiu K, Lau WM, Lau HT, So K-F, Chang RC-C. Micro-dissection of rat brain for RNA or protein extraction from specific brain region. J Vis Exp. 2007;7:269.
- Erikson KM, Pinero DJ, Connor JR, Beard JL. Regional brain iron, ferritin and transferrin concentrations during iron deficiency and iron repletion in developing rats. J Nutr. 1997;127(10):2030–8. doi:10.1093/jn/127.10.2030.
- Folch J, Lees M, Sloane Stanley GH. A simple method for the isolation and purification of total lipides from animal tissues. J Biol Chem. 1957;226(1):497–509. doi:10.1016/S0021-9258(18)64849-5.
- Zheng X, Kang A, Dai C, Liang Y, Xie T, Xie L, et al. Quantitative analysis of neurochemical panel in rat brain and plasma by liquid chromatography–tandem mass spectrometry. Anal Chem. 2012;84(22):10044–51. doi:10.1021/ac3025202.
- Liu M-Y, Yin C-Y, Zhu L-J, Zhu X-H, Xu C, Luo C-X, et al. Sucrose preference test for measurement of stress-induced anhedonia in mice. Nat Protoc. 2018;13(7):1686–98. doi:10.1038/s41596-018-0011-z.
- Grundwald NJ, Brunton PJ. Prenatal stress programs neuroendocrine stress responses and affective behaviors in second generation rats in a sex-dependent manner. Psychoneuroendocrinology. 2015;62(1):204–16. doi:10.1016/j.psyneuen.2015.08.010.
- Ennaceur A, Delacour J. A new one-trial test for neurobiological studies of memory in rats. 1: behavioral data. Behav Brain Res. 1988;31(1):47–59. doi:10.1016/0166-4328(88)90157-X.
- Ma M, Zhang Y, Zhang X, Yan H, Zhang D, Yue W. Common and distinct alterations of cognitive function and brain structure in schizophrenia and major depressive disorder: a pilot study. Front Psychiatry. 2021;12, English. doi:10.3389/fpsyt.2021.705998.
- Mokoena M, Harvey B, Viljoen F, Ellis S, Brink C. Ozone exposure of flinders sensitive line rats is a rodent translational model of neurobiological oxidative stress with relevance for depression and antidepressant response. Psychopharmacology. 2015;232(16):2921–38. doi:10.1007/s00213-015-3928-8.
- Noldus LPJJ, Spink AJ, Tegelenbosch RAJ. Ethovision: a versatile video tracking system for automation of behavioral experiments. Behav Res Methods Instrum Comput. 2001;33(3):398–414. doi:10.3758/BF03195394.
- Möller M, Du Preez JL, Viljoen FP, Berk M, Emsley R, Harvey BH. Social isolation rearing induces mitochondrial, immunological, neurochemical and behavioural deficits in rats, and is reversed by clozapine or N-acetyl cysteine. Brain Behav Immun. 2013;30:156–67. doi:10.1016/j.bbi.2012.12.011.
- Sotoyama H, Inaba H, Iwakura Y, Namba H, Takei N, Sasaoka T, Nawa H. The dual role of dopamine in the modulation of information processing in the prefrontal cortex underlying social behavior. FASEB J. 2022;36(2):e22160. doi:10.1096/fj.202101637R.
- Kassir A. Iron deficiency: a diagnostic and therapeutic perspective in psychiatry. Encephale. 2016;43(1):85–9. doi:10.1016/j.encep.2016.08.002.
- Dichter GS, Damiano CA, Allen JA. Reward circuitry dysfunction in psychiatric and neurodevelopmental disorders and genetic syndromes: animal models and clinical findings. J Neurodev Disord. 2012;4(1):19. doi:10.1186/1866-1955-4-19.
- Brand SJ, Moller M, Harvey BH. A review of biomarkers in mood and psychotic disorders: a dissection of clinical vs. preclinical correlates. Curr Neuropharmacol. 2015;13(3):324–68. doi:10.2174/1570159X13666150307004545. Eng.
- Manduca A, Bara A, Larrieu T, Lassalle O, Joffre C, Layé S, Manzoni OJ. Amplification of mGlu 5 -endocannabinoid signaling rescues behavioral and synaptic deficits in a mouse model of adolescent and adult dietary polyunsaturated fatty acid imbalance. J Neurosci. 2017;37(29):6851–68. doi:10.1523/JNEUROSCI.3516-16.2017.
- Pino JMV, da Luz MHM, Antunes HKM, Giampá S, Martins VR, Lee KS. Iron-restricted diet affects brain ferritin levels, dopamine metabolism and cellular prion protein in a region-specific manner. Front Mol Neurosci. 2017;10, English. doi:10.3389/fnmol.2017.00145.
- Möller M, Swanepoel T, Harvey BH. Neurodevelopmental animal models reveal the convergent role of neurotransmitter systems, inflammation, and oxidative stress as biomarkers of schizophrenia: implications for novel drug development. ACS Chem Neurosci. 2015;6(7):987–1016. doi:10.1021/cn5003368. Eng.
- Unger EL, Bianco LE, Jones BC, Allen RP, Earley CJ. Low brain iron effects and reversibility on striatal dopamine dynamics. Exp Neurol. 2014;261:462–8. doi:10.1016/j.expneurol.2014.06.023. Eng.
- Uys MM, Shahid M, Harvey BH. Therapeutic potential of selectively targeting the α(2C)-adrenoceptor in cognition, depression, and schizophrenia-new developments and future perspective. Front Psychiatry. 2017;8:144. doi:10.3389/fpsyt.2017.00144. Eng.
- Beard J. Iron deficiency alters brain development and functioning. J Nutr. 2003;133(5 Suppl 1):1468s–72s. doi:10.1093/jn/133.5.1468S. Eng.
- Takeuchi T, Iwanaga M, Harada E. Possible regulatory mechanism of DHA-induced anti-stress reaction in rats. Brain Res. 2003;964(1):136–43. doi:10.1016/S0006-8993(02)04113-6.
- Li Y, Kim J, Buckett PD, Böhlke M, Maher TJ, Wessling-Resnick M. Severe postnatal iron deficiency alters emotional behavior and dopamine levels in the prefrontal cortex of young male rats. J Nutr. 2011;141(12):2133–8. doi:10.3945/jn.111.145946.
- Nishikura N, Hino K, Kimura T, Uchimura Y, Hino S, Nakao M, et al. Postweaning iron deficiency in male rats leads to long-term hyperactivity and decreased reelin gene expression in the nucleus accumbens. J Nutr. 2020;150(2):212–21. doi:10.1093/jn/nxz237.
- Kim DG, Gonzales EL, Kim S, Kim Y, Adil KJ, Jeon SJ, et al. Social interaction test in home cage as a novel and ethological measure of social behavior in mice. Exp Neurobiol. 2019;28(2):247–60. doi:10.5607/en.2019.28.2.247. Eng.
- Eseh R, Zimmerberg B. Age-dependent effects of gestational and lactational iron deficiency on anxiety behavior in rats. Behav Brain Res. 2005;164(2):214–21. doi:10.1016/j.bbr.2005.06.019.
- Erikson KM, Jones BC, Hess EJ, Zhang Q, Beard JL. Iron deficiency decreases dopamine D1 and D2 receptors in rat brain. Pharmacol Biochem Behav. 2001;69(3–4):409–18. doi:10.1016/S0091-3057(01)00563-9.
- Singh HB, Agrawal R, Sharma S, Huo Y-X, Ying Z, Gomez-Pinilla F. Omega-3 fatty acid deficiency during brain maturation reduces neuronal and behavioral plasticity in adulthood. PLoS One. 2011;6(12). doi:10.1371/journal.pone.0028451.
- Asher M, Asnaani A, Aderka IM. Gender differences in social anxiety disorder: a review. Clin Psychol Rev. 2017;56:1–12. doi:10.1016/j.cpr.2017.05.004.
- Kundakovic M, Rocks D. Sex hormone fluctuation and increased female risk for depression and anxiety disorders: from clinical evidence to molecular mechanisms. Front Neuroendocrinol. 2022;66:101010. doi:10.1016/j.yfrne.2022.101010.
- Kennedy BC, Dimova JG, Siddappa AJM, Tran PV, Gewirtz JC, Georgieff MK. Prenatal choline supplementation ameliorates the long-term neurobehavioral effects of fetal-neonatal iron deficiency in rats. J Nutr. 2014;144(11):1858–65. doi:10.3945/jn.114.198739.
- Chang S, Wang L, Wang Y, Brouwer ID, Kok FJ, Lozoff B, Chen C. Iron-deficiency anemia in infancy and social emotional development in preschool-aged Chinese children. Pediatrics. 2011;127(4):e927–33. doi:10.1542/peds.2010-1659.
- Kiser D, SteemerS B, Branchi I, Homberg JR. The reciprocal interaction between serotonin and social behaviour. Neurosci Biobehav Rev. 2012;36(2):786–98. doi:10.1016/j.neubiorev.2011.12.009.
- Delion S, Chalon S, Guilloteau D, Besnard JC, Durand G. Alpha-linolenic acid dietary deficiency alters age-related changes of dopaminergic and serotoninergic neurotransmission in the rat frontal cortex. J Neurochem. 1996;66(4):1582–91. doi:10.1046/j.1471-4159.1996.66041582.x.
- McNamara RK, Able J, Liu Y, Jandacek R, Rider T, Tso P, Lipton JW. Omega-3 fatty acid deficiency during perinatal development increases serotonin turnover in the prefrontal cortex and decreases midbrain tryptophan hydroxylase-2 expression in adult female rats: dissociation from estrogenic effects. J Psychiatr Res. 2009;43(6):656–63. doi:10.1016/j.jpsychires.2008.09.011.
- Grosso G, Galvano F, Marventano S, Malaguarnera M, Bucolo C, Drago F, Caraci F. Omega-3 fatty acids and depression: scientific evidence and biological mechanisms. Oxid Med Cell Longevity. 2014;2014:313570. doi:10.1155/2014/313570. Eng.
- Joffre C, Rey C, Layé S. N-3 polyunsaturated fatty acids and the resolution of neuroinflammation. Front Pharmacol. 2019;10:1022. doi:10.3389/fphar.2019.01022. Eng.
- Mncube K, Harvey BH. Bio-behavioural changes in treatment-resistant socially isolated FSL rats show variable or improved response to combined fluoxetine-olanzapine versus olanzapine treatment. IBRO Neuroscience Reports. 2022;13:284–98. doi:10.1016/j.ibneur.2022.08.009.
- Wolmarans W, Stein DJ, Harvey BH. Social behavior in deer mice as a novel interactive paradigm of relevance for obsessive-compulsive disorder (OCD). Soc Neurosci. 2017;12(2):135–49. doi:10.1080/17470919.2016.1145594. Eng.
- Swanepoel T, Möller M, Harvey BH. N-acetyl cysteine reverses bio-behavioural changes induced by prenatal inflammation, adolescent methamphetamine exposure and combined challenges. Psychopharmacology. 2018;235(1):351–68. doi:10.1007/s00213-017-4776-5. Eng.
- de Brouwer G, Fick A, Harvey BH, Wolmarans DW. A critical inquiry into marble-burying as a preclinical screening paradigm of relevance for anxiety and obsessive–compulsive disorder: mapping the way forward. Cogn Affect Behav Neurosci. 2019;19(1):1–39. doi:10.3758/s13415-018-00653-4.
- Szechtman H, Harvey BH, Woody EZ, Hoffman KL. The psychopharmacology of obsessive-compulsive disorder: a preclinical roadmap. Pharmacol Rev. 2020;72(1):80–151. doi:10.1124/pr.119.017772. Eng.
- Lueptow LM. Novel object recognition test for the investigation of learning and memory in mice. J Vis Exp. 2017;126. doi:10.3791/55718. Eng.
- Scaglioni S, De Cosmi V, Ciappolino V, Parazzini F, Brambilla P, Agostoni C. Factors influencing children's eating behaviours. Nutrients. 2018;10(6). doi:10.3390/nu10060706. Eng.
- Zeiss CJ. Comparative milestones in rodent and human postnatal central nervous system development. Toxicol Pathol. 2021;49(8):1368–73. doi:10.1177/01926233211046933.
- Roberts H, Bourque SL, Renaud SJ. Maternal iron homeostasis: effect on placental development and function. Reproduction. 2020;160(4):R65–78. doi:10.1530/REP-20-0271. Eng.