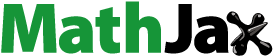
ABSTRACT
The assessment of asymmetric slab behaviour is out of reach in standard Falling Weight Deflectometer (FWD) tests, because deflections are measured along the driving direction only. Herein, a new T-shaped arrangement of the geophones is proposed. It allows for rapid quantification of asymmetric slab behaviour in central FWD testing of concrete slabs. One geophone is positioned at the centre of impact (= centre of the slab), six along the driving direction, one right and one left of the centre. The ‘Lateral Asymmetry Index (LASIX)’ is introduced as a corresponding dimensionless deflection basin parameter. Its value increases with increasing asymmetric behaviour of the slab. The main research challenge tackled herein is to optimise the radial distance of the lateral geophones from the centre of the slab, such as to maximise the expressiveness of LASIX for the quantification of asymmetric slab behaviour. In this context, FWD tests with measurement of deflections in eight different directions are carried out, and the ‘effective asymmetry index ()’ is introduced as another new dimensionless deflection basin parameter. It summarises the asymmetric behaviour based on deflection differences quantified for all 28 pairs of directions which can be combined out of the eight available measurement directions. The optimal radial distance of the lateral geophones from the centre of the slab is found as 1.20 m. Corresponding values of LASIX larger than
refer to coefficients of directional variation of the AREA7 parameter larger than 5%. This indicates directional degradation of the pavement structure resulting from eccentric traffic loads. T-shaped FWD testing requires in situ efforts equivalent to those of standard testing, while allowing for a rapid and reliable quantification of asymmetric behaviour. It allows for the assessment of whether the standard evaluation of uniform moduli of subgrade reaction is realistic or questionable.
1. Introduction
Falling Weight Deflectometry (FWD) is frequently used for non-destructive characterisation of concrete slabs. An FWD test consists of dropping a falling weight (= standardised mass) from a defined height onto a load plate placed on top of the pavement's surface. During the impact, geophones (= displacement sensors) measure the deflection history of several points at the surface of the pavement.
During standard FWD tests, surface deflections are measured along the driving direction, see (a). Several modifications to the standard FWD testing have been developed, e.g. the Light Weight Deflectometer which is a portable device used at places inaccessible to FWD-vehicles (Fleming et al., Citation2007, Nazzal et al., Citation2007), the Rolling Weight Deflectometer which performs measurements by means of a loaded wheel running over the pavement of interest (Bay et al., Citation1995, Briggs et al., Citation2000), and the Fast Falling Weight Deflectometer which allows for carrying out a large number of tests in a short period of time (Pratelli et al., Citation2018, Coni et al., Citation2021). All these approaches have in common that deflections are measured along the driving direction. This renders the assessment of asymmetric slab behaviour impossible and provides the motivation for the present paper.
Figure 1. Positions of geophones (see the • symbols) during central FWD testing on rigid pavements: top view onto the (a) standard approach with geophones aligned with the driving direction, (b) proposed T-shaped arrangement, where the lateral distance c is to be optimised, and (c) multi-directional testing according to Díaz Flores et al. (Citation2021), providing input data for the aforementioned optimisation.

Asymmetric slab behaviour during central FWD tests refers to different deflections recorded at the same radial distance from the centre of the slab, but along different directions, see (c) and (Díaz Flores et al., Citation2021). With increasing magnitude of these differences, deflections measured along the driving direction are decreasingly representative of the behaviour of the slab in other directions. This is problematic, because point-symmetric deflection basins are usually assumed when it comes to back-calculation of subgrade stiffness from deflections measured along the driving direction during central FWD tests. Herein, a new T-shaped arrangement of the geophones is proposed for rapid quantification of asymmetric slab behaviour in central FWD testing of concrete slabs.
One geophone is positioned at the centre of impact (= centre of the slab), six along the driving direction (N-direction), one right (E-direction) and one left (W-direction) of the centre, see (b), where N, E, and W refer to a local cardinal directional system. As for the quantification of asymmetric structural behaviour, we here introduce a dimensionless deflection basin parameter referred to as ‘Lateral Asymmetry Index’:
(1)
(1)
where ,
, and
refer to the deflections at the same radial distance r = c from the centre of the slab, but in the E-direction (right), W-direction (left), and N-direction (driving direction), respectively, and
denotes the absolute value of the difference of the deflections measured in the E-direction and the W-direction, at the radial distance r = c from the centre of the slab, see (b).
The main research challenge tackled herein is to optimise the radial distance c in Equation (Equation1(1)
(1) ) such as to maximise the informative content of LASIX regarding the quantification of asymmetric slab behaviour. This optimisation requires the evaluation of LASIX for different values of c and, therefore, the measurement of deflections during central FWD testing on several concrete slabs (i) not only in driving direction, but also right and left of the centre of the slab, and (ii) at several radial distances. The optimal value of c will be determined such that corresponding values of LASIX correlate in the best possible fashion with another newly introduced dimensionless deflection basin parameter: the effective asymmetry index
. The latter summarises the asymmetric behaviour of every tested slab in a detailed fashion because it contains information on differences of deflections measured (i) not only in driving direction, right, and left of the centre of the slab, but in eight different directions, and (ii) at several radial distances, see (c).
The present paper is organised as follows. Section 2 refers to the optimisation of the T-shaped arrangement of geophones based on experimental data from central FWD testing of 10 concrete slabs, with multi-directional measurements of deflections. For all 10 tested slabs, these data provide the basis for quantification (i) of the effective asymmetry index , and (ii) of the lateral asymmetry index LASIX for six different values of c. The optimal distance c will be identified such that associated values of LASIX exhibit the best possible correlation with
. Section 3 contains a discussion regarding the sources of asymmetric slab behaviour and their associated implications for the back-calculation of pavement properties from deflections measured during FWD testing. Section 4 contains the conclusions drawn from the results obtained from the presented study.
2. Optimisation of a T-shaped arrangement of geophones for rapid quantification of the asymmetric behaviour of concrete slabs in central FWD tests
2.1. Detailed asymmetry characterisation of 10 concrete slabs subjected to central FWD testing
The development of an optimised T-shaped arrangement of geophones for central FWD testing on concrete slabs requires comprehensive insight into the asymmetries of several slabs. This provides the motivation to perform multi-directional FWD tests (Díaz Flores et al., Citation2021) at the centres of 10 concrete slabs located on the Austrian highways ‘A1’ and ‘A2’. The tests were performed early in the morning of days during which no significant temperature variations were expected. This excluded problems resulting from slab curling due to temperature gradients (Ioannides and Khazanovich, Citation1998, Khazanovich et al., Citation2001).
Five tested slabs are part of the highway ‘A1’. Three of them had been in service for 22 years (‘old slabs’), the other two had been recently installed (‘new slabs’). All slabs have a thickness of 0.22 m and a length of 5.50 m. The widths of the slabs located on the acceleration lane, the first lane, and the emergency lane, are equal to 4.20 m, 3.80 m, and 3.20 m, respectively, see . The maximum forces imposed during central multi-directional FWD testing range from 201 kN to 203 kN.
Another five tested slabs are part of the highway ‘A2’. Three of them had been in service for 33 years (‘old slabs’), the other two had been recently installed (‘new slabs’). All slabs have a thickness of 0.22 m. Their length ranges from 4.50 to 5.60 m, and their width from 3.10 to 4.00 m, see . The maximum forces imposed during central multi-directional FWD testing range from 189 kN to 193 kN.
During multi-directional FWD testing, deflections are measured along eight specific radial directions, starting with the driving direction and proceeding clockwise as described in (Díaz Flores et al. Citation2021), , and , where N, E, S, and W refer to a local cardinal directional system with N pointing in the driving direction. Three tests are carried out immediately one after the other in every testing direction, in order to be able to assess test repeatability. After three such tests in all eight measurement directions, another final set of three tests is performed in the N direction, again for the sake of being able to assess test repeatability. Thus, the first and the ninth set of three tests correspond to the N direction.
During every single FWD test, nine geophones measure deflections of the surface of the slab. The first geophone (g = 1) is located at the centre of the slab, the other eight (g = 2 to g = 9) are fixed to a bar which ensures that the distances between them are constant. Structural constraints of the FWD-device make it necessary to move the bar 15 cm further away from the centre when measuring along the SE and SW directions, as compared to all the other directions, see .
Table 1. Properties of the 10 slabs characterised by means of multi-directional FWD testing.
In case that the outermost geophone(s) are located on the neighbouring slab or on the adjacent soil, their measurements are excluded. The number of excluded geophones are sometimes different in the E and W directions, see , because of small eccentricities of the FWD-device from the centre of the slab, which amounted to a few single centimetres.
All deflection maxima recorded by the geophones on the 10 tested slabs are listed in to . The deflection maxima measured on the slabs A2-54003 and A2-54440 are exemplarily illustrated in .
Test repeatability is satisfactory. The three to six (six tests were performed in driving direction, three tests in all other directions) tests performed in every direction delivered very similar deflection maxima in all radial distances, see e.g. the red zoom windows in (a) and (c). In all 27 tests performed per slab, the deflection maxima measured at the centre of impact are very similar, see the first columns of measured data in to . The first three tests and the last three tests, referring to the driving direction, delivered very similar results see e.g. the red zoom windows in (a) and (c) as well as data in to . This underlines that the support conditions of the 10 characterised slabs were stable during the approximately 45 minutes needed to perform a complete set of multi-directional FWD tests.
Figure 2. Arrangement of geophones during multi-directional FWD testing according to (Díaz Flores et al. Citation2021), and local cardinal directional system, with N referring to the driving direction.

Figure 3. Results from multi-directional FWD testing on the old slab A2-54003, see (a) and (b), as well as on the new slab A2-54440, see (c) and (d): the points refer to the deflection maxima listed in and , respectively, measured by the geophones along the N, S, E and W directions, see (a) and (c), as well as along the NE, SE, SW, and NW directions, see (b) and (d); the solid lines refer to splines interpolating between the average of the three (to six) deflections measured at each location.

Table 2. Polar angles of the eight different measurement directions.
Table 3. Radial distances of the geophones from the centre of impact (= centre of the slab), [m], as functions of the measurement direction:
,
,
,
,
,
,
,
.
Table 4. Geophones which were excluded since they were located outside the tested slab.
2.2. Quantification of the asymmetry of the structural behaviour by means of the new deflection basin parameter 

The deviation of the structural behaviour of the tested slabs from point symmetry with respect to the centre of impact (= centre of the slab) is quantified by means of the ‘effective asymmetry index’ which is a new deflection basin parameter introduced as
(2)
(2) where the relation between the summation index j and asymmetry indicators
is clarified in . The values of
quantify differences of deflections measured in two different directions d and δ, as introduced in (Díaz Flores et al., Citation2021), as
(3)
(3)
where both indexes d and δ run over the eight measurement directions: N, NE, E, SE, S, SW, W, and NW. Also in Equation (Equation3(3)
(3) ), r denotes the radial coordinate, while
and
stand for splines referring to the measurement directions d and δ, respectively. These splines (see e.g. the blue and black solid lines in ) interpolate between the average of the three (to six) deflection maxima measured at each point. Finally,
denotes the radial length of integration. Here,
is equal to
, except when comparing directions along which geophones were excluded, see . In these cases,
is equal to the distance from the centre of the slab to the last geophone that was included.
Table 5. Asymmetry indicators, according to Equation (Equation3
(3)
(3) ), quantifying the deviation of the measured structural behaviour from point symmetry with respect to the centre of impact (= centre of the slab); evaluated for the 10 tested slabs.
For every one of the 10 tested slabs, asymmetry indicators are evaluated according to Equation (Equation3(3)
(3) ) for all 28 combinations of two different directions out of the available eight measurement directions. The
asymmetry indicators are listed in .
according to Equation (Equation2
(2)
(2) ) refers to multi-directional FWD testing in eight different directions. The subscript ‘28’ to all 28 combinations of two different directions out of the eight available measurement directions. The effective asymmetry index
of every slab is obtained from inserting its 28 asymmetry indicators from into Equation (Equation2
(2)
(2) ), see for the results. The 10 obtained effective asymmetry indices allow for the correct classification of the tested slabs into ‘old slabs’ and ‘new slabs’, because
of all old/new slabs is larger/smaller than 4%. This corroborates the expressiveness of
regarding the assessment of asymmetric structural behaviour.
Table 6. Values of the effective asymmetry index, according to Equation (Equation2
(2)
(2) ), calculated for the 10 tested slabs; integrating the 28 asymmetry indicators of each slab (see ) into one single value.
2.3. Quantification of asymmetric structural behaviour based on T-shaped testing and the deflection basin parameter LASIX
A T-shaped arrangement of geophones is proposed with the aim to combine the advantages of standard and multi-directional FWD testing: (i) rapid in situ characterisation and (ii) expressiveness regarding the assessment of asymmetric structural behaviour. Surface deflections are measured by means of nine geophones: one at the centre of impact (= centre of the slab), six along the driving direction, one left and one right of the centre of impact, see (b).
The corresponding assessment of asymmetric structural behaviour is based on the deflection basin parameter LASIX, introduced in Equation (Equation1(1)
(1) ). The remaining open research question refers to optimising the radial distance c of the geophones from the centre of the slab, in order to maximise the informative content of LASIX for the quantification of asymmetric slab behaviour. This optimisation requires an evaluation of LASIX for different values of c, which is possible, because multi-directional testing delivers geophone measurements at so many positions, that focusing on subsets of the available geophone positions allows for simulating T-shaped arrangements of the geophones with several different values of c. LASIX is evaluated according to Equation (Equation1
(1)
(1) ) for six different values of c (0.30 m, 0.45 m, 0.60 m, 0.90 m, 1.20 m, and 1.50 m) and for all 10 slabs, see .
Table 7. Lateral Asymmetry Index, according to Equation (Equation1
(1)
(1) ), evaluated for different radial distances c of the geophones in E, W, and N directions.
2.4. Optimal distance c of the lateral geophones from the centre of the slab
The optimal radial distance c of the geophones from the centre of impact refers to the largest possible expressiveness of LASIX for the assessment of asymmetric structural behaviour. The related optimisation problem is solved as follows. For all six different values of c (0.30 m, 0.45 m, 0.60 m, 0.90 m, 1.20 m, and 1.50 m) the following steps are performed:
(1) | Ten values of LASIX, referring to T-shaped testing with one specific value of c, see the respective columns in , are correlated with the 10 corresponding values of | ||||
(2) | The best linear regression function is fitted to the pairs of values of LASIX and |
Table 8. Correlation between the values of in , referring to different values of the distance c, and values of
listed in .
The best correlation between LASIX, related to T-shaped FWD testing, and , related to multi-directional FWD testing, is obtained for deflections measured at a distance
from the centre of the slab, see . The corresponding maximum of the quadratic correlation coefficient amounts to
, see also . The values of LASIX referring to
allow for the correct classification of the tested slabs into ‘old slabs’ and ‘new slabs’. As for all new slabs, values of LASIX referring to
are smaller than or equal to 7.10%. As for all old slabs, values of LASIX referring to
are larger than or equal to 8.98%. This underlines the expressiveness of the newly introduced deflection-basin-parameter LASIX regarding the assessment of asymmetric structural behaviour. In , the threshold value of LASIX distinguishing old from new slabs was set equal to 8.00%.
The threshold value refers, according to the red regression line in , to
. This threshold value allows for the correct classification into old and new slabs in all but one case: the old slab A1-33874 with
and
, see and . Notably, this slab was part of an emergency lane, see . Therefore, it was subjected to traffic loading only indirectly, namely, because of load transfer via tie bars connecting the tested slab to its neighbour which was part of the first lane and, therefore, directly exposed to traffic loads. This underlines that values of
call for an engineering assessment of the specific exposure of the tested slab to eccentric traffic loads.
The construction of trailers containing a fixed installation of the optimal T-shaped arrangement of geophones appears to be feasible, because the optimal distance between the two lateral geophones, m, is smaller than the maximum allowed width of vehicles. Such trailers will facilitate in situ data acquisition, because the operators can stay inside their vehicle, as in standard FWD testing, i.e. there is no need for the operators to get out of their vehicle and manipulate the geophones, as required for multi-directional FWD testing.
2.5. Arrangement of the geophones remaining aligned with the driving direction
As for the six geophones which remain aligned with the driving direction, it is proposed to arrange them at radial distances amounting to 0.30 m, 0.60 m, 0.90 m, 1.20 m, 1.50 m, and 2.10 m from the centre of the slab. Relative to standard FWD testing, the geophones at radial distances r = 0.45 m and r = 1.80 m are missing in the case of T-shaped FWD testing, because they are needed right and left of the centre of the slab.
Figure 4. Best correlation between the Lateral Asymmetry Index according to Equation (Equation1
(1)
(1) ), related to T-shaped FWD testing, and the effective asymmetry index
according to Equation (Equation2
(2)
(2) ), related to multi-directional FWD testing: the deflections
,
, and
used to evaluate LASIX were measured at a distance c = 1.20 m from the centre of impact; each symbol corresponds to one of the 10 tested slabs; the horizontal and vertical black lines represent the threshold values of
and
distinguishing mild from strong asymmetries.

The associated loss of information regarding deflections measured along the driving direction is assessed as follows. Splines are used for interpolation between the deflections measured along the driving direction during T-shaped testing, see the blue lines and the black circles in and . They are evaluated at the positions of the removed geophones (i.e. at r = 0.45 m and r = 1.80 m, respectively). The resulting spline-interpolated values are compared with deflections measured during multi-directional testing at these positions, see the red stars in and . The relative error between spline-interpolated (index int) and measured (index m) values, , is smaller than 4% for all 10 tested slabs and at both positions from which geophones were removed, see . Thus, the loss of information resulting from the re-arrangement of two geophones from the driving direction to the lateral positions is tolerable for engineering purposes.
Figure 5. Deflections measured by geophones 3 and 8 (red stars, r = 450 mm and 1800 mm, respectively), and spline (blue line) interpolating between the rest of deflections measured in the driving direction (black circles), for slabs (a) A1-33354, (b) A1-33360, (c) A1-33868, (d) A1-33873, (e) A1-33874 .

Figure 6. Deflections measured by geophones 3 and 8 (red stars, r = 450 mm and 1800 mm, respectively), and spline (blue line) interpolating between the rest of deflections measured in the driving direction (black circles), for slabs (a) A2-47543, (b) A2-50000, (c) A2-51995, (d) A2-54003, (e) A2-54440.

Table 9. Values of the mean deflections measured () by the geophones at radial distances of r = 0.45 m and r = 1.80 m from the centre of the slab, and values of the deflections (
), at the same locations, obtained from the spline interpolating between the measurements of the rest of the geophones along the driving direction, for all 10 slabs, as well as the relative error between them.
3. Discussion
Central FWD tests with the developed T-shaped arrangement of geophones, and their evaluation by means of quantification of LASIX according to Equation (Equation1(1)
(1) ), was shown to provide a reliable assessment of asymmetric structural behaviour of rigid pavements. The following discussion deals with the question of where do such asymmetries come from, and with implications for the back-calculation of subgrade properties.
3.1. Reasons for asymmetric slab behaviour
Values of the effective asymmetry index of the four new slabs range from 2.73% to 3.87%, see . The corresponding mean value amounts to
. Thus, even new slabs exhibit asymmetric structural behaviour when subjected to central FWD testing.
The reason for an asymmetric behaviour in new slabs can be explained by their finite size as well as slab-to-slab interaction through dowels and tie bars (it is unlikely that a non-uniform distribution of subgrade stiffness is responsible for asymmetric behaviour of new slabs, because methods such as the dynamic compaction control are used during construction to ensure that all subgrade layers of pavement structure have uniform properties). The dense arrangement of dowels (typical spacing: 25 to 30 cm) render slab-to-slab load transfer in driving direction effective. Dowel-connected new slabs thus almost behave as if they were continuous in driving direction. The less dense arrangement of tie bars (typical spacing: 150 cm) renders the load transfer in lateral direction less effective. In addition, many slabs are connected by means of tie bars to a neighbour on one side, while the opposite lateral edge is free. Differences in boundary conditions of the edges of the slabs, together with their rectangular (rather than quadratic) shape, render their behaviour asymmetric already right after construction. The deviations from point symmetry are quantified through .
Values of of the six old slabs range from 4.29% to 9.67%, see . The corresponding mean value amounts to
. This underlines that the old slabs showed, on average, twice as much asymmetric structural behaviour than the new slabs. Recurrent loading over many years is responsible for the increase of the asymmetries. Notably, traffic loads are eccentric in the standard case that tire tracks are asymmetrically arranged relative to the N-S-axis running through the centre of the slabs, see . The corresponding asymmetric fatigue loading results in a directional deterioration of the pavement structure. This yields increasing deviations from point-symmetric behaviour in central FWD testing and, therefore, increasing values of
. As regards ‘eccentric’ traffic loads, it is noteworthy that it is currently ensured by design that the tire tracks run as far away as possible from the free edges of the slab (those facing the shoulder). As a consequence, edge stresses are minimised and the fatigue life of the pavement is extended. In addition, some design methods include the option of installing a tied shoulder beyond the traffic lanes, with the aim of either improving service life or reducing the required slab thickness, see e.g. (Packard, Citation1984, Packard and Tayabji, Citation1985).
Imperfect positioning of the falling weight also contributes to asymmetric structural behaviour. An eccentric positioning in driving direction has a smaller influence than the same eccentricity in lateral direction, because of the following two reasons:
(1) | Eccentricities of the falling weight are to be related to the size of the tested slab. The lateral width of slabs is smaller than their length. Thus, the same eccentricity in both directions leads to a larger effective imperfection in lateral direction. Similarly, if different slabs are tested with the same unintentional lateral eccentricity, its influence will be the larger the smaller the lateral width of the tested slab. | ||||
(2) | If dowel-connected slabs almost behave as if they were continuous in driving direction, eccentricities in driving direction will not result in significant asymmetries. Lateral eccentricities, in turn, must be expected to particularly increase asymmetries of slabs which are connected by means of tie bars to a neighbour on one side, while the opposite lateral edge is free. |
Figure 7. Top view onto a slab subjected to eccentric long-term loading resulting from tire tracks which are asymmetrically arranged relative to the N-S-axis running through the centre of the slab.

All described FWD tests on new plates were carried out with small eccentricities of the FWD-device from the centre of the slab, amounting to a few single centimetres. The resulting asymmetries were small enough that allowed for the correct classification into new and old slabs. This underlines that asymmetries arising from unintentional eccentric FWD testing are implicitly considered in the threshold value of
, distinguishing newly built from directionally degraded pavement structures.
3.2. Back-calculation of subgrade properties based on deflections known from FWD testing
Surface deflections measured during FWD testing are functions of the loading exerted by the falling weight and of the properties of the pavement structure. Thus, it is conceptually possible to back-calculate properties of the pavement structure from known surface loading and deflections. To the best of the authors' knowledge all currently available back-calculation approaches assume a uniform distribution of subgrade stiffness and, therefore, a symmetric behaviour of pavement structures. This assumption is challenged by the asymmetric structural behaviour found by means of multi-directional FWD testing of old slabs. In the following, ‘dense liquid’ models as well as the corresponding AREA and Best Fit methods will be briefly summarised. This provides the basis for subsequent correlation of LASIX with the directional variation of the deflection basin parameter AREA7, see Section 3.3.
The ‘dense-liquid’ model (AASHTO, Citation2008) idealises rigid pavements as infinite thin elastic plates on a uniform Winkler foundation (Winkler, Citation1867). There are two different back-calculation approaches: the AREA method and the Best Fit method. Both of them are based on closed-form solutions, which can be traced back to (Westergaard, Citation1926). Back-calculation concerns identification of the radius of relative stiffness, , from surface deflections measured during FWD testing.
is equal to
, where D denotes the bending stiffness of the plate and k the modulus of subgrade reaction of the Winkler foundation. Formulae for the final transition from
to the modulus of subgrade reaction were proposed, see e.g. (Darter et al. Citation1995, Hall et al., Citation1997). They have become part of the mechanistic-empirical design and evaluation of rigid pavements (AASHTO, Citation2008, Smith et al., Citation2017).
The AREA method is based on the AREA parameter. It was originally introduced as the area (hence the name) under the graph showing surface deflections of the pavement structure over the radial distance from the falling weight (Hoffman and Thompson, Citation1980). In order to account for different amplitudes of the falling weight, which yield qualitatively similar but quantitatively different surface deflections, the latter were normalised with respect to their maximum value, , measured at the position of the falling weight (Hoffman and Thompson, Citation1980). Therefore, the normalised version of the AREA parameter has the physical dimension of a length rather than an area. As for a configuration of four geophones with a uniform spacing equal to
, it reads as
(4)
(4) where
refers to the deflection measured by the geophone at the radial distance of
. The last expression in Equation (Equation4
(4)
(4) ) refers to numerical integration using the trapezoidal rule. Inspired by a dimensionless representation of Westergaard's solution by Losberg (Citation1960), Ioannides (Citation1990) used dimensional analysis for the derivation of a relation between the AREA parameter according to Equation (Equation4
(4)
(4) ) and the radius of relative stiffness, see in (Ioannides, Citation1990). Other sensor configurations were studied e.g. by Hall and Mohseni (Citation1991). The method that is based on four measured deflections is referred to as ‘AREA4’, the one based on seven measured deflections as ‘AREA7’. Both of them are used for rigid pavements (Khazanovich et al., Citation2001).
The Best Fit method is also based on a dimensionless representation of Westergaard's solution by Losberg (Citation1960). A closed-form solution for surface deflections, which contains Kelvin Bessel functions, is used for fitting of measured deflections (Ioannides, Citation1990). The method that is based on four measured deflections is referred to as ‘Best Fit 4’, the one based on seven measured deflections as ‘Best Fit 7’ (Khazanovich et al., Citation2001).
Depending on the number of deflection measurements used for the AREA method and the Best Fit method, respectively, the two methods deliver slightly different moduli of subgrade reaction (Khazanovich et al., Citation2001). Multi-layered elastic simulations performed with the DIPLOMAT program (Khazanovich, Citation1994, Khazanovich and Ioannides, Citation1995) were the basis to recommend the ‘Best Fit 4’ method (using deflections measured in radial distances of 0, 305, 610, and 914 mm) for rigid pavements (Khazanovich et al., Citation2001), see also (Hall et al., Citation1997). Still, it is noteworthy that the ‘Best Fit 4’ method and the ‘AREA7’ method (integrating the deflection basin up to a radial distance of 1524 mm) are practically equivalent. Both methods deliver virtually the same back-calculated values of the modulus of subgrade reaction, see and in (Khazanovich et al., Citation2001). This provides the motivation to relate LASIX to directional variations of the deflection basin parameter AREA7.
3.3. Relation between LASIX and the coefficient of directional variation of AREA7 (‘COVAREA7’)
Standard FWD tests are nowadays evaluated by means of methods that are based on the assumption of an infinite plate, uniform subgrade stiffness, and, therefore, point-symmetric slab behaviour. However, multi-directional FWD testing underlined that rigid pavements which had been in service for a long time behave in a remarkable asymmetric fashion. Thus, back-analysing uniform slab properties from FWD tests could be questionable. In this context it is useful to categorise the asymmetric behaviour of slabs into ‘mild asymmetries’ and ‘strong asymmetries’. This is supported by LASIX, as will be demonstrated in the remainder of the present section.
The database shown in Appendix 1 contains the deflection measurements from 27 FWD tests performed on every one of the 10 tested slabs. For every slab, the following analysis is performed. The three (to six) deflections, measured by means of repeated testing in the same direction and at the same distance to the centre of the falling weight, are averaged. The deflection profile between the averaged deflections is approximated, in every direction, by means of a spline. It is integrated from the centre of the falling weight to a radial distance :
(5)
(5) This yields one value of the AREA7 parameter for every measurement direction, see .
Table 10. Values of AREA7 [mm] obtained from the average of the three (to six) FWD tests performed along each of the eight directions for every one of the 10 slabs.
For all slabs except the old slab A1-33874, the -value of which amounts to 4.29%, see , which is smaller than the corresponding threshold value 5.2%, see , the values of COVAREA7 from are correlated with the values of LASIX from , see . The quadratic correlation coefficient amounts to 94%.
Values of LASIX smaller than 8% refer to values of COVAREA7 smaller than 4.6%. Such coefficients of directional variation of the AREA7 parameter are representative for new slabs. They show only mild asymmetries resulting from their finite size and slab-to-slab interaction, while the stiffness of the subgrade can be expected to be uniform. Thus, it is realistic to identify a uniform modulus of subgrade reaction from deflections measured in driving direction, either by the Best Fit 4 method or the AREA7 method.
Figure 8. Correlation between the coefficient of variation of the direction-dependent AREA7 values, COVAREA7, quantified based on results from multi-directional FWD testing over eight directions, and the Lateral Asymmetry Index according to Equation (Equation1
(1)
(1) ), related to T-shaped FWD testing; each symbol corresponds to one of the 10 tested slabs; the horizontal and vertical black lines represent the threshold values of
and
distinguishing mild from strong asymmetries.

Table 11. Mean value, , standard deviation,
, and coefficient of variation, COVAREA7, of the eight direction-dependent values of the AREA7 parameter of each slab.
Values of LASIX larger than 8% refer, according to the red regression line in , to values of COVAREA7 larger than 4.6%. Such coefficients of directional variation of the AREA7 values are representative for old slabs which show asymmetries because of directional degradation of the pavement structure, resulting from eccentric traffic loads. Whether or not the identification of uniform pavement properties, either by the Best Fit 4 method or the AREA7 method, is still realistic will be analysed in Section 3.4.
The exposure situation explains why of the old slab A1-33874 amounts to 3.33%, see , which is smaller than the corresponding threshold value 4.6%. This slab was part of an emergency lane, see . Therefore, it was subjected to traffic loading only indirectly, namely, because of load transfer via tie bars connecting the tested slab to its neighbour which was part of the first lane and, therefore, directly exposed to traffic loads.
3.4. Variation of the modulus of subgrade reaction back-calculated from eight direction-specific values of AREA7 per slab
In the AREA method, the ‘dense-liquid’ model is used to back-calculate a uniform modulus of subgrade reaction from deflections measured in the driving direction. This model idealises the pavement structure as a plate (finite thickness, but infinite in-plane dimensions) resting on a Winkler foundation. Assuming that deflections measured in the driving direction are axisymmetric with respect to the axis of impact of the falling weight, the AREA parameter is translated into the radius of relative stiffness (Ioannides et al., Citation1989, Ioannides, Citation1990):
(6)
(6) where
,
,
, and
are coefficients that depend on the specific AREA-parameter used, see (Hall et al., Citation1997). The radius of relative stiffness, in turn, is equal to the fourth root of the bending stiffness of the plate, D, divided by the modulus of subgrade reaction, k, Westergaard (Citation1926):
(7)
(7) The values of D and k are usually optimised such that the model-simulated deflection basin reproduces the measured deflections is the best-possible fashion (Hall et al., Citation1997, Khazanovich et al., Citation2001). Therefore, D is not necessarily equal to the bending stiffness of the concrete slab.
If measured defections are direction-dependent (= asymmetric), the assumption of a point-symmetric deflection basin is only useful, provided that direction-dependent values of the AREA parameter are translated, by means of Equations (Equation6(6)
(6) ) and (Equation7
(7)
(7) ), into virtually the same value of k. Whether or not this is the case, will be checked in the following.
For every slab, the following two-step procedure is performed. Step 1: The eight direction-dependent values of AREA7, see , are translated by means of Equation (Equation6(6)
(6) ) into eight corresponding values of
. Given that the AREA7-parameter is expressed in millimetres, the corresponding values of the empirical ξ-constants read as
,
,
,
, see (Hall et al., Citation1997) for details. With these values, Equation (Equation6
(6)
(6) ) yields values of
in millimetres. Step 2: The eight direction-dependent values of
are translated by means of Equation (Equation7
(7)
(7) ) into eight corresponding values of k. Thereby, the plate stiffness D is set equal to
which denotes the bending stiffness of the concrete slabs (other choices for D will be discussed at the end of the Section):
(8)
(8) where
denotes the modulus of elasticity of concrete,
the thickness of the slab, and
Poisson's ratio of concrete. Herein, these quantities are equal to 36500 MPa, 0.22 m, and 0.2, respectively, see also (Díaz Flores et al., Citation2021). Inserting these values into Equation (Equation8
(8)
(8) ) yields
(9)
(9) An expression for corresponding values of k is obtained by solving Equation (Equation7
(7)
(7) ) for k:
(10)
(10) This completes Step 2.
Applying the procedure described in the preceding paragraph to eight direction-dependent values of AREA7 per slab, allows for computing eight k-values per slab. They are the basis for computing slab-specific mean values of k, denoted as , as well as corresponding standard deviations,
, and coefficients of variation,
, see . The 10 slab-specific values of
are finally correlated with the corresponding values of COVAREA7 from , see .
Figure 9. Correlation between the coefficient of variation of eight values of the modulus of subgrade reaction, back-calculated from eight direction-dependent AREA7 values per slab, see in , and COVAREA7, see ; each symbol corresponds to one of the 10 tested slabs; the horizontal and vertical black lines represent threshold values separating mild from strong asymmetries.

Table 12. Mean value, , standard deviation,
, and coefficient of variation,
, of the eight direction-dependent values of the modulus of subgrade reaction k of each slab.
For pavement structures exhibiting mild asymmetries, as expressed by COVAREA7-values smaller than 4.6%, values of smaller than 35% are found. In such cases, the assumptions of a point-symmetric deflection basin and a uniform modulus of subgrade reaction appear to be useful for engineering purposes. For pavement structures exhibiting strong asymmetries, as expressed by COVAREA7-values larger than 4.6%, values of
larger than 35% are found. In such cases, the assumptions of a point-symmetric deflection basin and uniform pavement properties are questionable.
Finally, it is shown that the values of listed in and illustrated in are independent of the specific choice for the plate stiffness D in Equation (Equation7
(7)
(7) ). Above, the specific choice
was made. This provides the motivation to multiply
by a slab-specific scaling factor
. In order to analyse the corresponding implications on k, both sides of Equation (Equation10
(10)
(10) ) are multiplied by
:
(11)
(11) Equation (Equation11
(11)
(11) ) underlines that scaling of
by a factor
leads to k-values scaled by the same factor
. Scaling of the eight k-values per slab by a factor of
, in turn, leads to mean values
and standard deviations
scaled by the same factor
. In other words, both the
-values and
-values in are to be multiplied by
. This underlines that the coefficient of variation,
, is independent of
. It is concluded that the last column in and the ordinate values in remain the same, even if D is optimised in order to reproduce the measured deflections (rather than setting it equal to
).
3.5. Limitations and future outlook
The here-analysed FWD experiments were carried out on slabs made from normal concrete, with a thickness of 0.22 m, lengths ranging from 4.50 m to 5.60 m, widths from 3.10 m to 4.20 m, and length-to-width ratios from 1.12 to 1.81. The optimal value of c and the threshold values of LASIX, , COVAREA7, and CV(k) refer to slabs with properties listed above. Otherwise, these values are questionable. In the case of bonded white-toppings featuring square slabs 1.80 m long and wide, for instance, even a new radial distance c of the lateral geophones from the centre of the slabs would need to be optimised.
All non-standard FWD tests performed so far included multi-directional measurements of surface deflections. A prototype for FWD testing with a T-shaped arrangement of the geophones is in its design phase. This testing device will reduce the in-situ efforts to those known from standard FWD testing: piloting the FWD trailer to the slab of interest, lowering the impact transducer and the geophones onto the surface of the slab, lifting and dropping the falling weight while recording the surface deflections, uplifting the impact transducer and the geophones, and piloting the FWD trailer to the next slab of interest.
4. Conclusions
An optimal T-shaped arrangement of nine geophones was developed: one at the centre of impact (= centre of the slab), six along the driving direction, one right and one left of the centre. The following conclusions are drawn:
As for central FWD testing with a T-shaped arrangement of geophones, the optimal distance of the lateral geophones from the centre of impact is equal to
.
It is possible to integrate the proposed arrangement (or an alternative similar to it) into trailers complying with the maximum allowed widths of vehicles, e.g.
in the USA,
in China, and
in Europe.
This renders highly automated and, therefore, rapid FWD testing feasible, with on-site efforts equal to those known from standard FWD testing.
State-of-the-art evaluation of deflections measured in driving direction remains possible, as long as a suitable amount of geophones remain arranged along the driving direction (here: seven including the one at the centre of the falling weight).
The ‘Lateral Asymmetry Index’ (LASIX) is a deflection-basin-parameter customised for T-shaped FWD testing. It enables the quantification of asymmetric slab behaviour.
Values of LASIX smaller than 8% refer to coefficients of directional variation of AREA7 smaller than 4.6%. For slabs presenting geometric and stiffness properties within the intervals studied, such values are representative for new slabs which show only mild asymmetries. The latter result from their finite size and slab-to-slab interaction, while the stiffness of the subgrade can be expected to be uniform. Thus, it is realistic to identify a uniform modulus of subgrade reaction, either by the Best Fit 4 method or the AREA7 method.
Values of LASIX larger than 8% refer to coefficients of directional variation of AREA7 larger than 4.6%. For slabs presenting geometric and stiffness properties within the intervals studied, such values are representative for old slabs with directional degradation of the pavement structure, resulting from eccentric traffic loads. Thus, it is questionable to identify uniform properties of the pavement structure.
In the future, it will be interesting
to integrate central FWD tests with a T-shaped arrangement of geophones into the monitoring strategy of pavement slabs made of concrete, i.e. to perform such tests regularly on specific slabs in order to study the evolution of LASIX as a function of the age of the slab and of the service loads to which it is exposed (traffic and hygro-thermal loads), and
to perform such tests on slabs with different sizes and stiffness properties, in order to widen their range of applicability.
List of symbols
Table
List of abbreviations
Acknowledgments
The help of Pia Mandahus (TU Wien, Vienna, Austria), Marek Milcevic, Roman Oblak and Harald Aigner (Nievelt Labor GmbH, Höbersdorf, Austria) as well as interesting discussions with Wolfgang Kluger-Eigl (TU Wien), Martin Peyerl and Gerald Maier (Smart Minerals GmbH, Vienna, Austria) as well as Reinhard Lohmann-Pichler and Karl Gragger (ASFINAG Bau Management GmbH) are gratefully acknowledged.
Disclosure statement
No potential conflict of interest was reported by the author(s).
Additional information
Funding
References
- AASHTO, 2008. Mechanistic-Empirical Pavement Design Guide: A Manual of Practice. In: American Association of State Highway and Transportation Officials. Washington, D.C.
- Bay, J., Stokoe II, K., and Jackson, J., 1995. Development and preliminary investigation of rolling dynamic deflectometer. Transportation Research Record, 1473, 43–54.
- Briggs, R.C., et al. 2000. A comparison of the rolling weight deflectometer with the falling weight deflectometer. In: Nondestructive Testing of Pavements and Backcalculation of Moduli: Third Volume. ASTM STP 1375.
- Coni, M., et al. 2021. Fast falling weight deflectometer method for condition assessment of RC bridges. Applied Sciences, 11 (4), 1743.
- Darter, M.I., Hall, K.T., and Kuo, C.M., 1995. Support under Portland cement concrete pavements. National Cooperative Highway Research Program, Project 1-30 FY'93.
- Díaz Flores, R., et al. 2021. Multi-directional falling weight deflectometer (FWD) testing and quantification of the effective modulus of subgrade reaction for concrete roads. International Journal of Pavement Engineering, 1–19. doi:10.1080/10298436.2021.2006651.
- Fleming, P.R., Frost, M.W., and Lambert, J.P., 2007. Review of lightweight deflectometer for routine in situ assessment of pavement material stiffness. Transportation Research Record, 2004 (1), 80–87.
- Hall, K.T., and Mohseni, A., 1991. Backcalculation of asphalt concrete-overlaid portland cement concrete pavement layer moduli. Transportation Research Record, 1293, 112–123.
- Hall, K.T., et al. 1997. LTPP data analysis. phase I: Validation of guidelines for k-value selection and concrete pavement performance prediction. Federal Highway Administration, No. FHWA-RD-96-198.
- Hoffman, M.S., and Thompson, M., 1980. Mechanistic interpretation of nondestructive pavement testing deflections. University of Illinois at Urbana-Champaign, FHWA/IL/UI-190.
- Ioannides, A.M., 1990. Dimensional analysis in NDT rigid pavement evaluation. Journal of Transportation Engineering, 116 (1), 23–36.
- Ioannides, A., Barenberg, E., and Lary, J., 1989. Interpretation of falling weight deflectometer results using principles of dimensional analysis. In: Proceedings, 4th International Conference on Concrete Pavement Design and Rehabilitation, Purdue University. 231–247.
- Ioannides, A.M., and Khazanovich, L., 1998. Nonlinear temperature effects on multilayered concrete pavements. Journal of Transportation Engineering, 124 (2), 128–136.
- Khazanovich, L., 1994. Structural analysis of multi-layered concrete pavement systems. Thesis (PhD). University of Illinois at Urbana-Champaign.
- Khazanovich, L., and Ioannides, A.M., 1995. DIPLOMAT: analysis program for bituminous and concrete pavements. Transportation Research Record, 1482, 52–60.
- Khazanovich, L., Tayabji, S.D., and Darter, M.I., 2001. Backcalculation of layer parameters for performance for LTPP test sections, volume I: Slab on elastic solid and slab on dense-liquid foundation analysis of rigid pavements. United States. Federal Highway Administration. Office of Engineering.
- Losberg, A., 1960. Structurally reinforced concrete pavements. (Vol. 29), Akademiforlaget Gumperts.
- Nazzal, M.D., et al. 2007. Evaluating the light falling weight deflectometer device for in situ measurement of elastic modulus of pavement layers. Transportation Research Record, 2016 (1), 13–22.
- Packard, R.G., 1984. Thickness design for concrete highway and street pavements. Portland Cement Association.
- Packard, R.G., and Tayabji, S.D., 1985. New PCA thickness design procedure for concrete highway and street pavements. In: Third International Conference on Concrete Pavement Design and Rehabilitation, West Lafayette. Purdue University.
- Pratelli, C., et al. 2018. Preliminary in-situ evaluation of an innovative, semi-flexible pavement wearing course mixture using fast falling weight deflectometer. Materials, 11 (4), 611.
- Smith, K.D., et al. 2017. Using Falling Weight Deflectometer Data with Mechanistic-Empirical Design and Analysis, Volume I. Federal Highway Administration, United States, FHWA-HRT-16-009.
- Westergaard, H.M., 1926. Stresses in concrete pavements computed by theoretical analysis. Public roads.
- Winkler, E., 1867. Die Lehre von der Elasticität und Festigkeit mit besonderer Rücksicht auf ihre Anwendung in der Technik [lessons on elasticity and strength of materials with special consideration of their application in technology]. H. Dominicus, Prague.
Appendix
Appendix 1. Results of multi-directional FWD testing of all slabs
Table A1. Maximum deflections measured during the 27 FWD tests performed on the old slab A1-33354 [mm].
Table A2. Maximum deflections measured during the 27 FWD tests performed on the new slab A1-33360 [mm].
Table A3. Maximum deflections measured during the 27 FWD tests performed on the old slab A1-33868 [mm].
Table A4. Maximum deflections measured during the 27 FWD tests performed on the new slab A1-33873 [mm].
Table A5. Maximum deflections measured during the 27 FWD tests performed on the old slab A1-33874 [mm].
Table A6. Maximum deflections measured during the 27 FWD tests performed on the old slab A2-47543 [mm].
Table A7. Maximum deflections measured during the 27 FWD tests performed on the old slab A2-50000 [mm].
Table A8. Maximum deflections measured during the 27 FWD tests performed on the new slab A2-51995 [mm].
Table A9. Maximum deflections measured during the 27 FWD tests performed on the old slab A2-54003 [mm].
Table A10. Maximum deflections measured during the 27 FWD tests performed on the new slab A2-54440 [mm].