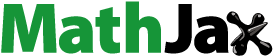
Abstract
Intratympanic (IT) therapies have been explored to address several side effects that could be caused by systemic administration of steroids to treat inner ear diseases. For effective drug delivery to the inner ear, an IT delivery system was developed using poly(lactic-co-glycolic acid) (PLGA) nanoparticles (NPs) and thermosensitive gels to maintain sustained release. Dexamethasone (DEX) was used as a model drug. The size and zeta potential of PLGA NPs and the gelation time of the thermosensitive gel were measured. In vitro drug release was studied using a Franz diffusion cell. Cytotoxicity of the formulations was investigated using SK-MEL-31 cells. Inflammatory responses were evaluated by histological observation of spiral ganglion cells and stria vascularis in the mouse cochlea 24 h after IT administration. In addition, the biodistribution of the formulations in mouse ears was observed by fluorescence imaging using coumarin-6. DEX-NPs showed a particle size of 150.0 ± 3.2 nm in diameter and a zeta potential of −18.7 ± 0.6. The DEX-NP-gel showed a gelation time of approximately 64 s at 37 °C and presented a similar release profile and cytotoxicity as that for DEX-NP. Furthermore, no significant inflammatory response was observed after IT administration. Fluorescence imaging results suggested that DEX-NP-gel sustained release compared to the other formulations. In conclusion, the PLGA NP-loaded thermosensitive gel may be a potential drug delivery system for the inner ear.
1. Introduction
Hearing loss caused by inner ear disease is increasing because of prolonged exposure to noise, increased life expectancy, and the use of medicines such as anticancer drugs (Kim, Citation2017). Therefore, there is a growing interest in drug delivery to the inner ear. Glucocorticoid drugs, such as dexamethasone (DEX), are considered potential otoprotective drugs with anti-inflammatory effects (Van De Water et al., Citation2010). However, systemic drug administration usually results in only a small amount of drug that reaches the inner ear and a risk of side effects. The benefits of inner ear drug delivery are that they do not cross the blood–labyrinth barrier, avoid first-pass metabolism from systemic administration, maintain high drug concentrations in the inner ear, and reduce the total amount of administered drug (Juhn et al., Citation1982; Mäder et al., Citation2018).
Intratympanic (IT) administration, a process in which drugs are injected into the middle ear through the eardrum to deliver drugs into the inner ear, has been studied for many years (Ersner et al., Citation1951; Schuknecht, Citation1956). Since the mid-1990s, local drug delivery to the ear has been used as a clinical treatment. If the drug is administered to the middle ear, it must pass through the round window membrane (RWM) to reach the inner ear (Nedzelski et al., Citation1993; Toth & Parnes, Citation1995). Therefore, the administered drug must remain in the middle ear for sufficient time and be in contact with the RWM. However, the drug administered to the middle ear is rapidly removed into the Eustachian tube through the flow of the mucosa (Salt & Plontke, Citation2018).
Over the past several years, biodegradable polymer nanoparticles (NPs) have attracted interest for drug delivery to the inner ear. As a carrier for local drug administration, NPs composed of poly(lactic-co-glycolic acid) (PLGA), approved by the Food and Drug Administration and the European Medicine Agency, have been considered an ideal carrier (Blasi, Citation2019; Elmowafy et al., Citation2019; Schoubben et al., Citation2019). Previous studies have demonstrated the application of rhodamine-containing PLGA NPs to the RWM, which were then transported to the perilymph in experimental animals (Tamura et al., Citation2005; Ge et al., Citation2007). Indeed, it was confirmed that PLGA NPs carried the drug through the RWM into the inner ear; however, the process can be difficult because of the loss of NP suspension that occurs through the Eustachian tube (Salt et al., Citation2011; Zhang et al., Citation2013; Cai et al., Citation2014).
Hydrogels have been evaluated in many studies and have been shown to retain drugs in the middle ear. Thermosensitive gels made of Poloxamer 407 (P407) and Poloxamer 188 (P188) that exist in the solution state at room temperature and in gel state at body temperature, have been actively studied (Buwalda et al., Citation2014). Poloxamers have been studied for topical drug delivery via many routes, such as the nasal, ocular, rectal, vaginal, and transdermal routes (Mao et al., Citation2016). Applications in inner ear drug delivery based on thermosensitive gels have also been studied. For effective inner ear drug delivery of gentamicin, chitosan glycerophosphate hydrogel and chitosanase were used for continuous and controlled inner ear drug delivery (Lajud et al., Citation2013). Another study demonstrated delayed release of drugs without histologic changes in the inner ear through PLGA NPs based on chitosan/glycerophosphate (Dai et al., Citation2018). This combination of NPs and hydrogels is currently considered as a promising approach that provides a synergistic effect to control drug delivery to the inner ear, in which hydrogels reduce Eustachian tube clearance and prolong the residence time in the middle ear, while NPs enhance the permeability of the drug through the RWM.
In this study, DEX-loaded PLGA NPs based on poloxamer hydrogels as inner ear drug delivery carriers were developed for sustained drug release. The NPs were characterized in terms of size, zeta potential, and encapsulation efficiency (EE). In addition, the gelation time of the hydrogels was determined. The in vitro release study predicted the release of the formulation after IT administration. The cytotoxicity of the formulations was assayed for cellular safety after reaching the inner ear cells. The in vivo toxicity and retention of the DEX-NP-gel formulations after administration were observed using histopathology and fluorescence imaging. We believe that the obtained results could show the superiority related to the effectiveness and safety of the PLGA-hydrogel system compared to that of PLGA NPs only.
2. Materials and methods
2.1. Materials
PLGA (50:50; lactide:glycolide, MW 38,000–54,000), polyvinyl alcohol (PVA, MW 30,000–70,000), tetrazolium dye 3-(4,5-di-methylthiazol-2-2-yl)-2,5-diphenyltetrazolium bromide (MTT), and coumarin-6 were purchased from Sigma-Aldrich (St. Louis, MO). Phosphate-buffered saline (PBS), fetal bovine serum (FBS), antibiotics (100 IU/mL penicillin G sodium and 100 µg/mL streptomycin sulfate), and minimum essential medium (MEM) were purchased from Hyclone (Logan, UT). Poloxamer 407 (P407) and Poloxamer 188 (P188) were purchased from BASF Laboratories (Wyandotte, MI). DEX was purchased from Tokyo Chemical Industry (Tokyo, Japan). SK-MEL-31 cells were purchased from the Korean Cell Line Bank (Seoul, Republic of Korea). All solvents were purchased from Samchun Chemical (Pyeongtaek, Republic of Korea).
2.2. Preparation of PLGA NPs and NP-loaded thermosensitive gels
DEX-loaded PLGA NPs were prepared using a modified nanoprecipitation method as listed in (Govender et al., Citation1999; Choi et al., Citation2014). First, PLGA and DEX were dissolved in 5 mL of organic solvents (acetone, dichloromethane (DCM), and a mixture of acetone and DCM) in an ultrasonic bath for 15 min. Coumarin-6 was loaded instead of DEX for fluorescence imaging studies. The solution was injected into 30 mL of 1% PVA at a flow rate of 20 mL/h using an autoinjector (KDS 200, KD Scientific Inc., Holliston, MA). During the injection, the mixtures were stirred at 500 rpm using a magnetic stirrer. The residual solvents were then removed for 24 h in a fume hood. The resultant NPs were collected by centrifugation for 30 min at 10,000×g and washed three times with distilled water. Finally, the NPs were dispersed in PBS (pH 7.4).
Table 1. Composition of PLGA nanoparticles.
After washing the prepared DEX-NPs three times, 10 mL of the prepared thermosensitive gel was added to the DEX-NPs. Thereafter, DEX-NPs were dispersed using a vortex mixer (KMC-1300V, Vision Scientific, Daejeon, Republic of Korea) and an ultrasonic bath to produce DEX-NP-gels.
2.3. Physicochemical characterization of PLGA NPs
2.3.1. Determination of particle size and zeta potentials
The particle size of the PLGA NPs was measured using a Zetasizer Nano S90 (Malvern Instruments, Malvern, UK) at room temperature (20–22 °C). The samples were diluted with distilled water to obtain a specific intensity, as recommended by the manufacturer. The zeta potential of the PLGA NPs was measured using a Zetasizer Nano Z (Malvern Instruments, Malvern, UK).
2.3.2. Determination of encapsulation efficiency
To determine the EE of the DEX-NPs, dispersed NPs were dissolved in 99% ethanol to disrupt their overall structure. The solution was then centrifuged for 5 min at 10,000×g to remove any impurities. The amount of DEX in the supernatant was analyzed by high-performance liquid chromatography (HPLC). The EE was calculated using the following equation (Byeon et al., Citation2019):
where WT is the weight of the total DEX used to prepare DEX-NPs and WL is the weight of the loaded DEX in the DEX-NPs.
2.3.3. HPLC determination of the DEX content of NPs
The concentration of DEX was determined using HPLC (Shimadzu, Kyoto, Japan). The mobile phase used was a mixture of acetonitrile and water (35:65, v/v). The flow rate was 1 mL/min, and separation was performed using a Capcell Pak C18 column (150 nm × 4.6 mm, 5 µm particle size, Shiseido, Tokyo, Japan) set at 40 °C. The injection volume of the samples was 20 µL, and the signal was detected at 254 nm using a UV/Vis detector (Shiseido, Tokyo, Japan). The retention time of the samples was approximately 4.1 min (Milojevic et al., Citation2002; Prakash et al., Citation2012).
2.3.4. Differential scanning calorimetry (DSC)
Thermal analysis of DEX, blank-NPs, DEX-NPs, and a physical mixture (PM) was performed using DSC (S-650, Scinco, Seoul, Republic of Korea). Blank-NPs and DEX-NPs were lyophilized using a freeze dryer (FDU-200, EYELA, Tokyo, Japan), and 2 mg samples were placed on aluminum pans for DSC analysis. The samples were heated from 25 °C to 300 °C, at a rate of 10 °C/min (Yoon et al., Citation2003).
2.3.5. Fourier transform infrared (FT-IR) spectrometry
To evaluate the chemical interactions, FT-IR spectroscopy was performed using a VERTEX 80v spectrometer (Bruker, Bremen, Germany). The samples used for FT-IR were the same as those used for the DSC. The wavelengths, set from 4000 cm−1 to 600 cm−1, were scanned 64 times (Altındal & Gümüşderelioğlu, Citation2016).
2.4. Preparation of thermosensitive gel
A poloxamer was used to prepare the thermosensitive gel. P188 and P407 were mixed in various ratios (). Thermosensitive gels were prepared according to the cold method (Schmolka, Citation1972). P188 and P407 were slowly mixed in PBS (pH 7.4) with stirring. After stirring for 2 h, the mixtures were stored in a refrigerator at 4 °C until the solution became clear.
Table 2. Composition and gelation time of poloxamer mixtures (n = 3).
2.5. Measurement of poloxamer gelation time
The gelation temperature was set to 38 °C corresponding to a study on the ear temperature of mice (Saegusa & Tabata, Citation2003). Briefly, 1 mL of the prepared liquid gel was placed in an Eppendorf tube and placed in a water bath (VS-1250W, Vision Scientific, Daejeon, Republic of Korea) set at 38 °C. Simultaneously, time was measured using a stopwatch while observing the fluidity of the gels. The gelation time was measured as the time for the fluidity of the gels in the water bath to disappear. The time measurement proceeded to 300 s.
2.6. In vitro drug release study
In vitro drug release was evaluated using a transdermal diffusion cell system (LOGAN Instruments, Franklin Township, NJ). DEX, DEX-NP, and DEX-NP-gel formulations (1 mL) were applied to the donor chamber of the Franz diffusion cell. The dialysis membrane (6–8 kDa) was fixed to the permeable layer. PBS solution (12 mL, pH 7.4) containing 20% (v/v %) polyethylene glycol 400 (PEG 400) and a magnetic bar was placed in the receptor chamber. Samples (0.5 mL) were collected at 1, 2, 4, 8, 12, and 24 h, and an equal amount of fresh PBS (20% PEG 400) was added. The temperature of the circulating water was 37 ± 0.5 °C and the stirring speed was 200 rpm. The amount of DEX in the samples was measured using HPLC.
2.7. Stability
To investigate the stability of the DEX-NPs, the changes in particle size, zeta potential, EE, and in vitro drug release were investigated for 8 weeks. Likewise, in vitro drug release was also evaluated for 8 weeks to investigate the stability of DEX-NP-gels. The samples were stored at 4 °C and the evaluation methods were the same as in Sections 2.3 and 2.8.
2.8. Cell viability
Cell viability was evaluated using MTT assay. Human melanocyte SK-MEL-31 cells were grown in MEM cell culture media containing 10% (v/v %) FBS and 1% (v/v %) antibiotics (100 IU/mL penicillin G sodium and 100 µg/mL streptomycin sulfate). Cells were maintained in an incubator (BB15 CO2 incubator, Thermo Scientific, Waltham, MA) at 37 °C in a humidified 5% CO2 atmosphere. The cells were seeded into 96-well plates at a density of 5 × 104 cells/well and subsequently incubated for 24 h. Medium in each well was replaced with various concentrations of DEX, DEX-NPs, and DEX-NP-gels containing the same concentrations of DEX dissolved in MEM. After 4 h of incubation with the formulations, the medium were replaced with fresh MEM without FBS and antibiotics. The plates were then incubated for 48 h. The cell viability was assayed using MTT solution in fresh media (5 mg/mL). Following incubation, the media in each well was removed, and 100 µL of MTT solution was added. After incubation for 3 h, the MTT solution was removed and 200 µL dimethyl sulfoxide was added to each well. Absorbance was measured using a microplate reader (Infinite M200 PRO, Tecan Trading AG, Männedorf, Switzerland) at 520 nm (Choi et al., Citation2014; Ahn et al., Citation2019).
2.9. Histological evaluation
Histological evaluation was performed using 8-week-olds male BALB/c mice (Samtako, Osan, Republic of Korea). The mice were acclimated for 1 week prior to the experiments. All procedures were approved by the Ethics Committee of Chungnam National University (protocol no. 201903A-CNU-33).
The formulations that were administered included a blank, DEX solution, DEX-NP, and DEX-NP-gel. The dose was 5 µL according to previous studies, and the concentration of DEX was adjusted to 4 mg/mL for each formulation (Hargunani et al., Citation2006). Intratympanic administration was performed using a micropipette tip, and the posture of the mice was fixed for 10 min after administration. Two days after formulation administration, the mice were euthanized, and the cochleae on the administered side were extracted. Tissues were fixed in 10% buffered formalin, and decalcified for four days in 10% EDTA. After fixation and decalcification, the temporal bone was embedded in paraffin and sectioned into 4-µm slices. Sections were stained with hematoxylin and eosin and subjected to histopathological analysis (Choi et al., Citation2013; Freitas et al., Citation2021).
2.10. In vivo fluorescence imaging study
In vivo fluorescence imaging was performed with imaging equipment (VISQUE® InVivo Smart, Vieworks, Anyang, Republic of Korea) using 8-week-old male BALB/C mice. Coumarin-6 was used to prepare a coumarin-6 solution (Cou), coumarin-6 encapsulated NPs formulation (Cou-NPs), and NPs-dispersed gel formulation (Cou-NP-gels). The coumarin-6 concentration was set to 1 mg/mL for each formulation. Intratympanic administration was performed in 5 µL using a micropipette. The posture of the mice was maintained for 10 min after administration. Imaging was performed using an iris 3.6, 1× zoom, focused on the head of the mice.
2.11. Biodistribution study
A biodistribution study was performed in the cochlea of 8-week-olds male BALB/C mice. Mice were randomly divided into four groups (three mice per group): control, DEX, DEX-NPs, and DEX-NP-gel. The administration was performed in the same way as for the histological evaluation. After 24 h, the mice were sacrificed, and the cochlea were harvested. The process of dissecting the mouse head to collect the cochleae was performed in accordance with previous studies (Keiler & Richter, Citation2001; Soken et al., Citation2013; Mülazımoğlu et al., Citation2017). The collected cochleae were weighed and placed in PBS (pH 7.4, 10 mg/mL). For sample preparation, cochlear fragments were homogenized using a homogenizer (Omni mixer homogenizer, Omni International, Kennesaw, GA). Homogenates (3 mL) were added to 1 mL of methanol for extraction and mixed for 5 min. The samples were placed in an oven to completely evaporate the solvent. The evaporated samples were mixed in the mobile phase (ACN:distilled water = 35:65, 0.1 mL) and sonicated for 30 min. After centrifugation at 12,000 rpm for 10 min, 20 µL of the supernatant was injected into the HPLC system.
2.12. Statistical analysis
Statistical analysis of the data was performed using ANOVA with Tukey’s post hoc test in SigmaPlot (version 12.0, Systat Software Inc., Chicago, IL). Results are expressed as mean ± standard deviation (S.D.). In all analyses, p<.05 (*) and p<.01 (**) were considered statistically significant.
3. Results and discussion
3.1. Characterization of PLGA NPs
Screening of PLGA NPs containing DEX was performed based on particle size, zeta potential, and EE. The screening composition is listed in , and the measurement results are listed in . Particle size is one of the most important parameters for determining the delivery of inner ear drugs. In a previous study, the most suitable particle size of PLGA NPs for drug delivery to the inner ear, which were able to pass through the RWM and high EE for inner ear drug delivery, was in the range of 100–200 nm (Cai et al., Citation2017). The organic solvents used in the screening process were DCM and acetone. When DCM was used as the organic solvent, EE tended to be low. Previous studies have also shown that the use of DCM in the PLGA particle preparation process results in a larger particle size than acetone alone (Hickey et al., Citation2002; Choi et al., Citation2014). Moreover, PLGA was fully dissolved in acetone, and drug solubility also increased in acetone (Kang et al., Citation2017). In addition, increasing the amount of DEX increased the size, whereas increasing the amount of PLGA tended to decrease the size. Finally, formulation N11 with a size of 150.0 ± 3.2 nm, zeta potential of ‒18.7 ± 0.5 mV, and an EE of 64.4 ± 2.0% was selected.
Table 3. Particle size, PDI, zeta potential, and entrapment efficiency of formulation of N1–N13.
3.2. DSC
DSC analysis was used to evaluate the physical state of the drug and its formulation and to determine its thermal properties. The DSC profiles of DEX, PM, blank NP, and DEX-NP are shown in . DEX showed a single exothermic peak at 261.74 °C. In addition, exothermic peaks at 53.15, 223.12, and 260.69 °C were observed in the PM containing DEX, PLGA, and PVA, respectively. According to the literature, the exothermic peak at 223.12 °C was PVA, and the peak at 53.25 °C was PLGA (Karim & Islam, Citation2011; Pool et al., Citation2012). In blank NPs, peaks were found at 52.81 °C and 223.85 °C, which belong to the peaks of PLGA and PVA, respectively . Furthermore, DEX-NP also showed peaks of PLGA and PVA in order at 53.08 °C and 222.58 °C. However, it should be noted that a small peak appeared at 261.63 °C, and it was determined that this was unwashed DEX that remained in the samples from preparation of the formulation. This result confirmed that DEX was well incorporated into PLGA NPs.
3.3. FT-IR spectrometry
FT-IR was used to investigate the interactions between DEX and the formulations. presents the FT-IR spectra of DEX, PM, blank-NP, and DEX-NP. Two characteristic absorption peaks are observed in the DEX spectrum. The peak at 1704.96 cm−1 overlapped with the stretching vibration absorption of C=O, and the peak at 1661.24 cm−1 corresponded to C=C. The C=O peak at 1754.87 cm−1 was observed in the spectrum of blank NP (PLGA NP). In addition, blank NP showed a C–O methyl group stretching vibration at 1090.30 cm−1. Similar bands have been studied for DEX and PLGA NPs (Chiang et al., Citation2012; Khalil et al., Citation2013; Ghorbani et al., Citation2016). The stretching vibration absorption peaks of DEX and blank-NP did not change in the PM. In DEX-NP, absorption peaks appeared at 1754.47 cm−1 and 1090.36 cm−1, which were similar to those of blank-NP; however, no absorption peaks appeared for DEX. Therefore, the FT-IR spectra confirmed that the DEX-NPs were completely enclosed in the particles.
3.4. Gelation time of poloxamer thermosensitive gel
The gelation time of the poloxamer thermosensitive gel was the time required for the formulation to harden to the middle ear after administration. The formulations should remain in the contact with the RWM as they could be removed if they remained in the liquid state for an extended period of time in the middle ear. The gel requires sufficient time for gelation which is approximately 60 s to enable direct delivery of the sample into the middle ear cavity by using a fine syringe and forming a gel when exposed to body temperature. The results are presented in . Gels composed of P188 alone had a gelation time of more than 300 s (G1 − G3), which cannot undergo gelation at 37 °C. In a previous study, the gelation time of P407 at 37 °C decreased with increasing amounts of P407 (Engleder et al., Citation2014). There was a similar trend from G4 to G6, but it was not reasonable for the target time of 60 s. P188 was used in combination with P407 to control the gelation time of the poloxamer gel. The addition of P188 increased the hydrophilicity of P407, leading to an increase in the sol–gel transition temperature (Ban et al., Citation2017; Russo & Villa, Citation2019). The gelation time of the hydrogel containing both P188 and P407 was evaluated in six formulations from G7 to G12. From the results, formulation G8 was selected as a thermosensitive gel with a proper composition with a gelation time of 64.00 ± 7.21 s.
3.5. In vitro drug release study
The results of the in vitro drug release experiments are shown in . In this experiment, the dialysis membrane replaced the role of RWM, and the medium selected was PBS containing 20% PEG400 to ensure sink conditions. Thus, it was possible to predict the amount of durg in formulations that could pass through the RWM and into the inner ear. The DEX solution showed an initial burst of approximately 60% at 1 h, while DEX-NPs and DEX-NP-gel showed only about 20% release after 1 h. After 24 h, the released percentages of DEX, DEX-NPs, and DEX-NP-gels were 73.50 ± 1.42, 49.20 ± 2.94, and 46.27 ± 0.04%, respectively. If in vitro release was performed to 48 h, there was a clear difference between the drug and its formulation. However, other in vitro release studies have been investigated for up to 24 h if different releases between formulations can be observed (Gu & Zheng, Citation2015; Chibas et al., Citation2019). DEX showed the fastest release amount, whereas DEX-NPs and DEX-NP-gels showed relatively similar release percentages. The sustained release of the drug was attributed to both PLGA and gels. The entrapment of DEX into PLGA NPs decelerated drug release, in agreement with a previous study (Kim & Martin, Citation2006), because the drug release rate was controlled by diffusion and degradation of the polymer matrix (Han et al., Citation2016). In addition, the incorporation of DEX-loaded PLGA NPs into the hydrogel also extended the drug release of DEX according to the study by Dai et al. (Citation2018). In the current study, there was a small difference in the drug release profiles of DEX-NP and DEX-NP-gel within the first 8 h of testing, mainly because of the obstructive effect of the gel matrix (Ay Şenyiğit et al., Citation2015).
3.6. Stability
The particle size, polydispersity index (PDI), zeta potential, and EE of the N11 formulation, and in vitro release from the N11 and G8 formulations were determined for 8 weeks to evaluate their stability. As shown in and Figure S1, after 8 weeks, the particle size of N11 changed from 150.0 ± 3.2 nm to 150.6 ± 1.9 nm, PDI changed from 0.077 ± 0.056 to 0.101 ± 0.033, zeta potential changed from ‒18.7 ± 0.6 mV to ‒18.7 ± 0.5 mV, and EE changed from 64.4 ± 2.0% to 63.0 ± 5.7%. In addition, the release patterns of DEX-NP and DEX-NP-gel were similar for the initial and 8 weeks in vitro release studies. Therefore, no significant changes were observed for 8 weeks, and the formulations were considered to be stable. Our product is intended for storage in the refrigerator, so the chosen storage condition was 4 °C, as referenced from the study of Lemoine et al. (Citation1996). In terms of the duration of time for stability testing, ideally it is better with a longer stability time, and it is expected to be up to 12 months according to ICH guidelines. However, the duration of storage is not strictly followed in studies related to nanomedicines (Muthu & Feng, Citation2009), which can be seven days in the study by Shkodra-Pula et al. (Citation2019) or 2 weeks in the research of Swider et al. (Citation2019).
Table 4. Stability of formulation N11.
3.7. Cell viability
Cell viability was measured at various concentrations of DEX (0–1000 µg/mL). To evaluate the toxicity of the formulations, a human melanocyte cell line (SK-MEL-31 cells) was used, as it is similar to that of the cells that constitute the cochlea. Melanocyte deficiency can be observed in patients with Waardenburg’s syndrome. Waardenburg’s syndrome is a clinically heterogeneous disease resulting from mutations in different genes, and sensorineural hearing loss is a characteristic feature of this syndrome (Laurell et al., Citation2007). Although the association between loss of melanin and hearing loss due to damage to melanocytes has rarely been investigated, it is known that various drugs form complexes with melanin, which affects their activity (Ono & Tanaka, Citation2003; Wrześniok et al., Citation2005). The cell viability results are shown in . The DEX solution showed increased toxicity with increasing concentrations. The toxicities of DEX-NP and DEX-NP-gel were similar. As both formulations were toxic at 1000 µg/mL, it was necessary to confirm that toxicity was observed even after IT administration in animal studies.
3.8. Histological evaluation
Histological evaluation was conducted to demonstrate the safety of the drug delivery system. The influence of DEX solution, DEX-NP, and DEX-NP-gel on the morphological structure of the inner ear (spiral ganglion cells, stria vascularis) was evaluated at day 2 after application and the results are shown in . After IT administration of the prepared formulations, no significant changes in cytotoxicity or inflammatory responses were observed in the spiral ganglion cells and stria vascularis in the cochlea of mice. In the cell viability study, both DEX-NP and DEX-NP-gel showed cytotoxicity at 1 mg/mL, but no toxicity was observed at 4 mg/mL in vivo. The use of PLGA and poloxamer for preparation of drug delivery systems containing PLGA NPs embedded in poloxamer gel matrix were biocompatible for inner ear therapy, which was in line with the previous studies investigating PLGA NPs or poloxamer hydrogel alone for IT drug delivery (Wang et al., Citation2009; Cai et al., Citation2017). The phenomenon of DEX-NPs and DEX-NP-gels, which appeared toxic at 1 mg/mL of the drug in cell culture, was not structurally toxic at a concentration of 4 mg/mL in mice, which could be considered as the protective capacity of the RWM because the formulation must pass through the RWM to reach the inner ear compartment.
3.9. In vivo fluorescence imaging study
In vivo fluorescence images were obtained at 6, 24, and 48 h after IT administration of coumarin-6 formulations as shown in . When Cou-6 was administered, the solution was quickly lost through the Eustachian tube after 6 h. Cou-NP showed some remaining fluorescence for up to 24 h, and Cou-NP-gel showed a clear fluorescence up to 48 h, indicating that the fluorescence was maintained with slow loss. The fluorescence intensity was quantified and is shown graphically in . The overall fluorescence intensity tended to decrease over time in all the formulations. After 6 h, the fluorescence intensity of Cou, Cou-NP, and Cou-NP-gel was 909.4 ± 63.2, 1553.8 ± 225.4, and 2590.8 ± 596.9, respectively. The fluorescence intensity after 48 h was measured at 580.9 ± 26.6, 919.1 ± 101.4, and 1613.4 ± 687.8, respectively, for the three formulations. After 6 h, the fluorescence intensity of Cou was approximately three times lower than that of Cou-NP-gel. These results suggest that most of the Cou solution was lost to the Eustachian tube after IT administration (Salt & Plontke, Citation2018). At all time points, the Cou-NP-gel formulation showed the highest fluorescence intensity and was statistically significant compared to Cou (p<.05). At 48 h, Cou-NP-gel remained at 60% of the fluorescence intensity measured at 6 h and was approximately three times higher than that of Cou. It was confirmed that the Cou-NP-gel remained longer than the other formulations when administered in the middle ear cavity. Thus, the formulations containing PLGA NPs in thermosensitive gels could remain in the middle ear for an extended period of time, increasing the contact time with the RWM. In other studies, PLGA NPs or other NPs have been demonstrated to be distributed in various sites of the cochlea, passing through the RWM after IT administration (Tamura et al., Citation2005; Zhang et al., Citation2011). Therefore, a biodistribution study was needed to confirm whether the DEX-NP-gel formulation could reach the inner ear through the RWM.
Figure 5. In vivo study. (A) Representative fluorescence imaging and (b) fluorescence intensity of Cou, Cou-NP, and Cou-NP-gel. Cou; coumarin-6. Data are expressed as the mean ± S.D. (n = 3). Cou-NP-gel was statistically significant compared to Cou and Cou-NP by a one-way ANOVA and Tukey’s post hoc test (*p<.05, **p<.01).

3.10. Biodistribution study
The amount of DEX remaining in the cochlea is shown in . In the group administered DEX solution, the amount of DEX was detected as 1.83 ± 0.14 ng in the cochleae. In the DEX-NP and DEX-NP-gel group, 2.71 ± 0.53 ng, and 7.20 ± 1.76 ng of DEX was detected in the cochleae, respectively. These results suggest that the PLGA NP formulation in thermosensitive gel via IT administration delivered DEX to the inner ear. In addition, the long residence time of the formulation in the middle ear increased DEX absorption. Although only one time point was studied, it was suggested that the DEX-NP-gel formulation efficiently delivered DEX. Previous studies have confirmed the therapeutic effect of DEX by loading DEX into PLGA NPs to restore hair cells in damaged organs of Corti (Sun et al., Citation2016). Confocal images of the cochlear sections were taken to confirm that the particles were found 4 h after PLGA NPs were administered to the spiral ganglion neurons and stria vascularis at 24 h (Cai et al., Citation2017). These results suggest that DEX-NP-gel formulations may be distributed in various sites in the cochlea within 24 h after IT administration. In addition, more than half of the initial dose remained in the middle ear cavity for up to 48 h. Therefore, it is expected that a single dose of DEX-NP-gel may have a long therapeutic effect.
4. Conclusions
In this study, we evaluated the PLGA NPs of DEX dispersed in poloxamer gels, a thermosensitive gel, for inner ear drug delivery. DEX-NPs were prepared for inner ear drug delivery with a particle size of 150.0 ± 3.2 nm, zeta potential of −18.7 ± 0.6 mV, and an EE of 64.4 ± 2.0%. The NPs were dispersed in a poloxamer mixture that was converted into a gel at body temperature to form the final formulation (DEX-NP-gels). With this formulation, we attempted an in vitro release study using a transdermal diffusion cell system, cell viability, histological evaluation, and in vivo fluorescence imaging. As a result, DEX-NP-gels showed a similar release pattern to DEX-NPs and toxicity at 1 mg/mL in the cell viability study, but no cytotoxicity was observed even at a concentration of 4 mg/mL of DEX-NP-gels. In vivo fluorescence imaging studies also confirmed that DEX-NP-gels remained sufficient for delayed release in the middle ear after IT administration. In the biodistribution study, the longest-staying DEX-NP-gels in the middle ear were well absorbed into the inner ear. Therefore, our prepared DEX-NP-gels are a valuable potential formulation for inner ear drug delivery.
Supplemental Material
Download ()Disclosure statement
No potential conflict of interest was reported by the author(s).
Additional information
Funding
References
- Altındal DÇ, Gümüşderelioğlu M. (2016). Melatonin releasing PLGA micro/nanoparticles and their effect on osteosarcoma cells. J Microencapsul 33:53–63.
- Ay Şenyiğit Z, Karavana SY, Ilem Ozdemir D, et al. (2015). Design and evaluation of an intravesical delivery system for superficial bladder cancer: preparation of gemcitabine HCl-loaded chitosan-thioglycolic acid nanoparticles and comparison of chitosan/poloxamer gels as carriers. Int J Nanomedicine 10:6493–507.
- Ban E, Park M, Jeong S, et al. (2017). Poloxamer-based thermoreversible gel for topical delivery of emodin: influence of P407 and P188 on solubility of emodin and its application in cellular activity screening. Molecules 22:246.
- Ahn JB, Kim D-H, Lee S-E, et al. (2019). Improvement of the dissolution rate and bioavailability of fenofibrate by the supercritical anti-solvent process. Int J Pharm 564:263–72.
- Blasi P. (2019). Poly(lactic acid)/poly(lactic-co-glycolic acid)-based microparticles: an overview. J Pharm Investig 49:337–46.
- Buwalda SJ, Boere KWM, Dijkstra PJ, et al. (2014). Hydrogels in a historical perspective: from simple networks to smart materials. J Control Release 190:254–73.
- Byeon JC, Lee S-E, Kim T-H, et al. (2019). Design of novel proliposome formulation for antioxidant peptide, glutathione with enhanced oral bioavailability and stability. Drug Deliv 26:216–25.
- Cai H, Liang Z, Huang W, et al. (2017). Engineering PLGA nano-based systems through understanding the influence of nanoparticle properties and cell-penetrating peptides for cochlear drug delivery. Int J Pharm 532:55–65.
- Cai H, Wen X, Wen L, et al. (2014). Enhanced local bioavailability of single or compound drugs delivery to the inner ear through application of PLGA nanoparticles via round window administration. Int J Nanomedicine 9:5591–601.
- Chiang Z-C, Yu S-H, Chao A-C, Dong G-C. (2012). Preparation and characterization of dexamethasone-immobilized chitosan scaffold. J Biosci Bioeng 113:654–60.
- Chibas LC, Cintra PP, Moreira MR, et al. (2019). Polyalthic acid in polymeric nanoparticles causes selective growth inhibition and genotoxicity in MCF-7 cells. Nat Prod Commun 14:1934578X1984270.
- Choi J-S, Cao J, Naeem M, et al. (2014). Size-controlled biodegradable nanoparticles: preparation and size-dependent cellular uptake and tumor cell growth inhibition. Colloids Surf B Biointerfaces 122:545–51.
- Choi SG, Baek EJ, Davaa E, et al. (2013). Topical treatment of the buccal mucosa and wounded skin in rats with a triamcinolone acetonide-loaded hydrogel prepared using an electron beam. Int J Pharm 447:102–8.
- Dai J, Long W, Liang Z, et al. (2018). A novel vehicle for local protein delivery to the inner ear: injectable and biodegradable thermosensitive hydrogel loaded with PLGA nanoparticles. Drug Dev Ind Pharm 44:89–98.
- Elmowafy EM, Tiboni M, Soliman ME. (2019). Biocompatibility, biodegradation and biomedical applications of poly(lactic acid)/poly(lactic-co-glycolic acid) micro and nanoparticles. J Pharm Investig 49:347–80.
- Engleder E, Honeder C, Klobasa J, et al. (2014). Preclinical evaluation of thermoreversible triamcinolone acetonide hydrogels for drug delivery to the inner ear. Int J Pharm 471:297–302.
- Ersner MS, Spiegel EA, Alexander MH. (1951). Transtympanic injection of anesthetics for the treatment of Méniére's syndrome. AMA Arch Otolaryngol 54:43–52.
- Freitas LM, Antunes FTT, Obach ES, et al. (2021). Anti-inflammatory effects of a topical emulsion containing Helianthus annuus oil, glycerin, and vitamin B3 in mice. J Pharm Investig 51:223–32.
- Ge X, Jackson RL, Liu J, et al. (2007). Distribution of PLGA nanoparticles in chinchilla cochleae. Otolaryngol Head Neck Surg 137:619–23.
- Ghorbani F, Nojehdehian H, Zamanian A. (2016). Physicochemical and mechanical properties of freeze cast hydroxyapatite-gelatin scaffolds with dexamethasone loaded PLGA microspheres for hard tissue engineering applications. Mater Sci Eng C Mater Biol Appl 69:208–20.
- Govender T, Stolnik S, Garnett MC, et al. (1999). PLGA nanoparticles prepared by nanoprecipitation: drug loading and release studies of a water soluble drug. J Control Release 57:171–85.
- Gu X, Zheng Y. (2015). Preparation, characterization, and in vivo study of rhein-loaded poly(lactic-co-glycolic acid) nanoparticles for oral delivery. Drug Des Devel Ther 9:2301–2309.
- Han FY, Thurecht KJ, Whittaker AK, Smith MT. (2016). Bioerodable PLGA-based microparticles for producing sustained-release drug formulations and strategies for improving drug loading. Front Pharmacol 7:185.
- Hargunani CA, Kempton JB, DeGagne JM, Trune DR. (2006). Intratympanic injection of dexamethasone: time course of inner ear distribution and conversion to its active form. Otol Neurotol 27:564–9.
- Hickey T, Kreutzer D, Burgess DJ, Moussy F. (2002). Dexamethasone/PLGA microspheres for continuous delivery of an anti-inflammatory drug for implantable medical devices. Biomaterials 23:1649–56.
- Juhn SK, Rybak LP, Fowlks WL. (1982). Transport characteristics of the blood–perilymph barrier. Am J Otolaryngol 3:392–6.
- Kang B-S, Choi J-S, Lee S-E, et al. (2017). Enhancing the in vitro anticancer activity of albendazole incorporated into chitosan-coated PLGA nanoparticles. Carbohydr Polym 159:39–47.
- Karim MR, Islam MS. (2011). Thermal behavior with mechanical property of fluorinated silane functionalized superhydrophobic pullulan/poly(vinyl alcohol) blends by electrospinning method. J Nanomater 2011:979458.
- Keiler S, Richter C-P. (2001). Cochlear dimensions obtained in hemicochleae of four different strains of mice: CBA/CaJ, 129/CD1, 129/SvEv and C57BL/6J. Hear Res 162:91–104.
- Khalil KA, Fouad H, Elsarnagawy T, Almajhdi FN. (2013). Preparation and characterization of electrospun PLGA/silver composite nanofibers for biomedical applications. Int J Electrochem Sci 8:3483–93.
- Kim D-H, Martin DC. (2006). Sustained release of dexamethasone from hydrophilic matrices using PLGA nanoparticles for neural drug delivery. Biomaterials 27:3031–7.
- Kim D-K. (2017). Nanomedicine for inner ear diseases: a review of recent in vivo studies. Biomed Res Int 2017:3098230.
- Lajud SA, Han Z, Chi F-L, et al. (2013). A regulated delivery system for inner ear drug application. J Control Release 166:268–76.
- Laurell G, Ekborn A, Viberg A, Canlon B. (2007). Effects of a single high dose of cisplatin on the melanocytes of the stria vascularis in the guinea pig. Audiol Neurootol 12:170–8.
- Lemoine D, Francois C, Kedzierewicz F, et al. (1996). Stability study of nanoparticles of poly(ɛ-caprolactone), poly(d,l-lactide) and poly(d,l-lactide-co-glycolide). Biomaterials 17:2191–7.
- Mäder K, Lehner E, Liebau A, Plontke SK. (2018). Controlled drug release to the inner ear: concepts, materials, mechanisms, and performance. Hear Res 368:49–66.
- Mao Y, Li X, Chen G, Wang S. (2016). Thermosensitive hydrogel system with paclitaxel liposomes used in localized drug delivery system for in situ treatment of tumor: better antitumor efficacy and lower toxicity. J Pharm Sci 105:194–204.
- Milojevic Z, Agbaba D, Eric S, et al. (2002). High-performance liquid chromatographic method for the assay of dexamethasone and xylometazoline in nasal drops containing methyl p-hydroxybenzoate. J Chromatogr A 949:79–82.
- Mülazımoğlu S, Ocak E, Kaygusuz G, Gökcan MK. (2017). Retroauricular approach for targeted cochlear therapy experiments in Wistar albino rats. Balkan Med J 34:200–5.
- Muthu MS, Feng S-S. (2009). Pharmaceutical stability aspects of nanomedicines. Nanomedicine 4:857–60.
- Nedzelski JM, Chiong CM, Fradet G, et al. (1993). Intratympanic gentamicin instillation as treatment of unilateral Menière’s disease: update of an ongoing study. Am J Otol 14:278–82.
- Ono C, Tanaka M. (2003). Binding characteristics of fluoroquinolones to synthetic levodopa melanin. J Pharm Pharmacol 55:1127–33.
- Pool H, Quintanar D, de Dios Figueroa J, et al. (2012). Antioxidant effects of quercetin and catechin encapsulated into PLGA nanoparticles. J Nanomater 86:1–12.
- Prakash K, Sireesha KR, Kumari AS. (2012). Stability indicating HPLC method for simultaneous determination of dexamethasone sodium phosphate and chloramphenicol in bulk and formulations. Int J Pharm Pharm Sci 4:505–10.
- Russo E, Villa C. (2019). Poloxamer hydrogels for biomedical applications. Pharmaceutics 11:671.
- Saegusa Y, Tabata H. (2003). Usefulness of infrared thermometry in determining body temperature in mice. J Vet Med Sci 65:1365–7.
- Salt AN, Hartsock J, Plontke S, et al. (2011). Distribution of dexamethasone and preservation of inner ear function following intratympanic delivery of a gel-based formulation. Audiol Neurootol 16:323–35.
- Salt AN, Plontke SK. (2018). Pharmacokinetic principles in the inner ear: influence of drug properties on intratympanic applications. Hear Res 368:28–40.
- Schmolka IR. (1972). Artificial skin. I. Preparation and properties of Pluronic F-127 gels for treatment of burns. J Biomed Mater Res 6:571–82.
- Schoubben A, Ricci M, Giovagnoli S. (2019). Meeting the unmet: from traditional to cutting-edge techniques for poly lactide and poly lactide-co-glycolide microparticle manufacturing. J Pharm Investig 49:381–404.
- Schuknecht HF. (1956). Ablation therapy for the relief of Meniere’s disease. Laryngoscope 66:859–70.
- Shkodra-Pula B, Grune C, Traeger A, et al. (2019). Effect of surfactant on the size and stability of PLGA nanoparticles encapsulating a protein kinase C inhibitor. Int J Pharm 566:756–64.
- Soken H, Robinson BK, Goodman SS, et al. (2013). Mouse cochleostomy: a minimally invasive dorsal approach for modeling cochlear implantation. Laryngoscope 123:E109–E15.
- Sun C, Wang X, Chen D, et al. (2016). Dexamethasone loaded nanoparticles exert protective effects against cisplatin-induced hearing loss by systemic administration. Neurosci Lett 619:142–8.
- Swider E, Maharjan S, Houkes K, et al. (2019). Förster resonance energy transfer-based stability assessment of PLGA nanoparticles in vitro and in vivo. ACS Appl Bio Mater 2:1131–40.
- Tamura T, Kita T, Nakagawa T, et al. (2005). Drug delivery to the cochlea using PLGA nanoparticles. Laryngoscope 115:2000–5.
- Toth AA, Parnes LS. (1995). Intratympanic gentamicin therapy for Menière’s disease: preliminary comparison of two regimens. J Otolaryngol 24:340–4.
- Van De Water TR, Abi Hachem RN, Dinh CT, et al. (2010). Conservation of hearing and protection of auditory hair cells against trauma-induced losses by local dexamethasone therapy: molecular and genetic mechanisms. Cochlear Implants Int 11:42–55.
- Wang X, Dellamary L, Fernandez R, et al. (2009). Dose-dependent sustained release of dexamethasone in inner ear cochlear fluids using a novel local delivery approach. Audiol Neurootol 14:393–401.
- Wrześniok D, Surazyński A, Karna E, et al. (2005). Melanin counter act puromycin-induced inhibition of collagen and DNA biosynthesis in human skin fibroblasts. Life Sci 77:528–38.
- Yoon JJ, Kim JH, Park TG. (2003). Dexamethasone-releasing biodegradable polymer scaffolds fabricated by a gas-foaming/salt-leaching method. Biomaterials 24:2323–9.
- Zhang X, Chen G, Wen L, et al. (2013). Novel multiple agents loaded PLGA nanoparticles for brain delivery via inner ear administration: in vitro and in vivo evaluation. Eur J Pharm Sci 48:595–603.
- Zhang Y, Zhang W, Löbler M, et al. (2011). Inner ear biocompatibility of lipid nanocapsules after round window membrane application. Int J Pharm 404:211–9.