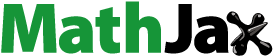
ABSTRACT
In the current study, taro starch was extracted and used for the encapsulation of probiotics to prolong their viability under stressed conditions. Taro starch and sodium alginate were used as wall materials for the encapsulation of Lactobacillus rhamnosus. Probiotic bacteria were encapsulated by the extrusion method, and obtained microbeads were subjected to various morphological, molecular, and structural characterization using Fourier transform infrared spectroscopy ;(FTIR), Scanning electron microscopy (SEM), and X-ray diffraction (XRD) technique. Furthermore, the viability of free and encapsulated probiotics was also accessed under simulated gastrointestinal conditions and in the food model (cheddar cheese). Average size microcapsules ranged from 6.16 ± 0.05 mm to 5.28 ± 0.03 mm. The encapsulation efficiency for taro and sodium alginate was recorded as 86.27% log CFU/g and 81.78% log CFU/g respectively. SEM micrographs exhibited entrapment of probiotics in wall materials. The surface of capsules was-irregular spherical structure FTIR spectra revealed broad characteristic peaks at 815 cm1, 1320 cm1, 1130 cm1, and ~ 1610 cm1. XRD images showed loss of crystalline structure following encapsulation. Higher probiotic viability was recorded under simulated gastrointestinal conditions for encapsulated probiotics compared to free probiotics. Likewise, a high probiotic count was observed in cheese fortified with encapsulated probiotics. Conclusively, taro starch wall material showed overall best results regarding the viability of probiotics under stressed conditions.
Introduction
The use of probiotic microbes has expanded due to growing knowledge about their links to several health advantages, particularly for the digestive system, when given in the right amounts. According to studies, probiotic bacteria must be consumed at a concentration of 106 to 107 CFU/g or CFU/mL.[Citation1] Probiotics maintain a healthy gut microbiota, limit pathogenic bacteria, relieve constipation, stimulate the immune system, synthesize vitamins and antibacterial compounds, and promote calcium absorption.[Citation2] Contrarily, live probiotics must survive an adverse gastric environment and make it to the large intestine in sufficient numbers before they may benefit the host. The significant barriers to the bacteria’s survival in gastric transit are the duodenum’s bile salts and the stomach’s acidic environment.[Citation3] Moreover, when supplemented into food systems, the strains of probiotics must endure the various unit processes in food processing. With the passage of time, microencapsulation technique has been introduced that is used to protect probiotic bacteria from the extreme environmental conditions.[Citation4]
Several studies have reported the protective role of microencapsulation is a promising method for bacterial cell protection.[Citation5] Several encapsulating technologies have been reported, including spray drying, extrusion coacervation, and emulsion.[Citation6] Diverse wall materials, particularly macromolecules including alginates, starch, proteins, pectin, glucan, chitosan, carrageenan, and celluloses, have been used to encapsulate various active substances.[Citation7] For the food industry, developing functional microcapsules containing core ingredients is crucial. Starch is a natural biodegradable polymer that encapsulates probiotics and bioactive compounds. Due to their increased coating properties and inter- and intra-granule voids, they make suitable encapsulating materials. Researchers have encapsulated probiotics in native starch molecules.[Citation8]
Therefore, examining the effectiveness of starch-based microparticles used in probiotic encapsulation is necessary. In the present study, a unique source of starch was used from taro (Colocasia esculenta), which is widely cultivated in humid, warm subtropical climates such as Nigeria, Africa, and Burkina Faso, as well as in India (according to the nutritional capacity of the soil in these lands). Taro (Colocasia esculenta) contains 73–80% starch,[Citation9] .8% fiber, 1.2% ash content, 1.5% protein content, and 0.2% fat.[Citation10] Many studies have reported Taro starch as an effective encapsulating material for probiotics.[Citation11] However, despite demonstrating results for the delivery of bioactive substances, there is still uncertainty whether the micro materials are appropriate for encapsulating live probiotic cells.[Citation12] This present study was designed to encapsulate probiotics using taro starch and to evaluate the stability of Lactobacillus rhamnosus. Moreover, the beads were characterized morphologically and structurally using various techniques and under simulated study.
Materials and methods
The freeze-dried culture of Lactobacillus rhamnosus was obtained from the culture bank of the National Institute of Food Science and Technology (NIFSAT), University of Agriculture Faisalabad. Taro corm were purchased from Jhang Bazar, Faisalabad (Pakistan). Encapsulating materials, chemicals and glassware were purchased from Sigma- Aldrich (St. USA). The study was conducted at the Food Safety and Biotechnology laboratory and Food Analysis laboratory of the Department of Food Science, Government College University Faisalabad, Pakistan.
Extraction of taro starch
The extraction of taro starch was carried out according to the method described by Anwar et al..[Citation13] Taro corms were washed to remove soil and other contaminants and peeled to remove defects. Taro was diced and placed in a 500 ml beaker with 3 quarts of water, then blended at low speed for 40 seconds to get a smooth slurry that was sieved to remove fiber. After two days, the starch mixture was rinsed numerous times until a clear liquid emerged on the surface and the starchy material dropped to the bottom. The starch slurry was homogenized (FSH-2A, China) and centrifuged (Heraeus megafuge, 8 R, Thermo-scientific, Germany). The drying oven (202- 0A, Golden) at 70°C removed water from starch cakes. The ground dry starch was stored at room temperature in food-grade containers. (25°C).
Bacterial culture activation
The freeze-dried probiotic culture was activated using the technique described by Afzaal et al..[Citation14] For bacterial activation, the cells of L. rhamnosus were grown in Man Rogosa and Sharpe (MRS) broth for approximately 24 hours in an incubator (memmert, Germany) at 37°C. For multiplication, L. rhamnosus cells were inoculated in 100 mL of Man Rogosa and Sharpe (MRS) broth. For 24 hours, the bacterial culture was incubated at 37°C. Following the collection of the cells, the medium was decanted, and the cells were centrifuged (Heraeus megafuge, 8 R centrifuge, Thermo-scientific, Germany). The cells were then suspended again in freshly made MRS medium, incubated for an additional 20 hours at 37°C, and decanted the medium. Data was logged, cells were collected, and they were weighed. The cell concentration was adjusted to 109 cfu/g.
Preparation of wall material and encapsulation process
Encapsulated taro and alginate cells were prepared by following the method of Tan et al..[Citation15] 10% of taro starch was dispersed using distilled water and heated using a hot plate for 30 minutes at 500 rpm. 2.0% (w/v) sodium alginate was used and dispersed in distilled water for sodium alginate solution. The mixtures were cooled down to room temperature the probiotics were immediately added to the solution in the 109 CFU/mL range. The extrusion method was used to prepare capsules, and the capsules were hardened by adding CaCl2 (0.05 M, 50 mL) dropwise in the solution. The microbeads obtained were cleaned with distilled water and kept for later analysis.
Encapsulation efficiency
The entrapment efficiency for probiotic cells was noted by following the method of Ghumman et al..[Citation16] The microbeads of taro starch and Sodium Alginate were taken randomly, and beads dissolution was performed using a stomacher bag with the pour plate method; afterward released cell number was calculated. The findings were expressed as cfu/bead. Using the following formulas, encapsulation yield was evaluated:
Encapsulation Efficiency = EE =
where N is number of live entrapped cells released, No is number of free cells added before encapsulation.
Particle size
The size of encapsulated probiotic microbeads was examined immediately following microencapsulation by using static light scattering instrument (Mastersizer S, Malvern Instruments, Worcestershire, UK), the method prescribed by Azizi et al..[Citation17] To size and structure of the beads were ensured. The particle sizes are reported as the surface-weighted mean diameter.
Zeta potential
Zeta potential was determined by the following method, as described by Fontes et al..[Citation18] Briefly, the samples were analyzed using a zeta potential analyzer or zeta-meter. In the aqueous suspension of taro and alginate, the zeta potential measures the strength of electrostatic attraction or repulsion between particles.
Scanning electron microscopy
The ocular and stage micrometers, together with a light microscope, were used to measure the beads’ sizes. The surface and interior appearance of the sodium alginate and taro starch beads were also analyzed by scanning electron microscopy utilizing the freeze-fracture/cold block method, as described by Cui et al..[Citation19] This technique involved placing unfixed samples on the block, freezing them in liquid nitrogen, and then examining them using a Philips electron microscope (model 505) at an accelerating voltage of 15.0 kV.
FTIR (Fourier transform infrared) spectroscopy
Taro starch and sodium alginate microencapsulated probiotic molecules. Infrared spectra were measured using an FTIR spectrophotometer using the method of Fareed et al..[Citation20] The sample was pressed to transform into pellets after mixing with potassium bromide. The prepared pellets were scanned at the 4000 to 600 cm − 1 range, and the spectra of samples were analyzed by system software.
X-ray diffraction (XRD) technique
The probiotic-encapsulated taro starch and sodium alginate microencapsulated particles were examined using the X-ray diffraction technique, as performed previously by Tian et al..[Citation21] An AXS Analytical X-Ray Systems Siemens D 5005 (Germany) diffractometer present at Central Hi-tech Lab, Government College University Faisalabad, was used to detect amorphousness and crystallinity of internal structure with Cu K alpha radiation (λ = 1.54056A°), voltage 40 kV and 40 mA current. The scanning angle ranged from 3 to 90° of 2θ, 2°/min, and 0.033°/s.
Simulated gastrointestinal analysis
Simulated gastric juice was prepared to determine the viability of free and encapsulated cells according to the protocol followed by Singh et al..[Citation22] For this purpose, pepsin (Sigma-Aldrich P7000) was dissolved in an aseptic sodium chloride solution (0.5%,w/v) at 2.5 g/L concentration, and the pH was adjusted to 2.0 by using HCl. The solution was poured into three test tubes, and the cells were added to an artificially created gastric solution (9.8 log CFU/mL). Afterward, the tubes containing the solution were incubated for 30, 60, 90, and 120 minutes at 37°C, and the viability was determined by incubating the cells in MRS agar aerobically.
According to the method followed by Nasiri et al.,[Citation23] the viability of encapsulated and free probiotics under artificial intestinal fluid was determined. The solution was prepared by mixing pancreatin (Sigma-Aldrich P-1500) in sodium chloride solution (0.5%w/v) 1 g/L and 4.25% bile salts while the pH was adjusted to 8.0 using 0.1 M NaOH. The solution was poured into three test tubes, and the cells were added to an artificially created intestinal solution (9.8 log CFU/mL). The tubes containing solution were incubated for 30, 60, 90, and 120 minutes at 37°C, and the viability was determined by incubating the cells in MRS agar aerobically.
Thermal stability of microcapsules
To investigate the microcapsules’ thermal stability, 10 mL of distilled water was introduced to one gram of the manufactured microcapsules in sterile test tubes. The test tubes were cooled using chilled water after exposure to 40°, 50°, and 75°C for 5 min. The bacteria were counted using the after-mentioned technique for three duplicates by the method described by Ma et al..[Citation24]
Viability of taro starch and sodium alginate encapsulated probiotics during refrigeration storage
The process of Brinques and Ayub[Citation25] is followed with minor modifications to examine probiotic resistance at different storage temperatures. The viability of the probiotic was tested by incubating 0.4 ml (about 9.5 log cfu g-1) of free cell, taro starch, and sodium alginate IN 1.8 ml of sterile sodium chloride solution (0.5% w/v) at 4°C. Every week, 1 ml aliquots were obtained to determine the total number of live cells. The SLMs were immobilized and dissolved in the appropriate buffer solution to determine the total number of viable cells.
Results and discussion
Encapsulation yield
The encapsulation efficiency has a direct relationship with the components of the wall media. The survivability of encapsulated material also depends on the efficient intestinal conditions.[Citation26] The viability and cell death of L. rhamnosus after encapsulation in alginate and taro starch are shown in . The initial count of cells before encapsulation was in the range of 9.99 log CFU/g, respectively. After encapsulation, the cell count was lowered to 8.73 ± 0.03 log CFU/g and 8.17 ± 0.02 log CFU/g which corresponds to cell death. Moreover, the highest yield for taro starch encapsulated beads was recorded as 87.38 (2.12%), whereas, for alginate beads the final yield obtained was 81.78 (1.53%). However, the results of the present study suggest starch microcapsules were to be highly efficient. Zanjani et al.[Citation27] found chitosan-starch and alginate-starch blends efficiency reached over 97% and increased the viability and storage condition.
Table 1. Mean values for Encapsulation efficiency, Size and Zeta-Potential of taro starch and sodium-alginate microbeads.
The values are represented as Mean± STD, superscripts are used to represent statistically significant differences (p < .05).
Size of micro-beads
Lactobacillus rhamnosus was encapsulated in taro starch and alginate wall material, and the prepared beads were examined for their size and morphology under a light microscope (Intech 101-A, China). The size of the capsules coated with taro starch was found to be 5.28 ± 0.05 mm, however, capsules having an alginate wall or coating material had a capsule size of 6.16 ± 0.03 mm. shows the mean values recorded for the size of the beads. Afzaal et al.[Citation28] studied the encapsulation of different wall materials and concluded that the form of encapsulating matrices and method directly affects the size of microbeads. Under the light microscope, the shape of the beads appeared to be spherical, and the probiotics seemed to be entrapped in the wall material when examined under the scanning electron microscope. The shape and size of the capsules are important as they impact the product’s overall organoleptic properties. The smaller the size of the capsule, the higher the chances of the product getting accepted. However, small capsule size has a disadvantage; as the walls of capsules become weaker, the probiotic inside has more chances to be released into the environment upon fracture due to the fragile and brittle surroundings of the walls.[Citation29]
Zeta potential (ζ-potential)
Zeta potential is another critical parameter for determining ionic strength and surface electrical attributes of encapsulated bacterial cells and defines its electrochemical properties. shows the mean values obtained for the zeta potential of prepared beads. Results showed that the wall material greatly impacted the zeta potential. The obtained results showed negative values that showed excellent ionic strength among wall materials. The maximum reading was obtained for beads that were encapsulated with taro starch. However, the capsules with alginate coating showed a lesser value due to the amount of different encapsulating materials, ionic strength, and pH value of the solution.[Citation30]
Morphological characterization of microencapsulated beads by scanning electron microscopy (SEM)
The detailed structure of morphology of microencapsulated L. rhamnosus encapsulated in taro starch, and alginate coatings was examined through SEM, and the images are shown in (). The fine micro-sized taro starch particles were used for the encapsulation of probiotic bacteria, while the other material used was sodium alginate having fine particles. It can be observed from that probiotics having taro starch encapsulation have covered all the pores of the wall material due to fine micro-starch particles and showed some cubic and tubular type appearance. However, the image shows the visual presentation of probiotics encapsulated with sodium alginate, clear large-sized pores can be observed in the structure of the wall coating. It indicates that the structure on addition in the simulated gastrointestinal solutions or thermal treatment wreak the probiotics easily dispersed in the surrounding environment. Thus, the wall material made of taro starch provides more protection to the probiotics in artificial gastrointestinal solutions. Hence, it increased the viability of the encapsulated probiotics and resulted in the enhanced survival rate of probiotic bacteria.
It has been reported that the decreased diameter of microcapsules can reduce the protective effect of microencapsulation while increasing the microcapsule diameter reduces the death of probiotic cells.[Citation31] A similar research was also conducted by Talebian et al.[Citation32] suggested that the use of biopolymer as a wall material for the encapsulation of probiotics. The result of the study suggests that the multilayer encapsulation of biopolymers provide better protection to the probiotic cells under extreme simulated conditions.
X-ray diffraction (XRD)
shows the X-ray diffraction (XRD) analysis of the taro and alginate encapsulated beads. The taro starch beads showed distinctive peaks that disclose the amorphous structure of taro starch; however, the intensity of the peaks showed a declining trend afterward. It was clear from the XRD analysis that alginate showed a lower intensity peak as compared to taro starch treatment peaks. A similar study was performed.[Citation33] The difference in peak intensity may ascend from the interaction between different groups of polysaccharides through OH-bonding. Similarly, Das and Sit developed taro starch nanoparticles which also showed crystalline nature.[Citation34]
FTIR analysis
FTIR analysis for taro starch encapsulated beads is shown in . It is possible to identify at 3800 cm1 COO group stretching vibrations that is the distinguishing property of taro starch structure. The alginate M/G ratio alterations have been documented in FTIR analysis, where mannuronic acid units usually have a band at about 825 cm1.. On the other hand, it is identified that no peak at 825 cm1 was recorded; however, there it is also noted that another peak at 809 cm1 has appeared. These circumstances are associated with the lower amounts of residues of M in LFR5/60 alginate because it is the one with the maximum content of G. however, other peaks are also present that suggest the presence of G residues in high amounts, i.e., the peaks at 1325 cm1 and 1135 cm1. Alginate beads samples have comparable results compared with the results of powder, however, some differences have been identified between both samples. The peak representing O-H stretching at ~ 3340 cm1 has been noted to amplify its intensity in all beads samples compared with powder samples; this indicates the presence of water. While the peak at 1610 cm1, which also exhibits a higher intensity in beads samples due to the replacement of sodium ions for calcium ions, which changes the charge density of carboxyl groups, is another significant difference between beads and powder samples. When comparing the bead spectra, it was indicated that the alginate LFR5/60 had a larger rise, which is another intriguing piece of information concerning this intensity increase in the 1610 cm1 peak. These results can be explained by the fact that this sample’s highest concentration of G residues produced samples with a higher calcium content, which significantly influenced these peak values.
Simulated gastrointestinal analysis
The mean results of the viability of free and encapsulated L. rhamnosus exposed to SIC (simulated intestinal conditions) are shown in . From the results, it can be noted that the viability of both microencapsulated and free probiotics reduces significantly (p < .05). Moreover, regarding the survivability of microcapsules, a significant decrease has been observed in all treatments as compared to the initial count (p < .05). A log reduction of 7.84 cfu/mL has been observed in free probiotic treatment, whereas, 1.78 cfu/mL and 2.81 cfu/mL was observed in Taro treatment and alginate treatment, respectively. The survival rate was higher at 0 min (9.98) and lowest at 120 min (5.84). The minimum value for SGJ was detected as 5.78 in FP (free probiotics). The probiotic population was greatest in TSE (8.21 ± 0.02), This higher probiotic yield might be due to better capsule formation, lower capsule size, and less porous structure than sodium alginate beads. Greater pores allow more probiotic dissolution in the simulated fluid and hence cannot maintain better probiotic viability, as in sodium alginate (7.17 ± 0.02) after 120 minutes. The study findings are in accordance with Guo et al..[Citation35] These results also align with the findings of Pradeep & Charalampopoulos.[Citation36]
Table 2. Mean significant values for simulated gastric fluid (SGF) and simulated intestinal fluid (SIF).
The mean results for microbeads compared to free probiotics under simulated intestinal fluid (SIF) are shown in , illustrating that the maximum reading for SIF was detected in treatment TSE (7.61 ± 0.01). In contrast, the minimum value for SIF (1.12 ± 0.01) was detected in FP (Free probiotics). A log reduction of 8.86 cfu/mL has been observed in the FP treatment, whereas 2.37 cfu/mL and 2.76 cfu/mL were observed in the TSE treatment and SAE, respectively.
Table 3. Mean significant values of probiotic viability during elevated temperatures (40°C, 60°C and 75°C).
The results showed that taro starch is a better wall material for the encapsulation of probiotics due to its lower capsule size and porous structure. When inserted in the bile salts and enzymes, the solution may enter the capsules’ pores, dissolving the capsules and exposing the probiotics in an extreme environment that results in the death of probiotics. The findings of the study are in accordance with Pourjavid et al..[Citation37] The results also align with Silva et al.[Citation38] who found that free probiotics did not survive well under artificial intestinal conditions.
Thermal resistance of free and encapsulated probiotics
The mean results for the effect of heat tolerance on free and encapsulated treatments is shown in . The results depicted a reducing trend in probiotic viability in all treatments with an increase in temperature. The initial probiotic levels were in the range of 9.9–10 log cfu, which decreases as the temperature reaches 60°C; however, a rapid fall was observed at 75°C. The mean results from all the treatments of probiotics (free and encapsulated) illustrated that the lowest reading for heat tolerance (3.25 ± 0.01) was detected in treatment FP (Free probiotics), while the maximum value for heat tolerance (9.67 ± 0.01) was detected in TSE (taro encapsulated treatment) and however, SAE showed lower vitality than TSE (8.72 ± 0.01). These results discussed above showed that the microencapsulation of probiotics provides a better barrier even at elevated temperatures. The findings of this research are in line with the findings of de Araújo et al..[Citation39]
Refrigeration storage analysis
The mean results for the effect of refrigeration storage on the replicates of treatments are shown in . The mean square results from all the treatments illustrated that the maximum reading for refrigeration storage (8.54 ± 0.01) on the 21st day of storage was detected in treatment TSE (encapsulated with taro starch) while the minimum value for refrigeration storage (3.16 ± 0.01) was detected in FP. SAE depicts a lower probiotic result (8.57 ± 0.01) after 21 days of storage study at 4°C. The present study indicates that combining taro starch as an encapsulating material can increase the chances for the probiotic bacteria to survive even at low refrigeration temperatures. The findings of the study are in line with the results of Shivani and Sathiavelu.[Citation40]
Table 4. Mean values for probiotic viability during refrigeration storage (4°C) for 21 days.
Conclusion
The current study was conducted to determine the potential of taro starch for encapsulating probiotics to prolong their viability under stressed conditions. Taro starch and sodium alginate were used as wall materials to synthesize microbeads for the encapsulation of probiotic bacteria. The structural and morphological characterization of the beads suggests that taro starch is a better medium to encapsulate the probiotic bacteria with lower bead size and less porous structure than alginate microbeads, resulting in higher probiotic yields. The microbeads of taro starch can resist extreme gastric and intestinal conditions and maintain probiotic viability at elevated temperatures.
Ethical approval
This article does not contain any studies with human participants or animals performed by any of the authors.
Credit authorship contribution statement
Waqas Zubair, Muhammad Afzaal and Farhan Saeed proposed this idea and conducted this research, Huda Ateeq, Noor Akram, Yasir Abbas Shah, Aasma Asghar, Salim Manoharadas, Asad Nawaz, Degnet Teferi Asres prepared the manuscript and also aided in conducting this research and in preparing figures and tables.
Consent to participate
Corresponding and all the coauthors are willing to participate in this manuscript.
Acknowledgments
The authors thank the Research Supporting Project for funding this work through Research Supporting Project number (RSPD2023R708), King Saud University, Riyadh, Saudi Arabia.
Disclosure statement
No potential conflict of interest was reported by the authors.
Data availability statement
Even though adequate data has been given in the form of tables and figures, all authors declare that if more data is required, the data will be provided on a request basis.
Additional information
Funding
References
- DUREC, J.; SMORÁDKOVÁ, K.; TOBOLKOVÁ, B. Evaluation of Lactibacillus Rhamnosus LGG and Bifidobacterium BB-12 Growth in Mixed Plant-Based Beverages in Industrial Pilot Production. J. Food . Nutr. Res. 2023, 62(3), 270–276.
- Iancu, M. A.; Profir, M.; Roşu, O. A.; Ionescu, R. F.; Cretoiu, S. M.; Gaspar, B. S. Revisiting the Intestinal Microbiome and Its Role in Diarrhea and Constipation. Microorganisms. 2023, 11(9), 2177. DOI: 10.3390/microorganisms11092177.
- Cassani, L.; Gomez-Zavaglia, A.; Simal-Gandara, J. Technological Strategies Ensuring the Safe Arrival of Beneficial Microorganisms to the Gut: From Food Processing and Storage to Their Passage Through the Gastrointestinal Tract. Food Res. Int. 2020, 129, 108852. DOI: 10.1016/j.foodres.2019.108852.
- Xu, Y.; Dong, M.; Xiao, H.; Young, Q. S.; Ogawa, Y.; Ma, G.; Zhang, C.; Advances in Spray-Dried Probiotic Microcapsules for Targeted Delivery: A Review. Crit. Rev. Food Sci. Nutr. 2023, 1–17. doi: 10.1080/10408398.2023.2235424.
- Călinoiu, L. F.; Ştefănescu, B. E.; Pop, I. D.; Muntean, L.; Vodnar, D. C. Chitosan coating applications in probiotic microencapsulation. Coatings. 2019, 9(3), 194. DOI: 10.3390/coatings9030194.
- Alemzadeh, I.; Hajiabbas, M.; Pakzad, H.; Sajadi, D. S.; Vossoughi, A. Encapsulation of Food Components and Bioactive Ingredients and Targeted Release. Int. J. Eng. 2020, 33(1), 1–11.
- Utama, G. L.; Oktaviani, L.; Balia, R. L.; Rialita, T. Potential Application of Yeast Cell Wall Biopolymers as Probiotic Encapsulants. Polymers. 2023, 15(16), 3481. DOI: 10.3390/polym15163481.
- Ashwar, B. A.; Gani, A.; Gani, A.; Shah, A.; Masoodi, F. A. Production of RS4 from Rice Starch and Its Utilization as an Encapsulating Agent for Targeted Delivery of Probiotics. Food. Chem. 2018, 239, 287–294. DOI: 10.1016/j.foodchem.2017.06.110.
- Momin, M. C.; Mitra, S.; Jamir, A. R.; Kongbrailatpam, M. Evaluation of Physicochemical Properties in Different Cultivars of Taro [Colocasia Esculenta (L.) Schott]: A Comparative Study. Pharm Innov J. 2021, 40(14), 1–9 .
- Shah, Y. A.; Saeed, F.; Afzaal, M.; Waris, N.; Ahmad, S.; Shoukat, N.; Ateeq, H. Industrial Applications of Taro (Colocasia Esculenta) as a Novel Food Ingredient: A Review. J. Food Process. Preserv. 2022, 46(11), 16951. DOI: 10.1111/jfpp.16951.
- Barroso, L.; Viegas, C.; Vieira, J.; Ferreira-Pêgo, C.; Costa, J.; Fonte, P. Lipid-Based Carriers for Food Ingredients Delivery. J. Food Eng. 2021, 295(1), 110451. DOI: 10.1016/j.jfoodeng.2020.110451.
- Reque, P. M.; Brandelli, A. Encapsulation of Probiotics and Nutraceuticals: Applications in Functional Food Industry. Trends Food Sci. Technol. 2021, 114, 1–10. DOI: 10.1016/j.tifs.2021.05.022.
- Anwar, M.; McConnell, M.; Bekhit, A. E. D. New Freeze-Thaw Method for Improved Extraction of Water-Soluble Non-Starch Polysaccharide from Taro (Colocasia Esculenta): Optimization and Comprehensive Characterization of Physico-Chemical and Structural Properties. Food. Chem. 2021, 349, 129210. DOI: 10.1016/j.foodchem.2021.129210.
- Afzaal, M.; Saeed, F.; Saeed, M.; Ahmed, A.; Ateeq, H.; Nadeem, M. T.; Tufail, T. Survival and Stability of Free and Encapsulated Probiotic Bacteria Under Simulated Gastrointestinal Conditions and in Pasteurized Grape Juice. J. Food Process. Preserv. 2020, 44(3), e14346. DOI: 10.1111/jfpp.14346.
- Tan, H. A.; Dizon, E.; Barraquio, V.; Mercado, S. Efficacy of Alginate-Taro (Colocasia Esculenta L. Schott) Starch for Yoghurt Starter Culture Encapsulation. J. Sci. Engin. Technol. 2016, 4(1), 1–10. DOI: 10.61569/zeywtk92.
- Ghumman, S. A.; Bashir, S.; Noreen, S.; Khan, A. M.; Malik, M. Z. Taro-Corms Mucilage-Alginate Microspheres for the Sustained Release of Pregabalin: In vitro & in vivo Evaluation. Int. J. Biol. Macromol. 2019, 139, 1191–1202. DOI: 10.1016/j.ijbiomac.2019.08.100.
- Azizi, S.; Rezazadeh-Bari, M.; Almasi, H.; Amiri, S. Microencapsulation of Lactobacillus Rhamnosus Using Sesame Protein Isolate: Effect of Encapsulation Method and Transglutaminase. Food. Biosci. 2021, 41, 101012. DOI: 10.1016/j.fbio.2021.101012.
- Fontes, G. C.; Calado, V. M. A.; Rossi, A. M.; Rocha-Leão, M. H. M. D. Characterization of Antibiotic-Loaded Alginate-OSA Starch Microbeads Produced by Ionotropic Pregelation. Biomed Res. Int. 2013, 2013, 1–11. DOI: 10.1155/2013/472626.
- Cui, C.; Jiang, H.; Guan, M.; Ji, N.; Xiong, L.; Sun, Q. Characterization and in vitro Digestibility of Potato Starch Encapsulated in Calcium Alginate Beads. Food. Hydrocoll. 2022, 126, 107458. DOI: 10.1016/j.foodhyd.2021.107458.
- Fareed, F.; Saeed, F.; Afzaal, M.; Imran, A.; Ahmad, A.; Mahmood, K.; Shah, Y. A.; Hussain, M.; Ateeq, H. Fabrication of Electrospun Gum Arabic–Polyvinyl Alcohol Blend Nanofibers for Improved Viability of the Probiotic. J. Food Sci. Technol. 2022, 59(12), 4812–4821. DOI: 10.1007/s13197-022-05567-1.
- Tian, S.; Xue, X. A.; Wang, X.; Chen, Z. Preparation of Starch-Based Functional Food Nano-Microcapsule Delivery System and Its Controlled Release Characteristics. Front. Nutrit. 2022, 9, 982370. DOI: 10.3389/fnut.2022.982370.
- Singh, M.; Sharma, D.; Chauhan, R.; Goel, G. Skimmed Milk-Based Encapsulation for Enhanced Stability and Viability of Lactobacillus Gastricus BTM 7 Under Simulated Gastrointestinal Conditions. Probiotics Antimicrob. Proteins. 2019, 11(3), 850–856. DOI: 10.1007/s12602-018-9472-1.
- Nasiri, H.; Golestan, L.; Shahidi, S. A.; Darjani, P. Encapsulation of Lactobacillus Casei in Sodium Alginate Microcapsules: Improvement of the Bacterial Viability Under Simulated Gastrointestinal Conditions Using Wild Sage Seed Mucilage. J. Food Meas. Charact. 2019, 15(5), 4726–4734. DOI: 10.1007/s11694-021-01022-5.
- Ma, X.; Liu, Y.; Liu, H.; Xu, B.; Zhou, J.; Xiao, F. Synthesis and Characterization of Microencapsulated Paraffin with TiO2 Shell as Thermal Energy Storage Materials. J. Mater. Sci. Mater. Electron. 2018, 29(17), 15241–15248. DOI: 10.1007/s10854-018-9666-z.
- Brinques, G. B.; Ayub, M. A. Z. Effect of Microencapsulation on Survival of Lactobacillus Plantarum in Simulated Gastrointestinal Conditions, Refrigeration, and Yogurt. J. Food Eng. 2011, 103(2), 123–128. DOI: 10.1016/j.jfoodeng.2010.10.006.
- Farahmand, A.; Ghorani, B.; Emadzadeh, B.; Sarabi-Jamab, M.; Emadzadeh, M.; Modiri, A.; Tucker, N. Millifluidic-Assisted Ionic Gelation Technique for Encapsulation of Probiotics in Double-Layered Polysaccharide Structure. Food Res. Int. 2022, 160, 111699. DOI: 10.1016/j.foodres.2022.111699.
- Zanjani, M. A. K.; Ehsani, M. R.; Ghiassi Tarzi, B.; Sharifan, A. Promoting Lactobacillus Casei and Bifidobacterium adolescentis Survival by Microencapsulation with Different Starches and Chitosan and Poly L‐Lysine Coatings in Ice Cream. J. Food Process. Preserv. 2018, 42(1), e13318. DOI: 10.1111/jfpp.13318.
- Afzaal, M.; Saeed, F.; Hussain, M.; Ismail, Z.; Siddeeg, A.; Ammar, A. F.; Aljobair, M. O. Influence of Encapsulation on the Survival of Probiotics in Food Matrix Under Simulated Stress Conditions. Saudi J. Biol. Sci. 2022, 29(9), 103394. DOI: 10.1016/j.sjbs.2022.103394.
- Feng, C.; Zong, X.; Cui, B.; Guo, H.; Zhang, W.; Zhu, J. Application of Carrier Materials in Self-Healing Cement-Based Materials Based on Microbial-Induced Mineralization. Crystals. 2022, 12(6), 797. DOI: 10.3390/cryst12060797.
- A M, H.-A.; Sarabia-Sainz, J. A. I.; González-Rios, H.; Vázquez-Moreno, L.; Montfort, G. R. C. Airbrush Encapsulation of Lactobacillus Rhamnosus GG in Dry Microbeads of Alginate Coated with Regular Buttermilk Proteins. LWT. 2020, 117, 108639. DOI: 10.1016/j.lwt.2019.108639.
- Liao, Y.; Hu, Y.; Fu, N.; Hu, J.; Xiong, H.; Chen, X. D.; Zhao, Q. Maillard Conjugates of Whey Protein Isolate–Xylooligosaccharides for the Microencapsulation of Lactobacillus Rhamnosus: Protective Effects and Stability During Spray Drying, Storage and Gastrointestinal Digestion. Food. Funct. 2021, 12(9), 4034–4045. DOI: 10.1039/D0FO03439H.
- Talebian, S.; Schofield, T.; Valtchev, P.; Schindeler, A.; Kavanagh, J. M.; Adil, Q.; Dehghani, F. Biopolymer‐Based Multilayer Microparticles for Probiotic Delivery to Colon. Adv. Healthcare Mater. 2022, 11(11), 2102487. DOI: 10.1002/adhm.202102487.
- Niranjana, P. T.; Prashantha, K. A Review on Present Status and Future Challenges of Starch Based Polymer Films and Their Composites in Food Packaging Applications. Polym. Compos. 2018, 39(7), 2499–2522. DOI: 10.1002/pc.24236.
- Das, A.; Sit, N. Modification of Taro Starch and Starch Nanoparticles by Various Physical Methods and Their Characterization. Starch‐Stärke. 2021, 73(5–6), 2000227. DOI: 10.1002/star.202000227.
- Guo, Q.; Li, S.; Tang, J.; Chang, S.; Qiang, L.; Du, G.; Yue, T.; Yuan, Y. Microencapsulation of Lactobacillus Plantarum by Spray Drying: Protective Effects During Simulated Food Processing, Gastrointestinal Conditions, and in Kefir. Int. J. Biol. Macromol. 2022, 194, 539–545. DOI: 10.1016/j.ijbiomac.2021.11.096.
- Pradeep, P. H.; Charalampopoulos, D. Encapsulation in an alginate–goats’ milk–inulin matrix improves survival of probiotic Bifidobacterium in simulated gastrointestinal conditions and goats’ milk yoghurt. Int. J. Dairy Technol. 2019, 72(1), 132–141. DOI: 10.1111/1471-0307.12568.
- Pourjavid, H.; Ataei, M.; Pourahmad, R.; Anvar, A. A.; Behmadi, H. Improvement of the Quality Parameters of a Novel Synbiotic Yogurt Sauce Using Microencapsulated Lactobacillus Paracasei and Natural Prebiotics. Food Sci. Technol. 2022, 42, 42. DOI: 10.1590/fst.40322.
- Silva, M. P.; Tulini, F. L.; Matos-Jr, F. E.; Oliveira, M. G.; Thomazini, M.; Fávaro-Trindade, C. S. Application of Spray Chilling and Electrostatic Interaction to Produce Lipid Microparticles Loaded with Probiotics as an Alternative to Improve Resistance Under Stress Conditions. Food. Hydrocoll. 2018, 83, 109–117. DOI: 10.1016/j.foodhyd.2018.05.001.
- de Araújo Etchepare, M.; Nunes, G. L.; Nicoloso, B. R.; Barin, J. S.; Flores, E. M. M.; de Oliveira Mello, R.; de Menezes, C. R. Improvement of the Viability of Encapsulated Probiotics Using Whey Proteins. LWT. 2020, 117, 108601. DOI: 10.1016/j.lwt.2019.108601.
- Shivani, T. M.; Sathiavelu, M. A Comprehensive Review on Functionality of Probiotics in Edible Packaging. Packag. Technol. Sci. 2023, 36(1), 15–30. DOI: 10.1002/pts.2690.