ABSTRACT
Introduction
Spinal cord injury (SCI) affects 25,000–50,000 people around the world each year and there is no cure for SCI patients currently. The primary injury damages spinal cord tissues and secondary injury mechanisms, including ischemia, apoptosis, inflammation, and astrogliosis, further exacerbate the lesions to the spinal cord. Recently, researchers have designed various therapeutic approaches for SCI by targeting its major cellular or molecular pathophysiology.
Areas covered
Some strategies have shown promise in repairing injured spinal cord for functional recoveries, such as administering neuroprotective reagents, targeting specific genes to promote robust axon regeneration of disconnected spinal fiber tracts, targeting epigenetic factors to enhance cell survival and neural repair, and facilitating neuronal relay pathways and neuroplasticity for restoration of function after SCI. This review focuses on the major advances in preclinical molecular therapies for SCI reported in recent years.
Expert opinion
Recent progress in developing novel and effective repairing strategies for SCI is encouraging, but many challenges remain for future design of effective treatments, including developing highly effective neuroprotectants for early interventions, stimulating robust neuronal regeneration with functional synaptic reconnections among disconnected neurons, maximizing the recovery of lost neural functions with combination strategies, and translating the most promising therapies into human use.
1. Introduction
After traumatic spinal cord injury (SCI), the initial injury causes tissue damage to the spinal cord and the lesion volume enlarges with time because of secondary injury mechanisms, including ischemic cell impairment, apoptosis, inflammation, and astrogliosis. The secondary cascades severely increase cell loss and lesion volume of the injured spinal cord. The early responses of the injured spinal cord include gradual hemorrhaging, vasospasm, intravascular thrombosis, free radical formation, lipid peroxidation, excitotoxicity, and cell edema. The subsequent inflammation and immunological responses around the lesion further destroy cells around the lesion. Injury to white matter tracts contributes to axon degeneration, apoptotic loss of myelinating oligodendrocytes along the spinal cord tracts, subsequent demyelination, and axon conduction failure. These injury events also result in cavitation, migration, and proliferation of reactive cells, and consequent astrogliosis. The scar tissue around the lesion prevents the extension of secondary injuries but also creates physical and chemical barriers to the elongation of severed axons. Accordingly, SCI frequently causes serious functional deficits mainly due to anatomical disconnection and conduction failure of axonal tracts between the spinal cord segments rostral and caudal to the lesion.
Researchers have recently designed various therapeutic approaches for SCI by targeting major cellular and molecular pathophysiological changes, including secondary injury mechanisms, disconnections of neuronal circuits of the damaged spinal cord, and deficits of essential functions due to SCI. Various neuroprotective drugs or strategies have been designed to target secondary injury mechanisms, including inflammatory and apoptotic factors. Other studies focused on enhancing axon regeneration of axotomized spinal cord tracts by regulating signaling pathways that control neuronal growth. Several approaches could promote robust regeneration in SCI rodents, including targeting mRNA binding protein Lin28a, the master kinase of LKB1 that phosphorylates AMPK members, and some microRNAs (miRNAs) [Citation1,Citation2]. Many groups focused on the potential of neural stem cells (NSC) for treating SCI. Transplants of NSCs into the injured spinal cord may generate new neurons to relay disconnected spinal neuronal pathways. Some preclinical studies showed dramatic axon regeneration of axotomized host neurons into the NSC grafts, which could extend great numbers of new axons into the caudal spinal cord [Citation3]. Other researchers have induced functional neurogenesis and neuroplasticity in animals with SCI or other CNS lesion models by targeting certain cytokines, transcriptional factors, and neurotrophic factors [Citation4].
Some therapeutic strategies that target cellular and molecular pathophysiology have been moved to clinical trials for SCI patients, such as the use of the neuroprotective Na channel blocker riluzole, the cytokine G-CSF, and systemic hypothermia [Citation5]. Other reagents aimed to promote axon regeneration are also under investigation in clinical trials for SCI, such as an anti-Nogo-A antibody (NG-101), a Nogo receptor antagonist [AXER-204 or NgR(310)ecto-Fc], and Elezanumab, a human anti-RGMa monoclonal antibody [Citation5]. Early systemic treatments with gabapentinoid, an α2δ subunit Ca channel blocker, enhanced motor recovery in SCI patients [Citation6]. Gabapentin is an FDA-approved drug used to treat regional seizures and neuropathic pain. Several clinical trials of SCI that target rewiring of spinal neuronal circuits are also ongoing, including transplants of adipose tissue-derived mesenchymal stem cells (MSCs) and neuro-spinal scaffolds, and implanting brain–spine interfaces. The recent clinical trials with transplanting human NSCs into patients with thoracic and cervical SCIs indicated a trend toward motor benefits [Citation7]. This overall review is aimed to provide insight into preclinical therapeutic targets for SCI reported in recent years.
2. Neuroprotection
Researchers have designed numerous strategies for reducing secondary injuries after SCI by targeting different cellular and molecular mechanisms, including excitotoxicity, oxidative stress, inflammation, cell death, energetic dysfunction, and demyelination (). The compounds and genes listed below regulate cell functions usually through numerous molecular mechanisms.
Figure 1. The major recently reported neuroprotective strategies to target secondary injury mechanisms after spinal cord injury (SCI). Various secondary injury mechanisms contribute to continuous damage to the spinal cord after SCI. Numerous preclinical strategies have recently been reported to protect lesioned tissues and improved functional recovery.
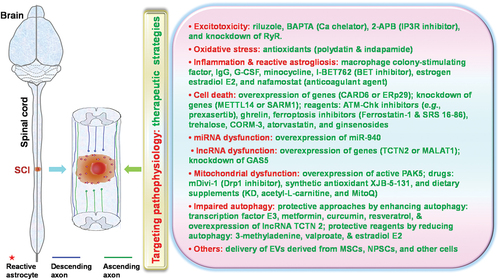
2.1. Excitotoxicity and oxidation
2.1.1. Riluzole
SCI disrupts cellular ionic homeostasis, including Na and Ca influx, and causes multiple pathophysiological changes, such as excitotoxicity, oxidative stress, mitochondrial dysfunction, cell edema, and cell death. Riluzole is an FDA-approved Na channel blocker used to treat neurodegeneration in amyotrophic lateral sclerosis. Preclinical studies with rodent SCI and other models indicate that treatment with riluzole protects neural cells by reducing glutamate release and excitotoxicity after CNS lesions [Citation8]. A phase I clinical trial in cervical SCI patients supported its safety and motor improvement 3 months after treatment [Citation9]. These promising results prompted the extended ongoing-phase II/III trials for SCI patients (NCT01597518).
2.1.2. Reagents to block Ca influx or release into intracellular space
Increase in intracellular Ca due to its entry from extracellular space and/or release from intracellular organelles after CNS injury plays a major role in many pathophysiological cascades, including excessive glutamate release from the intracellular space and excitotoxicity to neural cells due to over-activation of glutamate receptors. Treatment with levetiracetam, an FDA-approved antiepileptic drug, could enhance glutamate uptake from the extracellular space and neuronal and oligodendrocyte survival, reduce lesion cavity size, and improve motor functional recovery in SCI rats [Citation10]. Levetiracetam might act as a presynaptic Ca channel blocker to reduce neurotransmitter release, including glutamate. Intracellular Ca chelator, BAPTA, may protect neural cells from toxic Ca overload and reduce cell death. Local BAPTA application to lesioned spinal cord preserved tissues and promoted behavioral recovery in SCI mice [Citation11]. Because the release of Ca from organelles through IP3 receptors (IP3R) and/or ryanodine receptors (RyR) contributes to cell damage after SCI, knockdown of IP3R3 or treatment with 2-aminoethoxydiphenyl borate, a specific IP3R inhibitor, could also attenuate axon Ca levels and damage 24 hr post-SCI [Citation12].
2.1.3. Antioxidants
Polydatin, a natural precursor of resveratrol, provided neuroprotection in SCI rodents through various mechanisms, including the suppression of oxidative stress, inflammation, mitochondrial dysfunction, and apoptosis [Citation13]. Indapamide, an antioxidant drug, reduced oxidative stress and axon/myelin loss in lysolecithin-induced demyelination in mice. Because indapamide is an FDA-approved thiazide-like diuretic drug used to treat heart failure and hypertension, it will be interesting to assess its potential for treating SCI [Citation14].
2.2. Inflammation and immune responses
SCI activates resident microglia, which secrete proinflammatory cytokines and induce infiltration of neutrophils, macrophages, and immune cells into the injured area. SCI-induced inflammatory responses have diverse effects on tissue damage and repair after CNS injury. Early activation of microglia may be beneficial because it protects cells and activates glial responses to injury, but sustained activation of microglia may mediate further tissue damage by exacerbating inflammatory responses. Microglia activation after SCI induces various glial responses, including astrocytic proliferation and scar formation. Depleting microglia after SCI aggravates parenchymal immune infiltrates and neural cell loss and disturbs glial scar formation and locomotor recovery [Citation15]. Enhancing microglial proliferation by local treatment with macrophage colony-stimulating factor enhances tissue preservation and functional recovery, indicating neuroprotection provided by activated microglia after SCI. However, transient or delayed depletion of microglia has also been reported to alleviate neuroinflammation and tissue damage, thus enhancing functional recovery [Citation16,Citation17]. It seems important to design therapeutic strategies by targeting time-dependent microglial and astrocytic reactions after SCI.
2.2.1. Intravenous immunoglobulin G (IgG) and granulocyte colony stimulating factor (G-CSF)
Several neuroprotective drugs have been designed to target inflammatory responses initiated shortly after SCI. Some therapies are aimed to balance inflammatory responses by suppressing pro-inflammatory but boosting anti-inflammatory factors after SCI. Intravenous IgG was initially used to treat inflammatory and autoimmune diseases by antibody replacement, but it reduced infiltration of inflammatory cells and levels of cytokines and increased enhanced integrity of blood–brain barrier (BBB) structures around the lesioned spinal cord in rodents [Citation18,Citation19]. G-CSF was previously used in clinical trials for treating neutropenia by stimulating the proliferation and differentiation of bone marrow neutrophils. Recent preclinical studies showed that it improved cognitive function in early Alzheimer’s and functional recovery after SCI [Citation5]. Following a phase I/IIa clinical trial that proved the safety and feasibility of G-CSF in SCI patients, a recent phase III clinical trial failed to show a significant positive effect of G-CSF in primary endpoint, although sub-analyses suggested its potential benefits for specific population [Citation20].
2.2.2. Antibiotic minocycline
Minocycline, a clinically available synthetic tetracycline derivative with anti-inflammatory and antibiotic properties, could reduce tissue damage and improve functional recovery after SCI [Citation21,Citation22], as well as in other disorders (e.g. Huntington’s disease and multiple sclerosis). Minocycline promoted neural repair and locomotor recovery in SCI animals [Citation23]. Minocycline provides beneficial effects by downregulating caspases and suppressing inducible nitric oxide synthase, glutamate excitotoxicity, and inflammation. Minocycline might also alter the gut microbiota and alleviate SCI-associated anxiety. Minocycline treatments after acute SCI changed the gut microbiota composition, returned cytokines/chemokines toward normal levels, and prevented dysbiosis-related anxiety-like behavior in rats [Citation21]. A phase II clinical trial of minocycline for acute cervical SCI patients suggested potential improvement in motor function [Citation24].
2.2.3. Bromodomain and extra-terminal domain (BET) inhibitor, estrogen estradiol (E2), and anticoagulant agents
Systemic treatments with I-BET762, a BET inhibitor, significantly reduced the lesion size 8 weeks after contusion SCI in rats. Bromodomain-containing protein 4 (BRD4), one of the BET proteins, could regulate pro-inflammatory gene transcription after SCI, and its deletion in macrophages eliminated the neuroprotection to the injured spinal cord [Citation25]. BET proteins epigenetically regulate gene transcription and contribute to several pathological conditions, including infections, autoimmunity, and inflammation. E2 protected the injured spinal cord in rodents by reducing inflammation and apoptosis and improving angiogenesis, myelination, and motor function [Citation26,Citation27], although local E2 application to the injured spinal cord by poly(pro-E2) film failed to show beneficial effects [Citation28]. Delayed treatments (2–12 hr after SCI) with nafamostat, a serine protease inhibitor to target thrombin, could inhibit thrombin-mediated BBB breakdown and subsequent peripheral immune cell infiltration [Citation29]. Accordingly, deleting PAR1, a receptor for serine protease thrombin, reduced inflammation and astrogliosis and enhanced locomotor recovery in SCI mice [Citation30,Citation31]. The thrombin–PAR1 interactions have previously been reported to induce inflammatory responses, apoptosis, and glial reactions after various CNS injuries [Citation32].
2.3. Cell death and degeneration
2.3.1. Genes to regulate cell survival
Various cells die after SCI by the processes of necrosis, apoptosis, necroptosis (imitating features of apoptosis and necrosis), and ferroptosis. Many genes that regulate cell death may become therapeutic targets for SCI and potentially other CNS lesions. CARD6 inhibits apoptosis after SCI by inhibiting the release of mitochondrial cytochrome c into the cytosol and caspase‐3 signaling pathway [Citation33]. CARD6 deletion accelerated neuronal death and worsened locomotor functional recovery significantly. Spinal cord transection upregulated ERp29 in the motor cortex and viral over-expression of ERp29 in the motor cortex could reduce apoptotic neuronal loss and enhance corticospinal tract (CST) axon regrowth in rats [Citation34]. ERp29 may promote cell survival by regulating protein folding, trafficking, secretion, and ER homeostasis. The ATM-Chk pathways regulate DNA damage and cell death in various types of cells. Treatments with ATM-Chk inhibitors, including the Chk1 and Chk2 inhibitor prexasertib, provided neuroprotection and promoted functional recovery in rats with dorsal column crush injury at T8 [Citation35].
METTL14, an essential element in the control of mRNA binding and metabolic processes, could suppress the differentiation of hematopoietic progenitor and promote leukemogenesis through mRNA m6A modification [Citation36]. Silencing METTL14 repressed apoptosis of spinal cord neurons most likely by inhibiting m6A modification of EEF1A2 and activating the neuronal Akt/mTOR pathway [Citation37]. Systemic treatments with ghrelin, a gastric hormone (also called hunger hormone), could also reduce apoptotic neuronal and oligodendrocyte loss and lesion volume, as well as improve functional recovery in SCI rats [Citation38]. The benefits of ghrelin are most likely associated with multiple molecular mechanisms, such as suppressing mitochondrial cytochrome c release, downregulating the precursor of nerve growth factor (pro-NGF), and activating brain-derived neurotrophic factor (BDNF) dependent Erk signaling.
2.3.2. Ferroptosis inhibitors
Some therapies for SCI target the iron-dependent programmed cell death called ferroptosis. Iron overload triggers ferroptosis, which is characterized by noxious lipid peroxidation, membrane permeability, and consequent cell damage. Ferrostatin-1, a small potent inhibitor of ferroptosis, improved functional recovery in SCI rodents [Citation39]. Intraperitoneal treatments with SRS 16–86, also an inhibitor of ferroptosis, reduced lipid peroxidation products and cell loss and promoted functional recovery in SCI rats [Citation40]. Similarly, systemic trehalose, a sugar consisting of two molecules of glucose, improved the recovery of motor function in SCI mice by inhibiting cellular peroxidation and ferroptosis [Citation41]. CORM-3, a water-soluble complex that can release carbon monoxide, reduced inflammasome signals and pyroptosis-based cell death, while improving motor function recovery in SCI rats [Citation42].
2.3.3. SARM1 and neurodegeneration
It is also interesting to target the genes that control degeneration after axotomy. SARM1, an enzyme with NADase activity, depletes the metabolite NAD+ and mediates Wallerian degeneration in neurodegenerative diseases and after axonal injury, including SCI. Deleting SARM1 in neurons and astrocytes at the early stage after SCI could promote functional recovery in mice by reducing NF-κB-dependent neuroinflammation and facilitating neuronal regrowth [Citation43]. Interactions between SARM1 and ULK1 (an autophagy-associated protein) might partly mediate SARM1-mediated axon damage after CNS injuries [Citation44].
2.3.4. Atorvastatin and ginsenosides
Treatments with numerous compounds, such as atorvastatin (an inhibitor of HMG-CoA), could promote functional recovery in SCI rodents by activating autophagy and suppressing apoptotic cell loss [Citation45,Citation46]. Atorvastatin is a clinical drug used to treat dyslipidemia and prevent cardiovascular diseases. A clinical trial for SCI indicated that atorvastatin failed to improve motor function but reduced pain levels 6 months after treatments [Citation47]. Several traditional medicines, such as ginsenosides in the plant genus Panax (ginseng), provided neuroprotection to the injured spinal cord by suppressing inflammation, oxidative stress, apoptosis, and glial scar formation [Citation48]. The ginsenoside effects have been linked to multiple signaling pathways, including Bax/Bcl2, NOX2/ROS, PI3K/Akt, VEGF, and aquaporin 4.
2.4. Mitochondrial biogenesis
2.4.1. Mitochondrial dysfunction after SCI
Mitochondria supply energy to various cells and are essential for cell metabolism, ionic homeostasis, and survival. Their dysfunction usually induces membrane depolarization, DNA fragmentation, oxidative stress, mitophagy, and impaired calcium homeostasis and dynamics in mitochondria. Mitochondrial defect contributes to multiple pathophysiological events after SCI, including neuronal degeneration, immune response, cell death, and axon regeneration failure [Citation49]. It is attractive to design effective therapies for SCI and other neurological disorders by targeting mitochondrial dysfunction. Researchers have reported various mitochondria-based strategies, including targeting mitochondrial oxidative phosphorylation, ROS production, mitophagy, and biogenesis.
2.4.2. Targeting mitochondrial proteins or transplanting mitochondria
Pharmacological blockade of mitochondrial fissions with mDivi-1, an inhibitor of Drp1, before SCI attenuated caspase-3 release and apoptotic cell loss and enhanced ATP levels and locomotor recovery in rats [Citation50]. Drp1 is a GTPase mediating mitochondrial fragmentation. Repeated mitochondrial fissions (i.e. fragmentation into small particles) contribute to mitochondrial dysfunction and cell damage after SCI. Post-SCI treatment with mDivi-1 could also repress astrocyte activation and astroglial scar formation and preserve lesioned spinal cord in rats [Citation51]. Deleting syntaphilin, a mitochondria-anchoring protein, could improve mitochondrial transport, motor axon regrowth, and functional recovery [Citation52]. Upregulating active P21-activated kinase 5, which suppresses mitochondrial anchoring by phosphorylating syntaphilin, stimulated CST sprouting after unilateral pyramidotomy [Citation53]. Treatment with XJB-5-131, a synthetic antioxidant used to reduce oxidative damage to mitochondrial DNA, could decrease tissue damage, cell death, and motor deficits in adult rats with contusive SCI at T10 [Citation54]. Some researchers isolated exogenous mitochondria and transplanted them to the lesioned spinal cord for repair. Although transplanted mitochondria sustained acute bioenergetics in the injured spinal cord, they failed to promote tissue repair and functional recovery [Citation55].
2.4.3. Dietary supplements and other approaches
Some dietary supplements could facilitate recovery after SCI by improving mitochondrial function. Acetyl-L-carnitine treatments could boost mitochondrial function and decrease neuronal degeneration and cell death in SCI rodents [Citation56,Citation57]. Carnitine, a quaternary ammonium compound found in almost all cells, has a critical role in transporting long-chain fatty acids across the inner mitochondrial membrane. It also helps with synthesizing acetyl-CoA and boosting glutathione levels in tissues. Post-SCI intraperitoneal MitoQ, a synthetic analog of antioxidant coenzyme Q10, could improve angiogenesis and tissue repair of the lesioned spinal cord and mitochondrial function in mice. MitoQ increases mitochondrial penetration and bioavailability compared to coenzyme Q10.
Ketogenic diet (KD) treatments showed multiple benefits in SCI rodents, including improved anabolic steroids and mitochondrial biogenesis, reduced inflammation and cell death, and enhanced tissue repair and functional recovery [Citation58,Citation59]. KD is characterized by high fat (80–90%), low carbohydrate, and proper protein consumption and it can improve the production of ketone bodies (i.e. β-hydroxybutyrate) [Citation60]. KD treatment could enhance oxidative metabolism and ATP synthesis in mitochondria and reduce ROS formation [Citation61]. KD combined with ketone sodium could preserve injured axons and promote forelimb motor function in SCI rats [Citation62]. Clinical trials of KD indicated improved ketone body levels and motor functions in patients with SCI [Citation63].
2.5. Autophagy
2.5.1. Autophagy alterations after SCI
Some studies reported neural apoptosis due to reduced autophagy after SCI, but others showed neuronal death due to over-activated autophagy after SCI. Autophagy, a lysosomal degradation process, is essential for sustaining metabolism and homeostasis by removing protein aggregates and impaired organelles. The autophagy levels after SCI are dynamic and largely depend on the time course and types of injury. Early (within several days after SCI) damage to the spinal cord may activate autophagy around the lesioned spinal cord. Autophagy could be neuroprotective and favor functional recovery after SCI overall [Citation64], although over-activation of autophagy may induce cell death. Severe SCI impaired autophagy, but a moderate injury boosted it [Citation65,Citation66]. Activated autophagy after a moderate SCI may promote recovery by eliminating broken organelles and toxins in injured cells [Citation67]. Various therapies to target autophagy yielded inconsistent results in SCI models [Citation68].
2.5.2. Therapies to augment autophagy
Some reagents used to induce autophagy showed neuroprotection after SCI. Metformin, a clinical drug for treating type 2 diabetes, promoted autophagy, inhibited neuronal apoptosis, and improved functional recovery in SCI rodents, most likely by inhibiting the mTOR pathway [Citation69]. Curcumin, the major curcuminoid of turmeric, could also augment autophagy, inhibit oxidative stress, inflammatory responses, and apoptotic neuronal loss, and improve tissue repair and functional recovery also by inhibiting the Akt/mTOR and NF-κB pathways [Citation70,Citation71]. Resveratrol, a type of natural phenol enriched in many fruits, could support autophagy and functional recovery in SCI rodents through the AMPK/SIRT1 signaling pathway [Citation72]. The benefits of resveratrol might be associated with the upregulation of anti-apoptotic proteins (e.g. Bcl-2) and the downregulation of pro-apoptotic factors (caspase-3, caspase-9, and Bax) in SCI rodents [Citation73]. Activation of transcription factor E3, a main regulator of lysosomal biogenesis, boosted autophagy, inhibited cell loss, and promoted function recovery in SCI rodents [Citation74,Citation75]. Upregulating long non-coding RNA (lncRNA) TCTN2 could also enhance autophagy, suppress neuronal apoptosis, and promote recovery in SCI rats (see Section 4.3) [Citation76].
2.5.3. Therapies to suppress autophagy
In contrast, other studies showed that inhibiting autophagy was neuroprotective after SCI. 3-Methyladenine, the adenine substituted with a methyl group at position N-3, is frequently used as a PI3K and autophagy inhibitor. Its treatment could repress autophagosome formation and neurodegeneration and improve spontaneous functional recovery in SCI rodents [Citation77]. Valproate, a clinical drug mainly used to treat epilepsy and bipolar disorder, and E2 could also inhibit autophagy, prevent neural tissue damage, and enhance neurological function in SCI animals [Citation78,Citation79]. Notably, E2 has multiple effects on the damaged spinal cord, as discussed in section 2.2.3. Autophagy inhibitor spautin 1 increased the survival of cultured oligodendrocyte progenitor cells (OPCs), but treatments with either autophagy activator rapamycin or inhibitor spautin 1 did not affect locomotor recovery in mice with contusive SCI at T9 [Citation80].
2.6. Extracellular vesicles (EVs)
2.6.1. EVs and their functions
EVs have become attractive targets for designing molecular therapies for SCI and other neurological disorders. EVs are membrane-bounded particles secreted by various cell types, and they carry different bioactive biomaterials (e.g. proteins, lipids, and nucleic acids). EVs mediate important physiological and pathophysiological functions in CNS and other tissue systems by transporting cell-specific signaling cargoes, such as signal proteins and lipids, cytokines, and genetic information. EVs modify the function of targeted cells and affect the development of secondary lesions after SCI. Stimulation by ATP or proinflammatory factors (e.g. IL-1β, TNF-α, and IFN-γ) induces the release of various cytokines from microglia-derived EVs and mediates inflammation and reactive glial responses [Citation81]. EVs from reactive astrocytes might mediate progressive tissue damage after SCI by releasing small GTP-binding proteins (e.g. profilin-1, FSCN1, and destrin), cytokines (e.g. IL-1β), pathogen-expressed proteins (e.g. Nef), and miRNAs. EVs may also inhibit or facilitate axon regeneration by releasing axon growth inhibitors (e.g. Nogo A) or axon elongation promoters (e.g. retinoic acid receptor β) [Citation81,Citation82].
2.6.2. Treatments with EVs derived from MSCs
Many researchers attempted to develop EV-based cell-free therapies for SCI using the bioactivity and inherent biocompatibility of EVs. The cell source of EVs is one of the major determinants for designing EV-based strategies for treating CNS injury. EV-deriving cells usually control the contents of EVs, including cytosolic and cell-surface proteins and nucleic acids accessible for packaging into EVs during EV biogenesis. The producer cells also largely determine the types of small non-coding RNAs (ncRNAs) and miRNAs inside EVs. Given the frequent use of MSC transplants for CNS injury studies, the therapeutic potential of EVs derived from MSCs has been evaluated in animals with SCI. MSC-EVs could reduce lesion cavity and promote microvascular regeneration and functional recovery in SCI mice [Citation83]. Intravenous EVs derived from human umbilical cord MSCs could diminish inflammation and scar response in SCI rats [Citation84]. However, MSC-EVs failed to stimulate axon regeneration, neurogenesis, and neuronal reconnections after SCI [Citation81]. Because EVs can protect nucleic acids and other bioactive elements from degradation and elimination, MSC-EVs modified to overexpress therapeutic miRNAs (e.g. miR-124 and miR-133b) could enhance functional recovery in rats with SCI or brain injury [Citation85,Citation86]. Systemic EVs derived from miR-26a-modified MSCs might attenuate glial scarring and enhance neurogenesis and axon regeneration by activating PI3K/Akt signaling [Citation87].
2.6.3. Treatments with EVs derived from NSCs or other sources
Intrathecal or systemic EVs derived from NSCs could also alleviate pro-inflammatory cytokines, regulate autophagy, preserve lesioned spinal cord, and improve angiogenesis and functional recovery. The benefits of NSC-EVs have been linked to their enrichment of 14-3-3 proteins and VEGF and their repression of NLRP3 inflammasome complexes [Citation83,Citation88]. Moreover, EVs derived from cortical neurons could supply neuroprotective miR-124-3p and suppress microglia-mediated neuroinflammation and detriments of reactive astrocytes in SCI mice [Citation89]. EVs derived from M2 microglia could reduce neuronal loss by supplying pyroptosis-preventing miR-672-5p and promote axon regeneration and functional recovery in SCI mice [Citation90].
3. Axon regeneration
Disconnecting axonal tracts between the rostral and caudal spinal cord largely contributes to life-long functional loss in most SCI patients. Developing strategies for stimulating axon regrowth, regeneration, reconnection, and restoration of signal conduction between rostral and neurons is crucial for treating SCI [Citation91]. Because decreased intrinsic growth capacity of mature neurons and a non-permissive environment around the lesion are largely responsible for the failure of axon regeneration and repair in adult mammalian CNS [Citation92], numerous researchers attempted to discover therapeutic strategies that target these and other mechanisms ().
Figure 2. Major recently reported molecular strategies for promoting axon regeneration and neuronal relays after central nervous system (CNS) injuries. Various molecular strategies have recently been reported to stimulate axon regeneration and neuronal relays after spinal cord injury (SCI) or other CNS lesions, including those that target either neuronal intrinsic factors or extrinsic factors around the lesioned spinal cord.
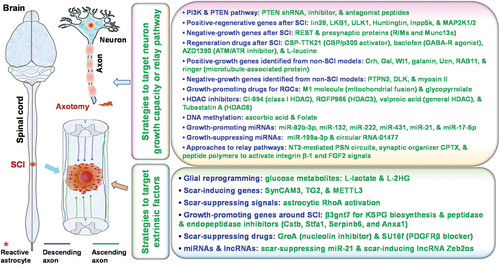
3.1. Targeting genes to enhance intrinsic growth capacity
3.1.1. PI3/Akt/PTEN pathway
Mature neurons largely lose their growth ability after axotomy, and numerous signaling pathways are linked to the low intrinsic growth capability. Among them, the PI3/Akt/PTEN pathway is important for controlling the regrowth of adult CNS neurons. Deleting or inhibiting PTEN, a negative regulator of mTOR, could enhance axon regeneration after SCI and other CNS injuries. PTEN knockdown by short-hairpin RNA (shRNA) or blockade by pharmacological approaches demonstrates various degrees of axon regrowth in adult rodents with CNS injuries [Citation93–95]. Systemic treatments with a PTEN antagonist peptide in adult rats with cervical SCI promoted extensive long-distance regeneration of injured rostral ventral respiratory group (rVRG) axons through the lesion, formed putative excitatory synaptic connections with phrenic motor neurons, and promoted recovery of diaphragmatic respiratory function [Citation96]. Impressively, systemic PTEN antagonist peptide treatments starting 8 weeks after cervical SCI still promoted substantial, long-distance regeneration of injured bulbospinal rVRG axons into and through the lesion and stimulated moderate recovery of diaphragm function [Citation97]. Similarly, PTEN deletion at one-year post-SCI induced modest regrowth of motor and sensory axon populations in knockout mice [Citation98]. PTEN knockdown with shRNAs has also been used to promote axon regrowth after SCI. Local injection of AAV-shRNA-PTEN into the sensorimotor cortex could enhance CST regeneration and skilled motor function of the upper extremities in rats with C5 dorsal hemisection SCI. Combined rehabilitative training with PTEN knockdown might further promote axon regeneration, synaptic reorganization, and improvement in forelimb skilled motor function after cervical SCI [Citation99]. Recently, retrograde AAV-shRNA-PTEN vectors have been designed to target motor neurons in cortices and subcortical regions in SCI rodents [Citation100]. Transcriptional activator Elk-1 promoted retinal ganglion cell (RGC) survival and axon regeneration in vivo downstream of PTEN signaling [Citation101]. Another transcriptome study suggested that Annexin A2 (a Ca-dependent phospholipid-binding protein) and its downstream ILK and MPP1 might mediate RGC growth and survival also through the PTEN pathway [Citation102].
3.1.2. Lin28 mRNA binding protein
It is very promising to promote CNS axon regeneration by targeting lin28, an RNA-binding protein that can bind and block let-7 miRNA. Upregulating lin28a before SCI in transgenic mice or after SCI with an AAV vector promotes robust regeneration of CST axons in adult mice [Citation1]. Upregulating lin28a also enhances significant recovery of locomotor function in adult mice with SCI. Moreover, upregulating lin28a stimulated robust and persistent regeneration of injured optic nerves in mice [Citation1,Citation103,Citation104]. Lin28 upregulation activated multiple neuronal pathways, including Akt signaling [Citation1,Citation103] although one recent study supported that amacrine cells, the inhibitory neurons that innervate RGCs, were the major cellular targets of lin28 in regulating the regeneration of axotomized RGCs [Citation104]. Lin28a overexpression combined with non-muscle myosin IIA/B deletion promoted additional long-distance axon regeneration in mice with optic nerve crush (ONC). Suppressing myosin II reorganizes actin and microtubules in growing axons and facilitates axon extension over the inhibitory substrates (CSPGs and myelin inhibitors) in vitro [Citation105] and may promote axon regeneration after CNS injury.
3.1.3. LKB1 master kinase and ULK1
A recent study supports that LKB1, known as a master kinase of AMPKs and AMPK-related kinases, is critical in promoting the regenerative ability of mature CNS neurons [Citation2]. Upregulating LKB1 by intracortical or systemic AAV vectors promoted dramatic regrowth of descending CST axons in adult mice. Treatments with AAV vectors for LKB1 also stimulated significant locomotor functional recovery in SCI mice [Citation2]. LKB1 may thus become an important molecular target for promoting neural repair, axon regeneration, and functional recovery in adult mammals. Inhibiting ULK1, a well-known kinase regulating autophagy, could protect neurons from neurodegeneration and stimulate regeneration of axotomized neurons. Upregulating a dominant-negative form of ULK1 by AAV vector could enhance sprouting of 5-HT and tyrosine hydroxylase fibers in the lesioned spinal cord, and regrowth of crushed optic nerve in rats [Citation106]. The ULK1-mediated axon regrowth has been linked to Erk activation and RhoA inhibition.
3.1.4. Huntingtin gene
A recent study indicated the critical role of Huntingtin gene in maintaining transcriptome activity for regeneration in adult CST neurons in rodents [Citation107]. Both SCI alone and SCI combined with neural precursor cell grafts induced early transcriptomic responses in host CST neurons, but only SCI plus neural precursor cell grafts could retain the regenerative transcriptome 2 weeks after injury. Deleting Huntingtin significantly reduced the regenerative capacity of CST neurons after SCI. Informatics analyses in axotomized RGCs also suggest that Huntingtin is a key regulator of RGC regrowth, in addition to mTOR and c-myc [Citation108].
3.1.5. Other promising regenerative genes and strategies for SCI
Local injection of peptide amphiphile supramolecular polymers into lesioned spinal cord could stimulate remarkable vascular growth, axonal regeneration, myelination, survival of motor neurons, reduced gliosis, and functional recovery [Citation109]. This study reported the new synthetic nanoscale polymers that contain peptides to activate integrin β-1 and basic FGF2 receptors. These biomaterials preserve biological signals of two receptors at the same extent but slightly mutate the tetrapeptide sequence of targeted domains, thus exhibiting great therapeutic potential for severe CNS injury. Treatments with epothilones, the natural compounds that could stabilize microtubules, increased axon regrowth and locomotor recovery of hindlimbs in SCI rats, and a combination of epothilone B with rehabilitation showed complementary effects on functional recovery [Citation110].
When mice with transection SCI were housed in an enriched environment, treatment with CSP-TTK21, a CBP/p300 activator, between 12 and 22 weeks after SCI stimulated motor and sensory axon growth, sprouting, and synaptic plasticity, but did not promote sensorimotor recovery [Citation111]. A recent genomics study indicated that REST might be an upstream suppressor of a pro-regenerative gene program associated with CNS axon regeneration [Citation112]. Upregulating Inpp5k, an enzyme to remove the phosphate on position 5 of inositol rings, could stimulate CST axon regrowth after pyramidotomy, stroke, and acute and chronic contusion injuries [Citation113]. A genetic study showed that some synaptic vesicle priming proteins in the presynaptic active zone, including RIMs and Munc13s, suppressed the axon growth of adult neurons by activating voltage-gated Ca channels [Citation114]. Systemic treatment with Baclofen, a GABA receptor agonist, could reduce voltage-dependent Ca influx in sensory neurons and promote their regeneration after SCI. PARP1 was upregulated by several growth inhibitors or CNS injury and its deletion or inhibition has been shown to facilitate axon regeneration of CNS neurons [Citation115,Citation116]. However, a recent study showed that PARP deletion or inhibition by systemic veliparib failed to induce axon regrowth and functional recovery in mice with either SCI or ONC [Citation117].
Several other reagents, such as the ATM/ATR inhibitor AZD1390 and an essential amino acid L-leucine, might also promote axon regeneration and functional recovery in SCI rodents [Citation118,Citation119]. DLK and LZK mediated axon regrowth in injured neurons and the sprouting of uninjured neurons in mice, indicating that they may become potential targets for neural repair after SCI [Citation120]. Repetitive transcranial magnetic stimulation of the brain stimulated CST axon regrowth by activating MAP2K1/2 signaling, suggesting that modulating MAP2K1/2 activities may alter the growth capacity of mature CNS neurons [Citation121].
3.1.6. Regenerative genes identified from RGC and other models
It will be interesting to study regeneration-associated genes recently identified from other cell types. A single-cell transcriptomic study from different RGC types has identified multiple genes to regulate RGC survival and regeneration after ONC, including CRH, GAL, WT1, galanin, and UCN [Citation122]. Upregulating these proteins or neuropeptides promoted significant axon regeneration of RGCs after axotomy. Upregulating protrudin, a scaffold protein, could boost the accumulation of integrins, RAB11 endosomes, and ERs in axons and promote RGC axon regeneration [Citation123]. PTPN2 inhibition combined with low-dose IFNγ enhanced regeneration of CNS axons through the cGAS-STING downstream pathway by amplifying IFNγ-STAT1 signaling [Citation124]. Local treatment with M1, a small molecule that potentially promotes mitochondrial fusion and transport, enhanced in vivo axon regeneration of peripheral neurons, injured RGCs, and visual function in mice with ONC [Citation125]. This group also reported that treatments with the small-molecule glycopyrrolate, an FDA-approved drug used to treat excessive sweating, promoted peripheral nerve regeneration, RGC survival, and sustained functional RGC regeneration in mice with ONC [Citation126]. In C. Elegans, DLK-mediated injury signaling might activate autophagy, which suppressed axon regeneration by reducing the level of LIN-12 and NOTCH proteins [Citation127]. A transcriptome profiling study in fly sensory neurons suggested the expression of ringer, a microtubule-associated protein, in sensory neurons before and after injury and its requirement for axon regeneration following the deletion of Rtca, a protein that regulates RNA metabolism [Citation128].
3.2. Extrinsic factors as targets for regeneration
Many regenerative strategies for SCI were designed to target the non-permissive environment around the lesion. In mammals, the lesioned spinal cord usually forms various barriers that block regeneration of injured axons, including inflammatory reactions, lesion cavities, physical glial and fibroblast scars, and numerous inhibitory molecules generated by intense scar tissue. Importantly, reactive glial cells, including astrocytes, upregulate scar-sourced inhibitors of CSPGs around the lesion and strongly prevent axon regrowth. Several inhibitors expressed by oligodendrocytes (e.g. Nogo, MAG, and OMgp) also repress the regrowth of injured spinal axons.
3.2.1. Glial reprogramming
A recent interesting study reported a novel strategy to promote neuronal regeneration and synaptogenesis by glial reprogramming and pioneered the glia–neuron interactions coupled with glycolytic metabolites [Citation129]. Reprogrammed glia by targeting their intracellular pathways, particularly PI3K and EGFR activation, induced significant axon regeneration after CNS injury. Increased generation of lactate and L-2HG, two glycolytic metabolites, in reprogrammed glia, stimulated dramatic axon regeneration by acting on GABAB receptors of axons. Glia programming could also enhance retinal neuron regeneration [Citation130]. Upregulating Ascl1 specifically in Müller glia combined with suppressing histone deacetylases (HDACs) facilitates Müller glia to generate retinal neurons in adult mice with retinal injury [Citation131].
3.2.2. Extracellular matrix (ECM) proteins and scar formation
Some strategies that target ECM regulators of scar formation stimulated axon regeneration after SCI. Synaptic cell adhesion molecules (SynCAMs) seem important in regulating recovery in SCI rodents by enhancing astrocytic scar formation [Citation132]. Deleting SynCAM3 prevented the transformation of reactive astrocytes into scar-forming astrocytes, reduced scar tissue, and improved functional recovery in SCI mice by reconstituting several ECM-related proteins, including type I collagens. A recent study on axon regeneration in spiny mice (Acomys) identified that B3gnt7, a key enzyme of keratan sulfate biosynthesis, enhanced dramatic axon regrowth by rewiring glycosylation biosynthetic pathways in the lesioned mouse spinal cord [Citation133], suggesting that B3gnt7 may facilitate the creation of a regeneration-promoting environment by depositing keratan sulfate proteoglycans around the lesioned spinal cord. TG2, a Ca-dependent enzyme that regulates cell adhesion, ECM stabilization, and wound healing, could control astrocytic scar formation after SCI [Citation134]. Selective TG2 deletion in astrocytes reduced astrocytic scarring in mice with contusion SCI. Notably, intracellular TG2 expression could promote neuronal survival after ischemia [Citation135]. Moreover, the methyltransferase activity of METTL3 supported reactive astrogliosis and neural repair after SCI because conditional deletion of this gene selectively in astrocytes exacerbated neural damage around the lesion and impaired functional recovery [Citation136].
3.2.3. Cell growth factors
Treatment with the nucleolin inhibitor, GroA, could inactivate EGFR, suppress scar formation, and improve the prognosis of SCI [Citation137]. Nucleolin, a nucleolar protein, interacts with and activates EGFR. EGFR activation mediates glial scar formation, and EGFR inhibitors reduce astrogliosis and improve axon regeneration and functional recovery in adult rats [Citation138]. PDGFRβ contributed to fibrotic scar formation and treatment with its blocker, SU16f, could suppress fibroblast proliferation and fibrotic scar formation and enhance 5-HT axons and locomotor recovery in SCI mice [Citation139].
3.2.4. RhoA as a scar regulator
Neuronal RhoA is a major intracellular signal downstream of multiple inhibitors for axon growth, and it restrains axon growth by targeting various axonal cytoskeletal proteins, including activating myosin-II-mediated actin arc formation in the growth cone [Citation140]. However, RhoA activation in astrocytes was recently shown to reduce astrogliosis through myosin II, independent of microtubules by activating YAP1 signaling [Citation141], suggesting the importance of cell-specific targeting RhoA for CNS repair. Notably, a previous study suggested that local inhibition of RhoA with C3 transferase reduced scar tissue formation in SCI rats [Citation142].
3.2.5. Homeostatic microglia-associated genes
An interesting study demonstrated that homeostatic microglia (i.e. MG3 subset), which were derived from initially activated microglia (MG1), were essential for scar-free wound healing after SCI in neonatal mice [Citation143]. In neonatal SCI mice, microglia-derived fibronectin could form the initial step for tissue bridging and wound healing. MG3 cells upregulated several genes encoding inhibitors of peptidases and endopeptidases (e.g. Cstb, Stfa1 Serpinb6, and Anxa1), which might mediate rapid inflammation resolution. Transplanting microglia treated with two chemical proteinase inhibitors, E64 and serpinA3, to adult SCI mice facilitated the reestablishment of MG3 cells and a permissive environment for axon regrowth.
4. Epigenetic factors, miRNAs, and long RNAs
Epigenetic regulators may become molecular targets for repairing the injured spinal cord (). Epigenetic factors, such as histone modifications, chromatin remodeling, DNA methylation, and ncRNAs, may regulate cell survival and neuronal growth by modifying the transcription and expression of heritable DNAs. Small non-coding miRNAs negatively regulate gene expression at the post-transcriptional level and could affect molecular pathways that regulate axon regeneration, inflammation, apoptosis, and remyelination after CNS injuries.
4.1. Histone modifications
Histone acetylation by histone acetyltransferases (HATs) and deacetylation by HDACs may regulate gene transcriptions and neuronal growth. HATs add acetyl groups to lysines and result in exposed chromatin for gene transcription, while HDACs condense chromatin and suppress genes by removing acetyl groups. HAT activation and HDAC inactivation may enhance cell growth programs and neuronal regeneration. Treatments with several HDAC inhibitors, including CI-994 (a class I HDAC inhibitor), RGFP966 (an HDAC3 inhibitor), and valproic acid (a general HDAC inhibitor), could stimulate neuronal repair and functional recovery after SCI [Citation144,Citation145]. Pharmacologically inhibiting HDAC6 by Tubastatin A could promote functional recovery in SCI mice probably by increasing axonal autophagy and skeletal dynamics [Citation146].
4.2. DNA methylation and RNA modification
It is interesting to promote regeneration by modifying DNA methylation. Ectopic expression of three transcriptional factors, Oct4, Sox2, and Klf4, in mouse RGCs, restored youthful DNA methylation patterns and transcriptomes, promoted axon regeneration after injury, and reversed vision loss in glaucoma mice and aged mice [Citation147]. The benefits of upregulating these genes probably required the DNA demethylases Tet1 and Tet2. Treatments with ascorbic acid (100 mg/kg) upregulated Tet1, Tet2, and Tet3, increased hydroxymethylcytosine (5hmC) levels in the motor cortex, and promoted axonal sprouting around the lesioned spinal cord and locomotor recovery in SCI rats [Citation148]. DNA methylation catalyzed by Tet1 was also required for myelin repair in young adult mice. Inducible Tet1 deletion in OPCs mimics the remyelination failure detected in old mice [Citation149]. Folate supplementation in F0 mating rodents increased regeneration of injured spinal axons in F1-4 generations of progeny in absence of folate administration to the progeny, suggesting transmission of the enhanced regeneration phenotype to progeny through DNA methylation [Citation150]. Also, RNA modifications, including m6A, could regulate RNA stability, protein translation, and probably axon regeneration [Citation151].
4.3. miRNAs and long RNAs as targets for neuroprotection
Some miRNAs and long RNAs may become therapeutic targets for SCI by regulating cell survival and other cellular functions. CNS injury alters the expression levels of many miRNAs, which may target numerous genes and alter cell functions, including cell differentiation and proliferation, immune responses, and cell survival. SCI downregulates some miRNAs (e.g. miR124, miR129, miR1, and miR-940), but upregulates others (e.g. SNORD2) [Citation152]. SCI decreased levels of miR-940, and locally overexpressing miR-940 in lesioned spinal cord suppressed inflammation and promoted functional recovery in mice [Citation153]. Upregulation of miR-7b-3p in mice with cervical SCI appeared to protect neurons from apoptosis [Citation154].
Knockdown of X–inactive specific transcript, a long-coding RNA (>200 nucleotides), inhibited neuronal apoptosis after SCI by stimulating Akt/mTOR pathway [Citation155]. Several lncRNAs also regulate different cellular processes after SCI, including inflammation, metabolism, and apoptosis. The conserved MALAT1 lncRNA mediated the inflammatory response of microglia and tissue damage in SCI rats [Citation156]. SCI upregulated MALAT1 in the contused epicenter, which was accompanied by activated NF-κB signaling and enhanced TNF-α and IL-1β proinflammatory cytokines. MALAT1 knockdown repressed the levels of TNF-α and IL-1β produced by activated microglia and improved recovery of hindlimb locomotor activity. lncRNAs may also act as competitive endogenous RNAs for miRNAs and block the function of targeted miRNAs. SCI downregulated TCTN2 lncRNA around the lesion in rats [Citation76]. Overexpressing TCTN2 facilitated autophagy and functional recovery potentially by reducing the levels of miR-216b and neuronal apoptosis. In contrast, the knockdown of GAS5 lncRNA suppressed inflammation and apoptotic neuronal loss in SCI mice, likely by inhibiting miR-93 and PTEN signaling [Citation157].
4.4 miRNAs and lncRNAs as targets for axon regeneration
Upregulating miR-92b-3p might promote neuronal growth and functional recovery by suppressing PTEN signaling in SCI rats [Citation158]. Delivery of a cocktail of miR-132, miR-222, and miR-431 using 3D fiber-hydrogel scaffold transplants promoted axon regeneration and functional recovery in SCI rats [Citation159]. Combining these miRNAs with methylprednisolone showed additional effects for promoting functional recovery. Moreover, some miRNAs could affect neuronal growth in vitro. In contrast to decreased neurite growth by miR-199a-3p, miR-21, and miR-17-5p could promote neurite outgrowth of cortical neurons by regulating the PTEN/mTOR or Stat3/Gap43 pathway [Citation160,Citation161]. Knockdown of circular RNA-01477 also increased the neurite length of cultured neurons by regulating the miR-3075, FosB, and Stat3 signals [Citation162].
Some miRNAs and lncRNAs could alter reactive glial responses and scar formation and become therapeutic targets for SCI. SCI upregulates miR-21, which may inhibit neuroinflammation, improve BBB integrity and angiogenesis, and regulate glial scar formation. Selectively upregulating miR-21 in astrocytes could reduce astrocytic hypertrophic response to SCI [Citation163] and control polarization of reactive astrocytes and glial scar progression in SCI mice by modulating TGF-β signaling [Citation164]. Analyses of lncRNA expression profiles in lesioned mouse spinal cord indicated that SCI also upregulated lncRNA Zeb2os and its antisense protein Zeb2 in reactive astrocytes [Citation165]. The conversed lncRNA Zeb2os may have a significant role in reactive astrogliosis after SCI because its knockdown reduced reactive astrogliosis in SCI mice.
5. Restoration of relay pathways and neuroplasticity
5.1. Relay pathways by propriospinal neurons (PSNs)
PSN interneurons have become attractive cellular targets for restoring neuroplasticity and function after incomplete SCI by remodeling neural circuits. PSNs could form relay neuronal circuits and facilitate functional recovery after CNS injuries, including SCI. Particularly, PSNs could relay the networks, bypass the lesion, and form detour neuronal circuits in rodents after incomplete SCI. Numerous groups have attempted to reorganize various descending and ascending axonal tracts with local interneurons and improve functional recovery after SCI. Recombinant viral vectors are frequently used to dissect and confirm the functional recovery of PSNs after SCI. Injection of retrograded AAV-NT3 into sciatic nerves promoted recovery in T9 SCI mice largely by reorganizing circuits between PSNs and motor neurons at lumbar levels [Citation166] and enhancing sprouting and synaptic formation of descending serotonergic, dopaminergic, and PSN axons on lumbar motor neurons [Citation167]. Using the AAV vector of CNO-initiated excitatory DREADD, another group reported that chronic activation of lumbar PSNs promoted the hindlimb stepping recovery in mice with bilateral hemisection SCI, further confirming the PSN-based restoration of motor function after SCI [Citation168]. Notably, a recent study suggested a harmful role of long ascending PSNs that link the upper and lower spinal enlargements because silencing spared ascending PSNs post-SCI could improve locomotor function, including paw placement order and timing, and the left–right hindlimb coordination [Citation169].
5.2. Neuroplasticity by collateral pruning and synaptic links
Neuropilin-1, a membrane-bound coreceptor to a tyrosine kinase receptor for VEGF and semaphorin, might contribute to the pruning of neuronal collaterals for motor recovery after partial SCI. Neuropilin-1 knockdown or deletion by local AAV vectors suppressed the recovery of skilled movement 3 and 4 weeks after hemisection SCI in mice [Citation170]. An interesting study reported a synthetic synaptic organizer called CPTX which combined structural elements from cerebellin-1 and neuronal pentraxin-1 [Citation171]. CPTX could interact with presynaptic neurexins and postsynaptic AMPA-type ionotropic glutamate receptors and induce the formation of excitatory synaptic links across the synaptic gap, thus restoring neuronal reconnections. They reported that local CPTX could rapidly induce the formation of excitatory synapses and functionally restore neuronal circuits for functional recovery in several mouse models, including SCI, ataxia, and Alzheimer’s disease.
6. Concluding remarks
Clinical treatments for SCI are currently unavailable, and there is a persistent need for developing highly effective strategies for repairing injured spinal cord. In the past several years, scientists have further advanced our understanding of the pathophysiology of SCI and identified new preclinical therapies for SCI by targeting diverse cellular and molecular pathophysiological changes. Some promising strategies target secondary injury mechanisms (e.g. various types of cell death, inflammation, energy biogenesis, and scar reactions) in acute SCI for neuroprotection, aiming to reduce continuing lesions. Timely controlling microglial and astrocytic reactions appears important for the design of effective strategies for SCI. Various miRNAs, long RNAs, and EVs have also become attractive therapeutic targets for SCI. The treatments for subacute and chronic SCI focus on neural repair, regeneration, synaptic reconnections, and rehabilitation. Numerous genes are critical for controlling the growth capacity of mature neurons, such as lin28, LKB1, Huntingtin, and some transcriptional factors. Many strategies that target extrinsic factors are also very promising for SCI repair, including metabolic reprogramming of glia and targeting ECM-related B3gnt7 and genes upregulated in homeostatic microglia. Most reagents and genes studied have multiple molecular targets and most likely facilitate repair and recovery through complicated pathways. Some compounds are FDA-approved clinical drugs or dietary supplements used in humans, and they may have greater translational potential for treating SCI. Notably, this review focuses on major progress in molecular strategies, but many researchers employed other approaches to improve recovery after SCI, including transplants of various types of cells or biomaterials, electrical and magnetic stimulations, intermittent hypoxia, task-specific rehabilitation training, and combined therapies.
7. Expert opinion
Scientists have recently reported promising molecular targets and therapies for SCI, but many critical issues remain in developing highly effective and translational therapies for SCI patients. Most reported strategies exhibited limited and moderate effects by targeting individual mechanisms, and their translational potential for human use is questionable. It is crucial to identify highly effective strategies for CNS repair and reproduce promising results with distinct model systems, including the use of different animal species and translational models. Most therapeutic strategies were evaluated with acute SCI models, but a few of them were reported to be effective in chronic SCI, such as systemic PTEN antagonist peptide and intraventricular pyruvate kinase isoform M2 [Citation97,Citation172]. It is very important to validate the effectiveness of additional highly effective therapies in subacute and chronic phases of SCI.
Recent transcriptomic studies using various types of neurons revealed numerous new molecular targets for neuroprotective and/or regenerative interventions. With the interesting new targets, researchers may identify more effective molecular therapies for SCI and other neurological disorders. Because gene and drug therapies usually have multiple molecular and cellular targets, targeting each of them most likely has complicated cellular function and significance. Combined approaches that affect different injury mechanisms for neuroprotection and neuronal regrowth may exhibit synergistic actions for repairing the injured spinal cord. Influencing multiple molecular pathways that control both intrinsic and extrinsic factors for neuronal growth should stimulate more robust and sustaining regeneration after SCI. Molecular therapies combined with other effective non-pharmacological strategies, such as transplants of cells or biomaterials to bridge the lesion gap and rehabilitative electrical/magnetic stimulation and task-dependent training, should further improve tissue repair and functional recovery.
Because axon disconnections principally induce functional deficits after SCI, axon regeneration and rewiring of impaired neural circuits around the lesion are essential for restoring lost function. Regulating certain genes identified could promote regeneration and/or sprouting regrowth, but it is extremely important to discover additional highly effective strategies for stimulating more robust and sustained regrowth in adult CNS neurons. Targeting multiple factors for neuronal growth failure potentially helps achieve long-distance regeneration of axotomized CNS axons. Because the growth status of adult neurons is time-dependent after SCI [Citation107], triggering neuronal growth programs during a particular period after SCI could be more efficient for achieving successful axon regeneration. Guiding regenerating axons to functionally meaningful neurons for proper synaptogenesis also seems important for successful CNS repair. Any strategies that facilitate the formation of useful synaptic contacts and circuits between the rostral and caudal spinal cord may enhance functional recovery. Specifically, targeting interneurons, including PSNs, is an alternative attractive method for improving neuroplasticity and functional recovery.
It is very important to translate the most promising strategies, including gene and pharmacological treatments, into clinical trials for SCI. Noninvasive therapies, such as systemic drug treatments, may have greater translational potential than invasive approaches. It is very interesting to study the potential of some clinical drugs for treating SCI. Several FDA-approved drugs used for treating other disorders could promote neural repair and functional recovery in preclinical SCI models by targeting certain signaling pathways. Treatments with NSAID ibuprofen or antiepileptic gabapentin promoted significant axon regeneration and functional recovery in SCI rodents by blocking RhoA and α2δ2 voltage-gated calcium channels, respectively [Citation173,Citation174]. The antiepileptic levetiracetam reduced glutamate excitotoxicity and lesioned size and enhanced motor recovery in SCI rodents [Citation10]. It should be more easily to translate these drugs to clinical trials for treating SCI.
Some promising preclinical studies have triggered the associated clinical trials of SCI. Although most new clinical trials for SCI focus on neuromodulation, electrical stimulation, and rehabilitation, the overall number of SCI clinical trials initiated each year is increasing and some of them focus on gene or drug therapies, including those aiming to provide neuroprotection and regeneration [Citation5,Citation175]. Most animal species used for SCI studies have similar pathobiology to humans and share homologs in molecules, but the distance required for axon regeneration and the types and level of functional recovery after SCI are very distinct between animals and humans. Validation of highly effective strategies with clinically relevant SCI models, such as contusion in primates, probably facilitates their successful translation to human trials. Targeted molecular therapies may also cause off-target effects, which may prevent their future clinical use if they are severe, such as tumorigenesis and serious neuropathic pain. It is very challenging to translate preclinical animal studies to clinical trials, and many of these trials may fail to stimulate functional recovery in patients, but success in some trials, even moderate improvements in motor, sensory, and autonomic functions, may improve the quality of life of SCI patients significantly by providing greater independence for self-care.
Article highlights
Overall review of insight into therapeutic molecular targets in preclinical SCI studies reported in recent years
Update effective neuroprotective strategies for SCI by targeting SCI pathophysiology, including excitotoxicity, oxidation, inflammation, cell death, neuronal degeneration, mitochondrial dysfunction, autophagy, and extracellular vesicles
Review progress in molecular targets for promoting axon regeneration after SCI, including targeting novel genes to enhance intrinsic growth capacity and numerous extrinsic factors to overcome the nonpermissive environment for axon growth
Update strategies that target epigenetic factors, including DNA and RNA modifications, miRNAs, and long RNAs, for treating SCI
Review advances in restoring relay pathways and neuroplasticity by targeting propriospinal neurons and rewiring synaptic connections among neurons
Provide expert opinion on major challenges for the future design of highly effective treatments for SCI and how to translate them into human clinical trials
This box summarizes key points contained in the article.
Abbreviations
AMPK | = | AMP-activated protein kinase |
ANXA1 | = | annexin A1 |
B3GNT7 | = | UDP-GlcNAc:betaGal beta-1,3-N-acetylglucosaminyltransferase 7 |
BBB | = | blood-brain barrier |
BET | = | bromodomain and extra-terminal domain |
CARD6 | = | caspase recruitment domain family member 6 |
CBP | = | CREB binding protein |
cGAS | = | cyclic GMP-AMP synthase |
CNO | = | clozapine N-oxide |
CNS | = | central nervous system |
CORM-3 | = | carbon monoxide releasing molecule 3 |
CRH | = | corticotropin releasing hormone |
CSPG | = | chondroitin sulfate proteoglycan |
CST | = | corticospinal tract |
CSTB | = | cystatin B |
DLK | = | dual leucine zipper kinase |
Drp1 | = | dynamin-related protein 1 |
E2 | = | estradiol |
ECM | = | extracellular matrix |
EEF1A2 | = | eukaryotic translation elongation factor 1 alpha 2 |
EGFR | = | epidermal growth factor receptor |
Elk-1 | = | ETS Like-1 protein |
ER | = | endoplasmic reticulum |
ERp29 | = | endoplasmic reticulum protein 29 |
EV | = | extracellular vesicle |
F1 | = | filial 1 hybrid |
FGF2 | = | fibroblast growth factor 2 |
FSCN1 | = | Fascin actin-bundling protein 1 |
GAL | = | galanin and GMAP prepropeptide |
GAP43 | = | growth associated protein 43 |
GAS5 | = | arrest‑specific transcript 5 |
G-CSF | = | cytokine granulocyte colony-stimulating factor |
HAT | = | histone acetyltransferase |
HDAC | = | deacetylation by histone deacetylase |
HMG-CoA | = | β-Hydroxy β-methylglutaryl-CoA |
IFN-γ | = | interferon gamma |
IgG | = | immunoglobulin G |
IL-1β | = | interleukin-1 beta |
ILK | = | integrin-linked kinase |
IncRNA | = | long non-coding RNA |
Inpp5k | = | inositol polyphosphate-5-phosphatase K |
IP3R | = | IP3 receptors |
KD | = | ketogenic diet |
KLF4 | = | Kruppel-like factor 4 |
L-2HG | = | L-2-hydroxyglutarate |
LKB1 | = | liver kinase B1 |
LZK | = | leucine zipper-bearing kinase |
MAG | = | myelin associated glycoprotein |
Mdivi-1 | = | mitochondrial division inhibitor 1 |
METTL | = | methyltransferase-like |
miRNA | = | microRNA |
MitoQ | = | mitoquinone mesylate |
MPP1 | = | MAGUK P55 scaffold protein 1 |
MSC | = | mesenchymal stem cells |
mTOR | = | mammalian target of rapamycin |
NAD | = | nicotinamide adenine dinucleotide |
NADPH | = | reduced nicotinamide adenine dinucleotide phosphate |
ncRNA | = | non-coding RNA |
Nef | = | negative regulatory factor |
NF-κB | = | Nuclear factor kappa-light-chain-enhancer of activated B cells |
NLRP3 | = | NLR family pyrin domain containing 3 |
NOX2 | = | NADPH oxidase 2 |
NSC | = | neural stem cell |
NT3 | = | neurotrophin 3 |
OCT4 | = | Octamer-binding transcription factor 4 |
OMgp | = | oligodendrocyte-myelin glycoprotein |
ONC | = | optic nerve crush |
OPC | = | oligodendrocyte progenitor cell |
PAR1 | = | proteinase-activated receptor 1 |
PARP1 | = | poly(ADP-ribose)polymerase-1 |
PDGFRβ | = | platelet-derived growth factor β |
PSN | = | propriospinal neuron |
PTEN | = | phosphatase and tensin homolog |
PTPN2 | = | protein tyrosine phosphatase non-receptor type 2 |
RAB11 | = | Ras-related protein Rab-11 |
REST | = | RE1-silencing transcription factor |
RGC | = | retinal ganglion cell |
RIM | = | Rab3 interacting molecule |
ROS | = | reactive oxygen species |
Rtca | = | RNA 3’-terminal phosphate cyclase |
rVRG | = | rostral ventral respiratory group |
RyR | = | Ryanodine receptors |
SARM1 | = | sterile alpha and TIR motif containing 1 |
SCI | = | spinal cord injury |
SERPINB6 | = | serpin family B member 6 |
shRNA | = | short hairpin RNA |
SIRT1 | = | sirtuin 1 |
SOX2 | = | SRY-box transcription factor 2 |
STAT1 | = | signal transducer and activator of transcription 1 |
Stfa1 | = | Stefin A1 |
STING | = | stimulator of interferon genes |
SynCAM | = | synaptic cell adhesion molecule |
TCTN2 | = | tectonic family member 2 |
Tet | = | ten-eleven-translocation enzyme |
TG2 | = | transglutaminase 2 |
TGF-β | = | transforming growth factor-beta |
TNF-α | = | tumor necrosis factor alpha |
UCN | = | urocortin |
ULK1 | = | Unc-51-like autophagy activating kinase 1 |
VEGF | = | vascular endothelial growth factor |
WT1 | = | WT1 transcription factor |
YAP1 | = | Yes1 associated transcriptional regulator |
Declaration of interest
The authors have no relevant affiliations or financial involvement with any organization or entity with a financial interest in or financial conflict with the subject matter or materials discussed in the manuscript. This includes employment, consultancies, honoraria, stock ownership or options, expert testimony, grants or patents received or pending, or royalties.
Reviewer disclosures
Peer reviewers on this manuscript have no relevant financial or other relationships to disclose.
Acknowledgments
We apologize to the authors whose papers are associated with this review but were not cited due to page limitations.
Additional information
Funding
References
- Nathan FM, Ohtake Y, Wang S, et al. Upregulating Lin28a promotes axon regeneration in adult mice with optic nerve and spinal cord injury. Mol ther. 2020 Apr 15;28(8):1902–1917.
- Ohtake Y, Sami A, Jiang X, et al. Promoting axon regeneration in adult CNS by targeting liver kinase B1. Mol ther. 2019 Jan 2;27(1):102–117.
- de Freria CM, Van Niekerk E, Blesch A, et al. Neural stem cells: promoting axonal regeneration and spinal cord connectivity. Cells. 2021 Nov 25;10(12):3296.
- Rodriguez-Barrera R, Rivas-Gonzalez M, Garcia-Sanchez J, et al. Neurogenesis after spinal cord injury: state of the art. Cells. 2021 Jun 15;10(6):1499.
- Hejrati N, Fehlings MG. A review of emerging neuroprotective and neuroregenerative therapies in traumatic spinal cord injury. Curr Opin Pharmacol. 2021 Oct;60:331–340.
- Warner FM, Cragg JJ, Jutzeler CR, et al. Early administration of gabapentinoids improves motor recovery after human spinal cord injury. Cell Rep. 2017 Feb 14;18(7):1614–1618.
- Levi AD, Anderson KD, Okonkwo DO, et al. Clinical outcomes from a multi-center study of human neural stem cell transplantation in chronic cervical spinal cord injury. J Neurotrauma. 2019 Mar 19;36(6):891–902.
- Zhou LY, Tian ZR, Yao M, et al. Riluzole promotes neurological function recovery and inhibits damage extension in rats following spinal cord injury: a meta-analysis and systematic review. J Neurochem. 2019 Jul;150(1):6–27.
- Grossman RG, Fehlings MG, Frankowski RF, et al. A prospective, multicenter, phase I matched-comparison group trial of safety, pharmacokinetics, and preliminary efficacy of riluzole in patients with traumatic spinal cord injury. J Neurotrauma. 2014 Feb 1;31(3):239–255.
- Lima R, Gomes ED, Cibrao JR, et al. Levetiracetam treatment leads to functional recovery after thoracic or cervical injuries of the spinal cord. NPJ Regen Med. 2021 Mar 2;6(1):11.
- Kang KR, Kim J, Ryu B, et al. BAPTA, a calcium chelator, neuroprotects injured neurons in vitro and promotes motor recovery after spinal cord transection in vivo. CNS Neurosci Ther. 2021 Aug;27(8):919–929.
- Orem BC, Rajaee A, Stirling DP. IP3R-mediated intra-axonal Ca(2+) release contributes to secondary axonal degeneration following contusive spinal cord injury. Neurobiol Dis. 2020 Dec;146:105123.
- Fakhri S, Gravandi MM, Abdian S, et al. The neuroprotective role of polydatin: neuropharmacological mechanisms, molecular targets, therapeutic potentials, and clinical perspective. Molecules. 2021 Oct 2;26(19):5985.
- Michaels NJ, Lemmon K, Plemel JR, et al. Aging-exacerbated acute axon and myelin injury is associated with microglia-derived reactive oxygen species and is alleviated by the generic medication indapamide. J Neurosci. 2020 Oct 28;40(44):8587–8600.
- Bellver-Landete V, Bretheau F, Mailhot B, et al. Microglia are an essential component of the neuroprotective scar that forms after spinal cord injury. Nat Commun. 2019 Jan 31;10(1):518.
- Poulen G, Aloy E, Bringuier CM, et al. Inhibiting microglia proliferation after spinal cord injury improves recovery in mice and nonhuman primates. Theranostics. 2021;11(18):8640–8659.
- Li Y, Ritzel RM, Khan N, et al. Delayed microglial depletion after spinal cord injury reduces chronic inflammation and neurodegeneration in the brain and improves neurological recovery in male mice. Theranostics. 2020;10(25):11376–11403.
- Ulndreaj A, Badner A, Fehlings MG. Promising neuroprotective strategies for traumatic spinal cord injury with a focus on the differential effects among anatomical levels of injury. F1000Res. 2017;6:1907.
- Chio JCT, Wang J, Surendran V, et al. Delayed administration of high dose human immunoglobulin G enhances recovery after traumatic cervical spinal cord injury by modulation of neuroinflammation and protection of the blood spinal cord barrier. Neurobiol Dis. 2021 Jan;148:105187.
- Koda M, Hanaoka H, Fujii Y, et al. Randomized trial of granulocyte colony-stimulating factor for spinal cord injury. Brain. 2021 Apr 12;144(3):789–799.
- Schmidt EKA, Raposo PJF, Torres-Espin A, et al. Beyond the lesion site: minocycline augments inflammation and anxiety-like behavior following SCI in rats through action on the gut microbiota. J Neuroinflammation. 2021 Jun 26;18(1):144.
- Wells JE, Hurlbert RJ, Fehlings MG, et al. Neuroprotection by minocycline facilitates significant recovery from spinal cord injury in mice. Brain. 2003 Jul;126(Pt 7):1628–1637.
- Zhang Y, Al Mamun A, Yuan Y, et al. Acute spinal cord injury: pathophysiology and pharmacological intervention (Review). Mol Med Rep. 2021 Jun;23(6):1–8.
- Casha S, Zygun D, McGowan MD, et al. Results of a phase II placebo-controlled randomized trial of minocycline in acute spinal cord injury. Brain. 2012 Apr;135(Pt 4):1224–1236.
- Cerqueira SR, Benavides S, Lee HE, et al. BET protein inhibition promotes non-myeloid cell mediated neuroprotection after rodent spinal cord contusion. Exp Neurol. 2022 Jun;352:114035.
- Zendedel A, Monnink F, Hassanzadeh G, et al. Estrogen attenuates local inflammasome expression and activation after spinal cord injury. Mol Neurobiol. 2018 Feb;55(2):1364–1375.
- Samantaray S, Das A, Matzelle DC, et al. Administration of low dose estrogen attenuates persistent inflammation, promotes angiogenesis, and improves locomotor function following chronic spinal cord injury in rats. J Neurochem. 2016 May;137(4):604–617.
- Gottipati MK, Ellman SAT, Puhl DL, et al. Acute dose-dependent neuroprotective effects of poly(pro-17beta-estradiol) in a mouse model of spinal contusion injury. ACS Chem Neurosci. 2021 Mar 17;12(6):959–965.
- Zhao C, Zhou T, Zhao X, et al. Delayed administration of nafamostat mesylate inhibits thrombin-mediated blood-spinal cord barrier breakdown during acute spinal cord injury in rats. J Neuroinflammation. 2022 Jul 16;19(1):189.
- Kim HN, Triplet EM, Radulovic M, et al. The thrombin receptor modulates astroglia-neuron trophic coupling and neural repair after spinal cord injury. Glia. 2021 Sep;69(9):2111–2132.
- Radulovic M, Yoon H, Wu J, et al. Targeting the thrombin receptor modulates inflammation and astrogliosis to improve recovery after spinal cord injury. Neurobiol Dis. 2016 Sep;93:226–242.
- Rohatgi T, Sedehizade F, Reymann KG, et al. Protease-activated receptors in neuronal development, neurodegeneration, and neuroprotection: thrombin as signaling molecule in the brain. Neuroscientist. 2004 Dec;10(6):501–512.
- Wang JL, Luo X, Targeting LL. CARD6 attenuates spinal cord injury (SCI) in mice through inhibiting apoptosis, inflammation and oxidative stress associated ROS production. Aging (Albany NY). 2019 Dec 16;11(24):12213–12235.
- Liu R, Zhao W, Zhao Q, et al. Endoplasmic reticulum protein 29 protects cortical neurons from apoptosis and promoting corticospinal tract regeneration to improve neural behavior via caspase and Erk signal in rats with spinal cord transection. Mol Neurobiol. 2014 Dec;50(3):1035–1048.
- Taylor MJ, Thompson AM, Alhajlah S, et al. Inhibition of Chk2 promotes neuroprotection, axon regeneration, and functional recovery after CNS injury. Sci Adv. 2022 Sep 16;8(37):eabq2611.
- Weng H, Huang H, Wu H, et al. METTL14 inhibits hematopoietic stem/progenitor differentiation and promotes leukemogenesis via mRNA m(6)A modification. Cell Stem Cell. 2018 Feb 1;22(2):191–205 e9.
- Gao G, Duan Y, Chang F, et al. METTL14 promotes apoptosis of spinal cord neurons by inducing EEF1A2 m6A methylation in spinal cord injury. Cell Death Discov. 2022 Jan 10;8(1):15.
- Lee JY, Chung H, Yoo YS, et al. Inhibition of apoptotic cell death by ghrelin improves functional recovery after spinal cord injury. Endocrinology. 2010 Aug;151(8):3815–3826.
- Ge H, Xue X, Xian J, et al. Ferrostatin-1 alleviates white matter injury via decreasing ferroptosis following spinal cord injury. Mol Neurobiol. 2022 Jan;59(1):161–176.
- Zhang Y, Sun C, Zhao C, et al. Ferroptosis inhibitor SRS 16-86 attenuates ferroptosis and promotes functional recovery in contusion spinal cord injury. Brain Res. 2019 Mar 1;1706:48–57.
- Gong F, Ge T, Liu J, et al. Trehalose inhibits ferroptosis via NRF2/HO-1 pathway and promotes functional recovery in mice with spinal cord injury. Aging (Albany NY). 2022 Apr 10;14(7):3216–3232.
- Zheng G, Zhan Y, Wang H, et al. Carbon monoxide releasing molecule-3 alleviates neuron death after spinal cord injury via inflammasome regulation. EBioMedicine. 2019 Feb;40:643–654.
- Liu H, Zhang J, Xu X, et al. SARM1 promotes neuroinflammation and inhibits neural regeneration after spinal cord injury through NF-kappaB signaling. Theranostics. 2021;11(9):4187–4206.
- Choi HMC, Li Y, Suraj D, et al. Autophagy protein ULK1 interacts with and regulates SARM1 during axonal injury. Proc Natl Acad Sci U S A. 2022 Nov 22;119(47):e2203824119.
- Gao S, Zhang ZM, Shen ZL, et al. Atorvastatin activates autophagy and promotes neurological function recovery after spinal cord injury. Neural Regen Res. 2016 Jun;11(6):977–982.
- Kwon BK, Okon E, Hillyer J, et al. A systematic review of non-invasive pharmacologic neuroprotective treatments for acute spinal cord injury. J Neurotrauma. 2011 Aug;28(8):1545–1588.
- Aghazadeh J, Samadi Motlagh P, Salehpour F, et al. Effects of atorvastatin in patients with acute spinal cord injury. Asian Spine J. 2017 Dec;11(6):903–907.
- Qi L, Zhang J, Wang J, et al. Mechanisms of ginsenosides exert neuroprotective effects on spinal cord injury: a promising traditional Chinese medicine. Front Neurosci. 2022;16:969056.
- Slater PG, Dominguez-Romero ME, Villarreal M, et al. Mitochondrial function in spinal cord injury and regeneration. Cell Mol Life Sci. 2022 Apr 13;79(5):239.
- Liu JM, Yi Z, Liu SZ, et al. The mitochondrial division inhibitor mdivi-1 attenuates spinal cord ischemia-reperfusion injury both in vitro and in vivo: involvement of BK channels. Brain Res. 2015 Sep 4;1619:155–165.
- Li G, Cao Y, Shen F, et al. Mdivi-1 inhibits astrocyte activation and astroglial scar formation and enhances axonal regeneration after spinal cord injury in rats. Front Cell Neurosci. 2016;10:241.
- Han Q, Xie Y, Ordaz JD, et al. Restoring cellular energetics promotes axonal regeneration and functional recovery after spinal cord injury. Cell Metab. 2020 Mar 3;31(3):623–641 e8.
- Huang N, Li S, Xie Y, et al. Reprogramming an energetic AKT-PAK5 axis boosts axon energy supply and facilitates neuron survival and regeneration after injury and ischemia. Curr Biol. 2021 Jul 26;31(14):3098–3114 e7.
- Liu NK, Deng LX, Wang M, et al. Restoring mitochondrial cardiolipin homeostasis reduces cell death and promotes recovery after spinal cord injury. Cell Death Dis. 2022 Dec 20;13(12):1058.
- Gollihue JL, Patel SP, Eldahan KC, et al. Effects of mitochondrial transplantation on bioenergetics, cellular incorporation, and functional recovery after spinal cord injury. J Neurotrauma. 2018 Aug 1;35(15):1800–1818.
- Karalija A, Novikova LN, Kingham PJ, et al. The effects of N-acetyl-cysteine and acetyl-L-carnitine on neural survival, neuroinflammation and regeneration following spinal cord injury. Neuroscience. 2014 6;Jun(269):143–151.
- Patel SP, Sullivan PG, Lyttle TS, et al. Acetyl-L-carnitine treatment following spinal cord injury improves mitochondrial function correlated with remarkable tissue sparing and functional recovery. Neuroscience. 2012 May;17(210):296–307.
- Zeng H, Lu Y, Huang MJ, et al. Ketogenic diet-mediated steroid metabolism reprogramming improves the immune microenvironment and myelin growth in spinal cord injury rats according to gene and co-expression network analyses. Aging (Albany NY). 2021 May 6;13(9):12973–12995.
- Sayadi JJ, Sayadi L, Satteson E, et al. Nerve injury and repair in a ketogenic milieu: a systematic review of traumatic injuries to the spinal cord and peripheral nervous tissue. PLoS One. 2021;16(1):e0244244.
- Keene DL. A systematic review of the use of the ketogenic diet in childhood epilepsy. Pediatr Neurol. 2006 Jul;35(1):1–5.
- Gasior M, Rogawski MA, Hartman AL. Neuroprotective and disease-modifying effects of the ketogenic diet. Behav Pharmacol. 2006 Sep;17(5–6):431–439.
- Tan BT, Jiang H, Moulson AJ, et al. Neuroprotective effects of a ketogenic diet in combination with exogenous ketone salts following acute spinal cord injury. Neural Regen Res. 2020 Oct;15(10):1912–1919.
- Yarar-Fisher C, Kulkarni A, Li J, et al. Evaluation of a ketogenic diet for improvement of neurological recovery in individuals with acute spinal cord injury: a pilot, randomized safety and feasibility trial. Spinal Cord Ser Cases. 2018;4:88.
- Galluzzi L, Bravo-San Pedro JM, Blomgren K, et al. Autophagy in acute brain injury. Nat Rev Neurosci. 2016 Aug;17(8):467–484.
- Liu S, Sarkar C, Dinizo M, et al. Disrupted autophagy after spinal cord injury is associated with ER stress and neuronal cell death. Cell Death Dis. 2015 Jan;6:e1582.
- Tang P, Hou H, Zhang L, et al. Autophagy reduces neuronal damage and promotes locomotor recovery via inhibition of apoptosis after spinal cord injury in rats. Mol Neurobiol. 2014 Feb;49(1):276–287.
- Sakai K, Fukuda T, Iwadate K. Immunohistochemical analysis of the ubiquitin proteasome system and autophagy lysosome system induced after traumatic intracranial injury: association with time between the injury and death. Am J Forensic Med Pathol. 2014 Mar;35(1):38–44.
- Zhou K, Sansur CA, Xu H, et al. The temporal pattern, flux, and function of autophagy in spinal cord injury. Int J Mol Sci. 2017 Feb;18(2):466.
- Liao HY, Wang ZQ, Ran R, et al. Biological functions and therapeutic potential of autophagy in spinal cord injury. Front Cell Dev Biol. 2021;9:761273.
- Li W, Yao S, Li H, et al. Curcumin promotes functional recovery and inhibits neuronal apoptosis after spinal cord injury through the modulation of autophagy. J Spinal Cord Med. 2021 Jan;44(1):37–45.
- Gu G, Ren J, Zhu B, et al. Multiple mechanisms of curcumin targeting spinal cord injury. Biomed Pharmacother. 2023 Mar;159:114224.
- Luo C, Tao L. The function and mechanisms of autophagy in spinal cord injury. Adv Exp Med Biol. 2020;1207:649–654.
- Zhao H, Chen S, Gao K, et al. Resveratrol protects against spinal cord injury by activating autophagy and inhibiting apoptosis mediated by the SIRT1/AMPK signaling pathway. Neuroscience. 2017 Apr 21;348:241–251.
- Zhou K, Zheng Z, Li Y, et al. TFE3, a potential therapeutic target for Spinal Cord Injury via augmenting autophagy flux and alleviating ER stress. Theranostics. 2020;10(20):9280–9302.
- Xu Y, Hu X, Li F, et al. GDF-11 protects the traumatically injured spinal cord by suppressing pyroptosis and necroptosis via TFE3-mediated autophagy augmentation. Oxid Med Cell Longev. 2021;2021:8186877.
- Ren XD, Wan CX, Niu YL. Overexpression of lncRNA TCTN2 protects neurons from apoptosis by enhancing cell autophagy in spinal cord injury. FEBS Open Bio. 2019 Jul;9(7):1223–1231.
- Bisicchia E, Latini L, Cavallucci V, et al. Autophagy inhibition favors survival of rubrospinal neurons after spinal cord hemisection. Mol Neurobiol. 2017 Sep;54(7):4896–4907.
- Hao HH, Wang L, Guo ZJ, et al. Valproic acid reduces autophagy and promotes functional recovery after spinal cord injury in rats. Neurosci Bull. 2013 Aug;29(4):484–492.
- Lin CW, Chen B, Huang KL, et al. Inhibition of autophagy by estradiol promotes locomotor recovery after spinal cord injury in rats. Neurosci Bull. 2016 Apr;32(2):137–144.
- Saraswat Ohri S, Bankston AN, Mullins SA, et al. Blocking autophagy in oligodendrocytes limits functional recovery after spinal cord injury. J Neurosci. 2018 Jun;38(26):5900–5912.
- Dutta D, Khan N, Wu J, et al. Extracellular vesicles as an emerging frontier in spinal cord injury pathobiology and therapy. Trends Neurosci. 2021 Jun;44(6):492–506.
- Dickens AM, Tovar YRLB, Yoo SW, et al. Astrocyte-shed extracellular vesicles regulate the peripheral leukocyte response to inflammatory brain lesions. Sci Signal. 2017 Apr 4;10(473):eaai7696.
- Zhong D, Cao Y, Li CJ, et al. Neural stem cell-derived exosomes facilitate spinal cord functional recovery after injury by promoting angiogenesis. Exp Biol Med (Maywood). 2020 Jan;245(1):54–65.
- Romanelli P, Bieler L, Scharler C, et al. Extracellular vesicles can deliver anti-inflammatory and anti-scarring activities of mesenchymal stromal cells after spinal cord injury. Front Neurol. 2019;10:1225.
- Yang Y, Ye Y, Kong C, et al. MiR-124 enriched exosomes promoted the M2 polarization of microglia and enhanced hippocampus neurogenesis after traumatic brain injury by inhibiting TLR4 pathway. Neurochem Res. 2019 Apr;44(4):811–828.
- Li D, Zhang P, Yao X, et al. Exosomes derived from miR-133b-modified mesenchymal stem cells promote recovery after spinal cord injury. Front Neurosci. 2018;12:845.
- Chen Y, Tian Z, He L, et al. Exosomes derived from miR-26a-modified MSCs promote axonal regeneration via the PTEN/AKT/mTOR pathway following spinal cord injury. Stem Cell Res Ther. 2021 Apr 5;12(1):224.
- Rong Y, Liu W, Lv C, et al. Neural stem cell small extracellular vesicle-based delivery of 14-3-3t reduces apoptosis and neuroinflammation following traumatic spinal cord injury by enhancing autophagy by targeting Beclin-1. Aging (Albany NY). 2019 Sep 28;11(18):7723–7745.
- Jiang D, Gong F, Ge X, et al. Neuron-derived exosomes-transmitted miR-124-3p protect traumatically injured spinal cord by suppressing the activation of neurotoxic microglia and astrocytes. J Nanobiotechnology. 2020 Jul 25;18(1):105.
- Zhou Z, Li C, Bao T, et al. Exosome-Shuttled miR-672-5p from anti-inflammatory microglia repair traumatic spinal cord injury by inhibiting AIM2/ASC/caspase-1 signaling pathway mediated neuronal pyroptosis. J Neurotrauma. 2022 Aug;39(15–16):1057–1074.
- O’Shea TM, Burda JE, Sofroniew MV. Cell biology of spinal cord injury and repair. J Clin Invest. 2017 Sep;127(9):3259–3270.
- Anderson MA, O’Shea TM, Burda JE, et al. Required growth facilitators propel axon regeneration across complete spinal cord injury. Nature. 2018;561(7723):396–400.
- Lewandowski G, Steward O. AAVshRNA-mediated suppression of PTEN in adult rats in combination with salmon fibrin administration enables regenerative growth of corticospinal axons and enhances recovery of voluntary motor function after cervical spinal cord injury. J Neurosci. 2014 Jul 23;34(30):9951–9962.
- Ohtake Y, Park D, Abdul-Muneer PM, et al. The effect of systemic PTEN antagonist peptides on axon growth and functional recovery after spinal cord injury. Biomaterials. 2014 May;35(16):4610–4626.
- Zukor K, Belin S, Wang C, et al. Short hairpin RNA against PTEN enhances regenerative growth of corticospinal tract axons after spinal cord injury. J Neurosci. 2013 Sep 25;33(39):15350–15361.
- Urban MW, Ghosh B, Block CG, et al. Long-distance axon regeneration promotes recovery of diaphragmatic respiratory function after spinal cord injury. eNeuro. 2019 Sep/Oct;6(5):ENEURO.0096–19.2019.
- Cheng L, Sami A, Ghosh B, et al. Respiratory axon regeneration in the chronically injured spinal cord. Neurobiol Dis. 2021 Jul;155:105389.
- Du K, Zheng S, Zhang Q, et al. Pten deletion promotes regrowth of corticospinal tract axons 1 year after spinal cord injury. J Neurosci. 2015 Jul 1;35(26):9754–9763.
- Pan L, Tan B, Tang W, et al. Combining task-based rehabilitative training with PTEN inhibition promotes axon regeneration and upper extremity skilled motor function recovery after cervical spinal cord injury in adult mice. Behav Brain Res. 2021 May;7(405):113197.
- Metcalfe M, Yee KM, Luo J, et al. Harnessing rAAV-retro for gene manipulations in multiple pathways that are interrupted after spinal cord injury. Exp Neurol. 2022 Apr;350:113965.
- Noro T, Shah SH, Yin Y, et al. Elk-1 regulates retinal ganglion cell axon regeneration after injury. Sci Rep. 2022 Oct 19;12(1):17446.
- Li L, Fang F, Feng X, et al. Single-cell transcriptome analysis of regenerating RGCs reveals potent glaucoma neural repair genes. Neuron. 2022 Aug 17;110(16):2646–2663 e6.
- Wang X-W, Li Q, Liu C-M, et al. Lin28 signaling supports mammalian PNS and CNS axon regeneration. Cell Rep. 2018 Sep 4;24(10):2540–2552 e6.
- Zhang Y, Williams PR, Jacobi A, et al. Elevating growth factor responsiveness and axon regeneration by modulating presynaptic inputs. Neuron. 2019 Jul 3;103(1):39–51 e5.
- Hur E-M, Yang IH, Kim D-H, et al. Engineering neuronal growth cones to promote axon regeneration over inhibitory molecules. Proc Natl Acad Sci U S A. 2011 Mar 22;108(12):5057–5062.
- Ribas VT, Vahsen BF, Tatenhorst L, et al. AAV-mediated inhibition of ULK1 promotes axonal regeneration in the central nervous system in vitro and in vivo. Cell Death Dis. 2021 Feb 26;12(2):213.
- Poplawski GHD, Kawaguchi R, Van Niekerk E, et al. Injured adult neurons regress to an embryonic transcriptional growth state. Nature. 2020 May;581(7806):77–82.
- Belin S, Nawabi H, Wang C, et al. Injury-induced decline of intrinsic regenerative ability revealed by quantitative proteomics. Neuron. 2015 May 20;86(4):1000–1014.
- Alvarez Z, Kolberg-Edelbrock AN, Sasselli IR, et al. Bioactive scaffolds with enhanced supramolecular motion promote recovery from spinal cord injury. Science. 2021 Nov 12;374(6569):848–856.
- Griffin JM, Hingorani Jai Prakash S, Bockemuhl T, et al. Rehabilitation enhances epothilone-induced locomotor recovery after spinal cord injury. Brain Commun. 2023;5(1):fcad005.
- Muller F, De Virgiliis F, Kong G, et al. CBP/p300 activation promotes axon growth, sprouting, and synaptic plasticity in chronic experimental spinal cord injury with severe disability. PLoS Biol. 2022 Sep;20(9):e3001310.
- Cheng Y, Yin Y, Zhang A, et al. Transcription factor network analysis identifies REST/NRSF as an intrinsic regulator of CNS regeneration in mice. Nat Commun. 2022 Jul 29;13(1):4418.
- Kauer SD, Fink KL, Li EHF, et al. Inositol polyphosphate-5-phosphatase K (Inpp5k) enhances sprouting of corticospinal tract axons after CNS Trauma. J Neurosci. 2022 Mar 16;42(11):2190–2204.
- Hilton BJ, Husch A, Schaffran B, et al. An active vesicle priming machinery suppresses axon regeneration upon adult CNS injury. Neuron. 2022 Jan 5;110(1):51–69 e7.
- Brochier C, Jones JI, Willis DE, et al. Poly(ADP-ribose) polymerase 1 is a novel target to promote axonal regeneration. Proc Natl Acad Sci U S A. 2015 Dec 8;112(49):15220–15225.
- Byrne AB, McWhirter RD, Sekine Y, et al. Inhibiting poly(ADP-ribosylation) improves axon regeneration. Elife. 2016 Oct 4;5:e12734.
- Wang X, Sekine Y, Byrne AB, et al. Inhibition of poly-ADP-ribosylation fails to increase axonal regeneration or improve functional recovery after adult mammalian CNS injury. eNeuro. 2016 Nov-Dec;3(6):ENEURO.0270–16.2016.
- Ahmed Z, Tuxworth RI. The brain-penetrant ATM inhibitor, AZD1390, promotes axon regeneration and functional recovery in preclinical models of spinal cord injury. Clin Transl Med. 2022 Jul;12(7):e962.
- Ma C, Teng L, Lin G, et al. L-leucine promotes axonal outgrowth and regeneration via mTOR activation. FASEB J. 2021 May;35(5):e21526.
- Saikia JM, Chavez-Martinez CL, Kim ND, et al. A critical role for DLK and LZK in axonal repair in the mammalian spinal cord. J Neurosci. 2022 May 4;42(18):3716–3732.
- Boato F, Guan X, Zhu Y, et al. Activation of MAP2K signaling by genetic engineering or HF-rTMS promotes corticospinal axon sprouting and functional regeneration. Sci Transl Med. 2023 Jan 4;15(677):eabq6885.
- Jacobi A, Tran NM, Yan W, et al. Overlapping transcriptional programs promote survival and axonal regeneration of injured retinal ganglion cells. Neuron. 2022 Aug 17;110(16):2625–2645 e7.
- Petrova V, Pearson CS, Ching J, et al. Protrudin functions from the endoplasmic reticulum to support axon regeneration in the adult CNS. Nat Commun. 2020 Nov 5;11(1):5614.
- Wang X, Yang C, Wang X, et al. Driving axon regeneration by orchestrating neuronal and non-neuronal innate immune responses via the IFNgamma-cGAS-STING axis. Neuron. 2022 Nov 4;111(2):236–255.e7.
- Au NPB, Chand R, Kumar G, et al. A small molecule M1 promotes optic nerve regeneration to restore target-specific neural activity and visual function. Proc Natl Acad Sci U S A. 2022 Nov;119(44):e2121273119.
- Au NPB, Kumar G, Asthana P, et al. Clinically relevant small-molecule promotes nerve repair and visual function recovery. NPJ Regen Med. 2022 Oct 1;7(1):50.
- Ko SH, Apple EC, Liu Z, et al. Age-dependent autophagy induction after injury promotes axon regeneration by limiting NOTCH. Autophagy. 2020 Nov;16(11):2052–2068.
- Vargas EJM, Matamoros AJ, Qiu J, et al. The microtubule regulator ringer functions downstream from the RNA repair/splicing pathway to promote axon regeneration. Genes Dev. 2020 Feb 1;34(3–4):194–208.
- Li F, Sami A, Noristani HN, et al. Glial metabolic rewiring promotes axon regeneration and functional recovery in the central nervous system. Cell Metab. 2020 Sep 9;32(5):767–785.
- Jorstad NL, Wilken MS, Todd L, et al. STAT signaling modifies Ascl1 chromatin binding and limits neural regeneration from Muller glia in adult mouse retina. Cell Rep. 2020 Feb 18;30(7):2195–2208 e5.
- Jorstad NL, Wilken MS, Grimes WN, et al. Stimulation of functional neuronal regeneration from Muller glia in adult mice. Nature. 2017 Aug 3;548(7665):103–107.
- Song BG, Kwon SY, Kyung JW, et al. Synaptic cell adhesion molecule 3 (SynCAM3) deletion promotes recovery from spinal cord injury by limiting glial scar formation. Int J Mol Sci. 2022 Jun 1;23(11):6218.
- Nogueira-Rodrigues J, Leite SC, Pinto-Costa R, et al. Rewired glycosylation activity promotes scarless regeneration and functional recovery in spiny mice after complete spinal cord transection. Dev Cell. 2022 Feb 28;57(4):440–450 e7.
- Elahi A, Emerson J, Rudlong J, et al. Deletion or inhibition of astrocytic transglutaminase 2 promotes functional recovery after spinal cord injury. Cells. 2021 Oct 29;10(11):2942.
- Filiano AJ, Tucholski J, Dolan PJ, et al. Transglutaminase 2 protects against ischemic stroke. Neurobiol Dis. 2010 Sep;39(3):334–343.
- Ge X, Ye W, Zhu Y, et al. USP1/UAF1-stabilized METTL3 promotes reactive astrogliosis and improves functional recovery after spinal cord injury through m(6)A modification of YAP1 mRNA. J Neurosci. 2023 Mar 1;43(9):1456–1474.
- Goldshmit Y, Schokoroy Trangle S, Afergan F, et al. Nucleolin inhibitor GroA triggers reduction in epidermal growth factor receptor activation: pharmacological implication for glial scarring after spinal cord injury. J Neurochem. 2016 Sep;138(6):845–858.
- Li ZW, Li JJ, Wang L, et al. Epidermal growth factor receptor inhibitor ameliorates excessive astrogliosis and improves the regeneration microenvironment and functional recovery in adult rats following spinal cord injury. J Neuroinflammation. 2014 Apr;5(11):71.
- Li Z, Yu S, Liu Y, et al. SU16f inhibits fibrotic scar formation and facilitates axon regeneration and locomotor function recovery after spinal cord injury by blocking the PDGFRbeta pathway. J Neuroinflammation. 2022 Apr 16;19(1):95.
- Dupraz S, Hilton BJ, Husch A, et al. RhoA controls axon extension independent of specification in the developing brain. Curr Biol. 2019 Nov 18;29(22):3874–3886 e9.
- Stern S, Hilton BJ, Burnside ER, et al. RhoA drives actin compaction to restrict axon regeneration and astrocyte reactivity after CNS injury. Neuron. 2021 Nov 3;109(21):3436–3455 e9.
- Fournier AE, Takizawa BT, Strittmatter SM. Rho kinase inhibition enhances axonal regeneration in the injured CNS. J Neurosci. 2003 Feb 15;23(4):1416–1423.
- Li Y, He X, Kawaguchi R, et al. Microglia-organized scar-free spinal cord repair in neonatal mice. Nature. 2020 Nov;587(7835):613–618.
- Zhang S, Fujita Y, Matsuzaki R, et al. Class I histone deacetylase (HDAC) inhibitor CI-994 promotes functional recovery following spinal cord injury. Cell Death Dis. 2018;9(5):460.
- Kuboyama T, Wahane S, Huang Y, et al. HDAC3 inhibition ameliorates spinal cord injury by immunomodulation. Sci Rep. 2017;7(1):8641.
- Zheng Z, Zhou Y, Ye L, et al. Histone deacetylase 6 inhibition restores autophagic flux to promote functional recovery after spinal cord injury. Exp Neurol. 2020 Feb;324:113138.
- Lu Y, Brommer B, Tian X, et al. Reprogramming to recover youthful epigenetic information and restore vision. Nature. 2020 Dec;588(7836):124–129.
- Hong JY, Davaa G, Yoo H, et al. Ascorbic acid promotes functional restoration after spinal cord injury partly by epigenetic modulation. Cells. 2020 May 25;9(5):1310.
- Moyon S, Frawley R, Marechal D, et al. TET1-mediated DNA hydroxymethylation regulates adult remyelination in mice. Nat Commun. 2021 Jun 7;12(1):3359.
- Patel NJ, Hogan KJ, Rizk E, et al. Ancestral folate promotes neuronal regeneration in serial generations of progeny. Mol Neurobiol. 2020 Apr;57(4):2048–2071.
- Weng YL, Wang X, An R, et al. Epitranscriptomic m(6)A regulation of axon regeneration in the adult mammalian nervous system. Neuron. 2018 Jan 17;97(2):313–325 e6.
- Strickland ER, Hook MA, Balaraman S, et al. MicroRNA dysregulation following spinal cord contusion: implications for neural plasticity and repair. Neuroscience. 2011 Jul;14(186):146–160.
- Wang B, Shen PF, Qu YX, et al. miR-940 promotes spinal cord injury recovery by inhibiting TLR4/NF-kappaB pathway-mediated inflammation. Eur Rev Med Pharmacol Sci. 2019 Apr;23(8):3190–3197.
- Ghibaudi M, Boido M, Green D, et al. miR-7b-3p exerts a dual role after spinal cord injury, by supporting plasticity and neuroprotection at cortical level. Front Mol Biosci. 2021;8:618869.
- Gu S, Xie R, Liu X, et al. Long coding RNA XIST contributes to neuronal apoptosis through the downregulation of AKT phosphorylation and is negatively regulated by miR-494 in rat spinal cord injury. Int J Mol Sci. 2017 Apr 1;18(4):732.
- Zhou HJ, Wang LQ, Wang DB, et al. Long noncoding RNA MALAT1 contributes to inflammatory response of microglia following spinal cord injury via the modulation of a miR-199b/IKKbeta/NF-kappaB signaling pathway. Am J Physiol Cell Physiol. 2018 Jul 1;315(1):C52–C61.
- Cao Y, Jiang C, Lin H, et al. Silencing of long noncoding RNA growth arrest-specific 5 alleviates neuronal cell apoptosis and inflammatory responses through sponging microRNA-93 to repress PTEN expression in spinal cord injury. Front Cell Neurosci. 2021;15:646788.
- Chen Z, Li Z, Jiang C, et al. MiR-92b-3p promotes neurite growth and functional recovery via the PTEN/AKT pathway in acute spinal cord injury. J Cell Physiol. 2019 Dec;234(12):23043–23052.
- Zhang N, Lin J, Lin VPH, et al. A 3D fiber-hydrogel based non-viral gene delivery platform reveals that microRNAs promote axon regeneration and enhance functional recovery following spinal cord injury. Adv Sci (Weinh). 2021 8;Aug(15):e2100805.
- Zhang L, Wang Z, Li B, et al. The inhibition of miR-17-5p promotes cortical neuron neurite growth via STAT3/GAP-43 pathway. Mol Biol Rep. 2020 Mar;47(3):1795–1802.
- Kar AN, Lee SJ, Sahoo PK, et al. MicroRNAs 21 and 199a-3p regulate axon growth potential through modulation of PTEN and mTor mRNAs. eNeuro. 2021 Jul-Aug;8(4):ENEURO.0155–21.2021.
- Qian X, Lin G, Wang J, et al. CircRNA_01477 influences axonal growth via regulating miR-3075/FosB/Stat3 axis. Exp Neurol. 2022 Jan;347:113905.
- Bhalala OG, Pan L, Sahni V, et al. microRNA-21 regulates astrocytic response following spinal cord injury. J Neurosci. 2012 Dec 12;32(50):17935–17947.
- Su Y, Chen Z, Du H, et al. Silencing miR-21 induces polarization of astrocytes to the A2 phenotype and improves the formation of synapses by targeting glypican 6 via the signal transducer and activator of transcription-3 pathway after acute ischemic spinal cord injury. FASEB J. 2019 Oct;33(10):10859–10871.
- Wei H, Wu X, You Y, et al. Systematic analysis of purified astrocytes after SCI unveils Zeb2os function during astrogliosis. Cell Rep. 2021 Feb 2;34(5):108721.
- Han Q, Ordaz JD, Liu NK, et al. Descending motor circuitry required for NT-3 mediated locomotor recovery after spinal cord injury in mice. Nat Commun. 2019 Dec 20;10(1):5815.
- Wang Y, Wu W, Wu X, et al. Remodeling of lumbar motor circuitry remote to a thoracic spinal cord injury promotes locomotor recovery. Elife. 2018;9:7.
- Gao Z, Yang Y, Feng Z, et al. Chemogenetic stimulation of proprioceptors remodels lumbar interneuron excitability and promotes motor recovery after SCI. Mol ther. 2021 Aug 4;29(8):2483–2498.
- Shepard CT, Pocratsky AM, Brown BL, et al. Silencing long ascending propriospinal neurons after spinal cord injury improves hindlimb stepping in the adult rat. Elife. 2021 Dec 2;10:e70058.
- Nakanishi T, Fujita Y, Yamashita T. Neuropilin-1-mediated pruning of corticospinal tract fibers is required for motor recovery after spinal cord injury. Cell Death Dis. 2019 Jan;10(2):67.
- Suzuki K, Elegheert J, Song I, et al. A synthetic synaptic organizer protein restores glutamatergic neuronal circuits. Science. 2020 Aug 28;369:6507.
- Kikuchi T, Tohda C, Suyama M. Recovery of motor function of chronic spinal cord injury by extracellular pyruvate kinase isoform M2 and the underlying mechanism. Sci Rep. 2020 Nov 10;10(1):19475.
- Fu Q, Hue J, Li S. Nonsteroidal anti-inflammatory drugs promote axon regeneration via RhoA inhibition. J Neurosci. 2007 Apr 11;27(15):4154–4164.
- Sun W, Larson MJ, Kiyoshi CM, et al. Gabapentinoid treatment promotes corticospinal plasticity and regeneration following murine spinal cord injury. J Clin Invest. 2020 Jan 2;130(1):345–358.
- Dietz VA, Roberts N, Knox K, et al. Fighting for recovery on multiple fronts: the past, present, and future of clinical trials for spinal cord injury. Front Cell Neurosci. 2022;16:977679.