Abstract
Aims
To study myocardial deformation in fetuses with ventricular afterload increase compared with gestational age-matched controls using speckle tracking echocardiography.
Methods and results
Eighty-nine fetuses were retrospectively selected from the pregnancy screen by echocardiography. There are 41 fetuses with gestational age-matched normal heart served as the control group, 25 fetuses with congenital heart disease (CHD) leading to left ventricular (LV) afterload increase as group LVA and 23 fetuses with CHD leading to right ventricular(RV) afterload increases as group RVA. LV and RV fractional shortening (FS) were measured by conventional methods. The longitudinal strain (LS) and strain rate (LSr) were analyzed by EchoPac software. Group LVA and RVA compared with control group, the LV FS was no significant difference, but LS and LSr values of LV were lower in fetuses with LVA compared to the control group (LS:−15.97(−12.50,−22.52)vs −27.53(−24.33,−29.16) %, p < .01; systolic strain rate (SRs):-1.34(−1.12,−2.16) vs −2.55(−2.28,−2.92) 1/sec, p < .01; early diastolic strain rate (SRe):1.70 ± 0.57 vs 2.46 ± 0.61 1/sec, p < 0.01; late diastolic strain rate (SRa):1.62 ± 0.82 vs 2.39 ± 0.81 1/sec, p < .01). LS and LSr values of LV or RV were lower in fetuses with RVA compared to the control group (LV: LS:−21.52 ± 6.68 vs −26.79 ± 3.22%, p < .01; SRs:-2.11 ± 0.78 vs −2.56 ± 0.43 1/sec; p = .02; RV: LS:−17.64 ± 7.58 vs −26.38 ± 3.97%, p < .01; SRs:−1.62 ± 0.67 vs −2.37 ± 0.44 1/sec; p < .01).
Conclusion
The results of this study showed that the ventricular LS, LSr, SRs, SRe, SRa values were lower in fetuses with LV or RV afterload increasing CHD estimated by speckle tracking imaging but LV and RV FS were normal,which indicated the strain imaging is feasible in evaluating cardiac function of fetus, and may be more sensitive.
Introduction
Fetal heart development can be affected by a variety of factors leading to primary or secondary myocardial systolic and/or diastolic dysfunction, reducing cardiac output [Citation1]. As the pregnancy progresses and the fetus develops, the fetal cardiac function is also in a dynamic process. Fetal echocardiography has become the main instrument for prenatal diagnosis of fetal cardiac structure and function abnormalities [Citation2]. In the past, fetal cardiac function was assessed by the ejection fraction (EF) and ventricular fraction shortening (FS) using M-mode and two-dimensional ultrasound. However, the use of those techniques before birth had some limitations because of the small dimensions of the fetal heart, movement artifacts, inappropriate fetal position, and the geometric complexity of the fetal right ventricle (RV) [Citation3]. Due to the greater technical difficulty in the use of conventional markers of cardiac function, there has been increasing interest in the study of advanced methods.Two-dimensional speckle tracking imaging is an accurate, convenient and reproducible method for cardiac function assessment; which has been widely used to assess adult cardiac function. However, its assessment of fetal cardiac function is still in development [Citation4,Citation5].
Congenital heart disease (CHD) of left ventricular (LV) or RV afterload increase is common in the fetus. Changes in ventricular myocardial function have been observed in children and adults with CHD of ventricular afterload, even when repaired without residual gradients and in the absence of hypertension. Even children who underwent repair at a very young age have been found to have ventricular function impairment [Citation6]. Studies on myocardial functions in fetal ventricular afterload increase are seldomly reported. We hypothesized that fetuses with ventricular afterload increase may present impaired myocardial function. Hence, the aim of this study was to study myocardial deformation in fetuses with ventricular afterload increase compared with gestational age-matched controls using speckle tracking echocardiography.
Method
Study population: In this retrospective study, the fetuses have simple CHD lead to LV or RV afterload increasing were selected as study population, among the pregnancies had echocardiograms between July 2015 to December 2018 in our center. Among these pregnancies, 20 fetuses were excluded due to pregnancy with chronic diseases such as diabetes and hypertension, and co-infection, or fetuses combined with complexes’ cardiac malformation, and 11 fetuses were excluded because of poor image quality. Final study population including 25 fetuses with simple CHD lead to LV afterload increasing as group LVA and 23 fetuses with RV CHD lead to afterload increasing as group RVA. Prenatal diagnosis was performed by more than 2 physicians. Surgical or autopsy findings differed from the prenatal diagnosis cases were excluded.
Any congenital stenotic lesions in the left ventricular outflow tract, including the subvalvular, valvular, or supravalvular portions of the aortic valve complex, can cause an increase in left ventricular afterload [Citation7]. Similarly, stenotic lesions in the right ventricular outflow tract, including the mid-RV, infundibulum, pulmonary valve, supravalvular region, or branch, can also lead to an increase in left ventricular afterload [Citation8]. We also enrolled 41 fetuses with normal hearts and matched them with healthy pregnancies in terms of gestational age to serve as the control group. The fetuses in the control group were closely monitored using echocardiography after birth to confirm the absence of any cardiac abnormalities. This study was approved by the Ethics Committee of Fuwai Yunnan cardiovascular hospital.
Echocardiographic and imaging analysis
Imaging was performed with Vivid E95 (GE, Milwaukee, WI, USA). A complete echocardiographic study was performed using standard views with care taken to avoid foreshortening. All images were recorded for 3 to 5 cardiac cycles. Measurements were performed using computerized off-line analysis software (EchoPac 201, GE).
Traditional Measurements: LV and RV FS (calculated by M-mode, take the transverse four-chamber or bi-ventricle short-axis view, the horizontal sampling line of the papillary muscle is perpendicular to the anterior wall of the RV, the interventricular septum, and the posterior wall of the LV).
Mitral inflow velocities were obtained from the apical window using pulsed wave Doppler with the sample volume placed at the center between tips of the mitral valve leaflets.
Tissue Doppler measurement: systolic s' of the mitral annulus and tricuspid annulus, early diastolic e' and late diastolic a'. The isovolumetric contraction time (IVCT), isovolumic relaxation time (IVRT) and ejection time (ET) was measured from tissue Doppler curve of the mitral or tricuspid annulus. The LV and RV myocardium performance index (MPI) was calculated as (IVCT + IVRT)/ET ).
Figure 1. The tissue Doppler curve showed the calculation of the MPI index. MPI: myocardial performance index; ICT: isovolumetric contraction time; IRT: isovolumic relaxation time; ET: ejection time; LV: left ventricle; LA: left atria; RV, right ventricle; RA, right atria; DAO: descending aorta.
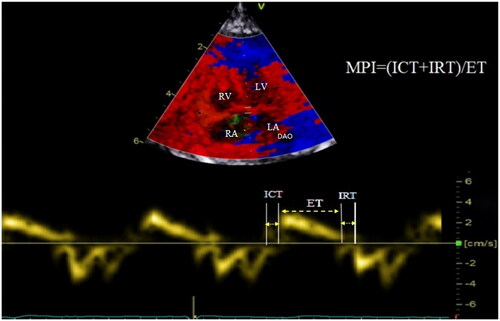
Two-dimensional speckle tracking parameters: a fetal four-chamber view with a frame rate of at least 80 frames per second is selected. The cardiac cycle was defined by the examiner selecting two consecutive end-diastolic frames (mitral valve closure) so that a single heart cycle was analyzed. LV and RV endocardial borders were defined manually at end diastole and tracking curves of endocardial and epicardial borders were created automatically by the software in subsequent frames. The caution is made for the fetal endocardium boarder as clear and magnified as much as possible. The same technique was used for both ventricles. The LV and RV longitudinal strain(LS), systolic strain rate(SRs), and early diastolic strain rate(SRe), late diastolic strain rate (SRa) are presented as .
Statistical analysis
Statistical analysis was performed using SPSS 24.0 statistical software. Normally distributed continuous variables are presented as the mean ± standard deviation (SD), and variables that did not follow a normal distribution are presented as medians. Graphically assess the distribution of continuous variables with histograms and quantile-quantile plots. Independent t-tests were used to analyze normally distributed continuous data, and Wilcoxon rank sum tests were used to analyze skewed data. p < .05 was considered as statistically significant. Credibility was selected by a one-way random effect model and inter-observer credibility was performed in 69 (control group 41, case group 28) using a two-factor random effects model to evaluate intra-group correlation coefficient. Correlation coefficient >0.8 is considered to be highly reliable, and 0.6 < correlation coefficient is considered to be good.
Results
Clinical characteristics
Twenty-five fetuses with different CHD leading to the LV afterload increasing as group LVA, and 23 fetuses with different CHD leading to the RV afterload increasing as group RVA. The disease groups are shown in . 25 fetuses of LVA, 23 fetuses of RVA and 41 gestational aged-matched controls were studied. There were no significant differences in the gestational age between the LVA, RVA and controls (LVA vs controls:25.52 ± 3.08 vs 25.96 ± 2.92 weeks, p > .05; RVA vs controls: 26.13 ± 3.71 vs 25.96 ± 2.92 weeks, p > .05).
Table 1. Diagnosis of congenital heart disease with left or right outflow obstruction.
Group LVA compared with the control group
LS value of LV was lower in fetuses with LVA compared to the control group (−15.97(−12.50,−22.52) vs −27.53(−24.33,−29.16)%, p < .01), however there were no statistical differences in FS of LV. Global systolic and diastolic longitudinal strain rate (LSr) values of LV were lower in fetuses with LVA (systolic: SRs:−1.34(−1.12,−2.16) vs −2.55(−2.28,−2.92) 1/sec, p < .01, diastolic: SRe:1.70 ± 0.57 vs 2.46 ± 0.61 1/sec, p < .01; SRa:1.62 ± 0.82 vs 2.39 ± 0.81 1/sec, p < .01). There was no significant difference in systolic and diastolic LS, and LSr values of RV between the RVA and the control group. The comparison of parameters between LVA and control group were listed in .
Table 2. The comparison of parameters between LVA and control group.
Group RVA compared with the control group
LS and LSr values of LV and RV were lower in fetuses with RVA compared to the control group (LV:LS: −21.52 ± 6.68 vs −26.79 ± 3.22%, p < .01; SRs:-2.11 ± 0.78 vs −2.56 ± 0.43 1/sec; p = 0.02; RV: LS:-17.64 ± 7.58 vs −26.38 ± 3.97%, p < .01; SRs:-1.62 ± 0.67 vs −2.37 ± 0.44 1/sec; p < .01), but there were no statistical differences in FS of LV and RV. The comparison of parameters between RVA and control group were listed in .
Table 3. The comparison of parameters between RVA and control group.
The correlation coefficients of intra- and inter-observer were good (Inter-class correlation coefficient > 0.6, ).
Table 4. Inter-observer and intra-observer variability.
Discussion
The results of our study demonstrated that the LV and RV values of LS, LSr, SRs, SRe, SRa were reduced in fetuses with LV and RV afterload increases estimated by speckle tracking imaging but their LV and RV FS were normal, which indicated the strain imaging is feasible in evaluating cardiac dysfunction of a fetus in early stage, and may be more sensitive. Although it has already been suggested that speckle tracking may have the potential to detect abnormalities of fetal cardiac function earlier than other conventional echocardiographic methods [Citation9], the clinical relevance of this technique must be demonstrated in each specific fetal condition. The role of this tool in CHD is currently under investigation and based on our results it seems promising.
The development of fetal heart structure and function is a dynamic process. Intrinsic factors leading to fetal cardiac dysfunction include cardiomyopathy, structural heart disease, etc. Fetal CHD often leads to changes in cardiac hemodynamics, volume and resistance load [Citation10–13], so it has a direct impact on the fetal cardiac function. Echocardiography is the most important technique for detecting fetal CHD, monitoring disease progression, and estimating changes in cardiac function and prognosis. For CHD, the deterioration of cardiac function is one of the causes of intrauterine death, but heart failure will experience a remodeling process in the early stage, so the timely detection of fetal cardiac insufficiency has clinical significance for predicting prognosis [Citation14]. Our results and previous study [Citation15,Citation16] indicated that with the development of new technologies, subclinical cardiac dysfunction in the fetal period can be detected.
Effects of increased LV afterload on cardiac function in fetuses with CHD
Studies have shown that LV and RV dysfunction can be observed in children and adults with LV outflow obstruction such as aortic coarctation, aortic arch disruption and other malformations, in the early stage [Citation17]. Previous studies have shown that the current possible mechanisms of increasing ventricular afterload on cardiac function may include three aspects: First, stenotic aortic arch will result in LV resistance increases, leads to myocardial cell hyperplasia, cardiac hypertrophy and myocardial fibrosis [Citation18]; the ventricular septum subendocardial and epicardial myocardial fibrosis from the autopsy results of congenital aortic arch coarctation has been reported [Citation19], which leading to systolic and diastolic dysfunction. Second, the aortic coarctation result in an arterial reflex wave increases LV diastolic pressure, while increasing aortic diastolic pressure and aortic wall stiffness. Third, aortic arch coarctation shortens diastolic time, corresponding coronary perfusion time is shortened, endomyocardial perfusion is reduced, and resulting in myocardial contractile function impaired [Citation20], present as the subclinical manifestation to heart failure. Some scholars have confirmed that the LV FS is still normal estimated by routine 2D echocardiography, but the deformation parameters have been significantly reduced in both systolic and diastolic phases in patients with LV outflow tract obstruction. In addition, the results of our study are consistent with Miranda JO et al. [Citation17] findings, they studied 12 fetuses with aortic coarctation have lower value of LV systolic LS, systolic and diastolic LSr when compared to gestational age-matched controls.
Some studies have pointed out that in LVA, the RV strain is also lower than normal [Citation21]. The study indicated that the RV strain was lower than control in all fetuses with aortic coarctation, which due to the function of interventricular septum can be affected by the LV and RV contractile force, so there is a significant correlation between the deformation parameters of the left and right ventricles [Citation21]. In addition to the hemodynamic, preload and afterload changes, a large part depends on the functional state of the interventricular septum, which is affected by factors such as LV shape, pressure, and pressure gradient between the left and right ventricles. Therefore, ventricular septum function can be impaired by increased LV afterload which in turn causes changes in RV function in patients with congenital aortic coarctation, interrupted aortic arch, etc [Citation22]. In our study, the value of RV LS and LSr were slightly lower in LVA than the control group.
The effect of RV afterload increasing on cardiac function in fetus with CHD
In this study, the RVA group consisted mainly of fetuses with dysplasia of pulmonary artery, pulmonary stenosis, and pulmonary atresia, our results showed normal LV and RV FS compared with the control group, but The LS and LSr values of the LV and RV were significantly lower than those of the control group, suggesting that the systolic and diastolic functions of the LV and RV were impaired.
During fetal life, cardiomyocytes grow with cellular proliferation rather than cellular hypertrophy. After birth, cell hypertrophy is predominant [Citation23,Citation24], and LV and RV myocytes are arranged differently. In the fetal period, RV-free wall myocardial cells are longitudinal and oblique fibers. The main manifestation is longitudinal shortening. The LV free wall cardiomyocytes are dominated by circumference fibers, so the main manifestation is circumference shortening [Citation25], so the right heart system dominates during the fetal period. In an animal study, the authors pointed out that after RV afterload increasing, the length of the end-diastolic muscle fibers increased, but the shortening of the muscle fibers during systole did not change [Citation26], so the right heart pressure load increased, the RV myocardial elasticity decreased resulting in a decrease of RV myocardial deformability.
The study indicated that an increase in RV afterload causes the value of RV strain decrease, and the strain value is more predictive and stable than the RV end-diastolic period in differentiating the acute from chronic RV pressure overload [Citation27]. The study suggests that the strain and strain rate between the LV and RV-free walls may be related to the direction of muscle fibers, myocardial force and ventricular geometry. In addition, the conduction of action potential between cardiomyocytes is also considered to cause local strain unequal strain rates [Citation28], however, the function of interventricular septum is affected by left and right cardiac functions. The study by DeVore et al. [Citation29] showed that in fetuses with structural abnormalities of the RV, in addition to abnormal RV strain, some fetuses also had reduced value of LV LS. In our study, we also found that the value of LV systolic and diastole deformation was decreased in fetuses with the RV afterload increased compared with the control group.
The MPI index is an ultrasound index that quantitatively evaluates the overall cardiac function. Studies have shown that there is good agreement between the MPI index and the “gold standard” radionuclide angiography in evaluating adult cardiac function [Citation30]. Compared with conventional cardiac function parameters, the MPI index is more accurate, and sensitive, it is easy to operate and not affected by ventricular shape and heart rate, which is helpful for early diagnosis of fetal cardiac dysfunction. It has been increasingly used in clinical practice [Citation31,Citation32]. Studies have shown that the normal fetal MPI index value is relatively stable, an average value was 0.36, which may vary slightly throughout the pregnancy, the range is about 0.28–0.44 [Citation33]. The numerical value is related to the severity and prognosis of fetal cardiac dysfunction; when the MPI index value is ≥0.54, the sensitivity is 87%, the specificity is 75%, and the likelihood ratio is 3.47, in predicting adverse pregnancy outcomes. When the MPI index value is ≥0.67, the sensitivity is 100%, the specificity is 81%, and the likelihood ratio is 5.28 in predicting perinatal mortality [Citation34,Citation35]. Although the MPI index evaluates cardiac function more accurately and sensitively, it is worth to note that in patients with aortic regurgitation or pulmonary regurgitation, ventricular ejection time is prolonged, and the accuracy of assessment of cardiac function using the MPI index may be reduced [Citation36]; there are certain restrictions. In this study, the MPI index increased in each subgroup of the fetuses compared with the normal control group.
Limitations
This is a retrospective study with a number of important limitations. First, fetal electrocardiograms (ECGs) cannot be easily recorded during intrauterine life. In the absence of a fetal ECG, manual determination of cardiac cycles can be error-prone. Secondly, since fetal growth and development in utero is a dynamic process, the impact of CHD on fetal heart function is also dynamic, and continuous monitoring of cardiac function throughout the fetal period can reflect changes in fetal heart function. But this study only estimated fetal heart function at a certain gestational age. Third, the requirement for advanced ultrasound tools limits the application of this new technology in routine clinical practice. In particular, our study only included patients with uncomplex congenital abnormalities that resulted in an increase in left or right ventricular afterload, and those who had good image quality. Cases selection bias is a major limitation of this study.
Conclusion
The results of the present study showed that fetuses with increased LV or RV afterload had normal LV and RV volume fractions but reduced values of LV or RV LS, LSr, SRs, SRe, SRa, suggesting speckle tracking imaging can be used to assess myocardial function in fetal heart, and this technique is feasible and reproducible in clinical practice. Quantitative assessment of LV myocardium deformation changes with cardiac structural abnormalities can provide further information for clinical guidance during pregnancy. However, large-scale cohort studies are still needed to further confirm the potential clinical value of fetal myocardial mechanical analysis and standardize its clinical application.
Disclosure statement
No potential conflict of interest was reported by the author(s).
Additional information
Funding
References
- Huhta JC. Fetal congestive heart failure. Semin Fetal Neonatal Med. 2005;10(6):542–552.
- Pham A, Melchior M. Screening for fetal congenital heart disease. CMAJ. 2017;189(12):E468–E468.
- Pauliks LB. The effect of pregestational diabetes on fetal heart function. Expert Rev Cardiovasc Ther. 2015;13(1):67–74.
- Comas M, Crispi F. Assessment of fetal cardiac function using tissue doppler techniques. Fetal Diagn Ther. 2012;32(1–2):30–38.
- DeVore GR, Polanco B, Satou G, et al. Two-dimensional speckle tracking of the fetal heart: a practical step-by-step approach for the fetal sonologist. J Ultrasound Med. 2016;35(8):1765–1781.
- Van der Ende J, Vázquez Antona CA, Erdmenger Orellana J, et al. Left ventricular longitudinal strain measured by speckle tracking as a predictor of the decrease in left ventricular deformation in children with congenital stenosis of the aorta or coarctation of the aorta. Ultrasound Med Biol. 2013;39(7):1207–1214.
- Gaynor JW, Elliott MJ. Congenital left ventricular outflow tract obstruction. J Heart Valve Dis. 1993;2(1):80–93.
- Bashore TM. Adult congenital heart disease: right ventricular outflow tract lesions. Circulation. 2007;115(14):1933–1947.
- Van Mieghem T, DeKoninck P, Steenhaut P, et al. Methods for prenatal assessment of fetal cardiac function. Prenat Diagn. 2009;29(13):1193–1203.
- Gardiner HM. Response of the fetal heart to changes in load: from hyperplasia to heart failure. Heart. 2005;91(7):871–873.
- Danford DA, Cronican PH. Hypoplastic left heart syndrome: progression of left ventricular dilation and dysfunction to left ventricular hypoplasia in utero. Am Heart J. 1992;123(6):1712–1713.
- Samson F, Bonnet N, Heimburger M, et al. Left ventricular alterations in a model of fetal left ventricular overload. Pediatr Res. 2000;48(1):43–49.
- Miller CE, Wong CL, Sedmera D. Pressure overload alters stress-strain properties of the developing chick hear. Am J Physio Heart Circ Physio. 2003;285:1849–1856.
- Crispi F, Gratacós E. Fetal cardiac function: technical considerations and potential research and clinical applications. Fetal Diagn Ther. 2012;32(1–2):47–64.
- Crispi F, Hernandez-Andrade E, Pelsers MM, et al. Cardiac dysfunction and cell damage across clinical stages of severity in growth-restricted fetuses. Am J Obstet Gynecol. 2008;199(3):254.e1–254.e8.
- Van Mieghem T, Gucciardo L, Done E, et al. Left ventricular cardiac function in fetuses with congenital diaphragmatic hernia and the effect of fetal endoscopic tracheal occlusion. Ultrasound Obstet Gynecol. 2009;34(4):424–429.
- Miranda JO, Hunter L, Tibby S, et al. Myocardial deformation in fetuses with coarctation of the aorta: a case-control study. Ultrasound Obstet Gynecol. 2017;49(5):623–629.
- Jashari H, Rydberg A, Ibrahimi P, et al. Left ventricular response to pressure afterload in children: aortic stenosis and coarctation: a systematic review of the current evidence. Int J Cardiol. 2015;178:203–209.
- Cheitlin MD, Robinowitz M, McAllister H, et al. The distribution of fibrosis in the left ventricle in congenital aortic stenosis and coarctation of the aorta. Circulation. 1980;62(4):823–830.
- Chambers J. The left ventricle in aortic stenosis: evidence for the use of ACE inhibitors. Heart. 2006;92(3):420–423.
- Germanakis I, Matsui H, Gardiner HM. Myocardial strain abnormalities in fetal congenital heart disease assessed by speckle tracking echocardiography. Fetal Diagn Ther. 2012;32(1-2):123–130.
- Giusca S, Jurcut R, Ginghina C, et al. The right ventricle: anatomy, physiology and functional assessment. Acta Cardiol. 2010;65(1):67–77.
- Erickson CT, Levy PT, Craft M, et al. Maturational patterns in right ventricular strain mechanics from the fetus to the young infant. Early Hum Dev. 2019;129:23–32.
- Ahuja P, Sdek P, MacLellan WR. Cardiac myocyte cell cycle control in development, disease, and regeneration. Physiol Rev. 2007;87(2):521–544.
- Haddad F, Hunt SA, Rosenthal DN, et al. Right ventricular function in cardiovascular disease, part I: anatomy, physiology, aging, and functional assessment of the right ventricle. Circulation. 2008;117(11):1436–1448.
- Wheeler M, Leipsic J, Trinh P, et al. Right ventricular assessment in adult congenital heart disease patients with right ventricle-to pulmonary artery. J Am Soc Echocardiogr. 2015;28(5):522–532.
- Rudolph AM. Myocardial growth before and after birth: clinical implications. Acta Paediatr. 2000;89:129–133.
- Hayabuchi Y, Sakata M, Kagami S. Right ventricular myocardial deformation patterns in children with congenital heart disease associated with right ventricular pressure overload. Eur Heart J Cardiovasc Imaging. 2015;16(8):890–899.
- DeVore GR, Klas B, Satou G, et al. Longitudinal annular systolic displacement compared to global strain in normal fetal hearts and those with cardiac abnormalities. J Ultrasound Med. 2018;37(5):1159–1171.
- Amano H, Abe S, Hirose S, et al. Comparison of echocardiographic parameters to assess right ventricular function in pulmonary hypertension. Heart Vessels. 2017;32(10):1214–1219.
- Bravo-Valenzuela NJ, Peixoto AB, Nardozza LM, et al. Applicability and technical aspects of two-dimensional ultrasonography for assessment of fetal heart function. Med Ultrason. 2017;19(1):94–101.
- Bhorat IE, Bagratee JS, Pillay M, et al. Use of the myocardial performance index as a prognostic indicator of adverse fetal outcome in poorly controlled gestational diabetic pregnancies. Prenat Diagn. 2014;34(13):1301–1306.
- Hernandez-Andrade E, Benavides-Serralde JA, Cruz-Martinez R, et al. Evaluation of conventional doppler fetal cardiac function parameters: e /a ratios, outflow tracts, and myocardial performance index. Fetal Diagn Ther. 2012;32(1-2):22–29.
- Bhorat IE, Bagratee JS, Pillay M, et al. Determination of the myocardial performance index in deteriorating grades of intrauterine growth restriction and its link to adverse outcomes. Prenat Diagn. 2015;35(3):266–273.
- Bhorat I, Bagratee J, Reddy T. Gestational age-adjusted trends and reference intervals of the modified myocardial performance index (mod-MPI) and its components, with its interpretation in the context of established cardiac physiological principles. Prenat Diagn. 2014;34(11):1031–1036.
- Kwok SY, Yeung SS, Li VW, et al. Ventricular mechanics after repair of subarterial and perimembranous VSDs. Eur J Clin Invest. 2017;47(12):e12852.