Abstract
Objective
Extremely preterm infants have low Nuclear Receptor (NR) expression in their developing hepatobiliary systems, as they rely on the placenta and maternal liver for compensation. NRs play a crucial role in detoxification and the elimination of both endogenous and xenobiotic substances by regulating key genes encoding specific proteins. In this study, we utilized an Artificial Placenta Therapy (APT) platform to examine the liver tissue expression of NRs of extremely preterm ovine fetuses. This fetal model, resembling a “knockout placenta,” lacks placental and maternal support, while maintaining a healthy extrauterine survival.
Methods
Six ovine fetuses at 95 ± 1 d gestational age (GA; term = ∼150 d)/∼600 g delivery weight were maintained on an APT platform for a period of 120 h (APT Group). Six age-matched, in utero control fetuses were delivered at 99–100 d GA (Control Group). Fetal liver tissue samples and blood samples were collected at delivery from both groups and assessed mRNA expression of NRs and target transporters involved in the hepatobiliary transport system using quantitative PCR. Data were tested for group differences with ANOVA (p < .05 deemed significant).
Results
mRNA expression of NRs was identified in both the placenta and the extremely preterm ovine fetal liver. The expression of HNF4α, LRH1, LXR, ESR1, PXR, CAR, and PPARα/γ were significantly elevated in the liver of the APT Group compared to the Control Group. Moreover, target transporters NTCP, OATP1B3, BSEP, and MRP4 were upregulated, whereas MRP2 and MRP3 were unchanged. Although there was no evidence of liver necrosis or apoptotic changes histologically, there was an impact in the fetal liver of the ATP group at the tissue level with a significant increase in TNFα mRNA, a cytokine involved in liver inflammation, and blood elevation of transaminases.
Conclusion
A number of NRs in the fetal liver were significantly upregulated after loss of placental-maternal support. However, the expression of target transporter genes appeared to be insufficient to compensate role of the placenta and maternal liver and avoid fetal liver damage, potentially due to insufficient excretion of organic anions.
Introduction
Over the past two decades advances in respiratory and circulation management have greatly improved the mortality rate of extremely preterm infants (EPIs <1000 g); however, although better, the long-term neurodevelopmental outcomes for these infants have not improved to the same extent. Accordingly, about one-third of these infants exhibit some neurological abnormalities at three years of age [Citation1,Citation2], emphasizing the necessity for additional research to enhance our understanding of the delicate balance of perinatal homeostasis and how to improve the transition from the protected intrauterine environment to the outside world in an immature neonate.
The adequate detoxification and excretion of endogenous and xenobiotics are important for healthy fetal development and protection from organ injury. Detoxification and excretion of fetal-derived endogenous metabolites in the fetal period are strongly dependent on the placenta-maternal axis, rather than the fetal hepatobiliary metabolism and secretion as is the case in children and adults [Citation3]. Impaired placental transport systems of bile salts and maternal cholestasis have been found to contribute to imbalanced fetal blood hepatobiliary metabolites and adverse fetal neurodevelopmental outcomes [Citation4,Citation5]. In preterm infants, these events are even more pronounced. After early removal of the placental-maternal axis, these early newborns often develop hyperbilirubinemia, drug-induced hepatitis, and physiological cholestasis [Citation6]. In severe cases or extremely preterm infants (EPIs), this could have a detrimental impact on neurodevelopment and long-term outcomes, matters of continued concern in modern neonatal care [Citation7,Citation8].
Recently, key genes encoding proteins involved in bile salts synthesis, metabolism, and transport have been found to be under the transcriptional regulation of the nuclear receptors (NRs) in the liver and placenta [Citation3]. NRs are a group of transcription factors that, through ligand-binding, act as sensors to changes in environmental, developmental, pathophysiologic, and endocrine conditions and drive adaptive responses via gene regulation to maintain metabolic homeostasis [Citation9]. Reportedly, there is robust expression of NRs in the placenta and maternal liver, but little or no expression of NRs in the immature fetal liver [Citation3,Citation10], where gene expression of key enzymes is mediated by sulfation and glucuronidation, and the expression of hepatobiliary transporters is much lower than seen in the adult liver [Citation11–14]. Despite the importance of NRs in metabolic homeostasis and its crucial role to maintain detoxification and excretion of endogenous and xenobiotics their expression in the preterm liver remains poorly understood.
We have previously developed a pumpless extracorporeal membrane oxygenation system known as artificial placenta therapy (APT), with the aim of developing a new life support platform for EPIs. A key element of this system is the provision of gas exchange without the need to aerate the lungs. Since the development of APT, our team has successfully maintained 95d gestational age (GA) ovine fetus weighing ∼600 g (∼24 weeks’ human GA), for up to two weeks [Citation15]. Although the cardiovascular stability of our platform has been well described, the ability of these APT-treated fetuses to compensate for the loss of detoxification and excretion systems represented by the placental-maternal axis is unclear. Resolving this question is of great importance because, within our APT system, the responsibility for all detoxification processes is transferred to the fetal liver, without any additional dialysis or cholekinetic support being offered [Citation16,Citation17], a situation similar to the daily management of EPI in NICUs.
For the present report, our goal was to utilize our previously established artificial placenta platform to evaluate the capacity of extremely preterm ovine fetuses [Citation16] to adapt to the loss of detoxification and excretion functions typically provided by the placental-maternal axis during in utero development. In this study, we evaluated: (i) the existence and changes of hepatic NRs that regulate hepatobiliary metabolism and transport; (ii) changes in hepatobiliary transporters, which are the target genes of those NRs; and (iii) evidence of liver necrosis or apoptotic changes.
Materials and methods
Overview of experiments
The healthy growth and survival of extremely preterm ovine fetuses maintained on an APT platform for 120 h have been previously published [Citation16]. The molecular biological assessments of liver tissues, placenta, and blood samples presented in this study were conducted using samples from previously reported APT in utero control animals, and their respective age-matched in utero control animals [Citation16]. As the fetal liver is known to be susceptible to the adverse effects of perinatal asphyxia [Citation18], animals in which cerebral ischemic change associated with the induction or management of APT was detected were excluded from the present study. A randomly selected subgroup of six APT animals (the APT Group; n = 6) and six age-matched in utero control animals (the Control Group; n = 6) were used for this analysis.
Animal work
The ARRIVE guidelines for the reporting of animal experiments were followed in the present study. All procedures were performed in Perth, Western Australia, following review and approval by the Animal Ethics Committee of the University of Western Australia (RA/3/100/1378). The details of preoperative fasting, premedication, anesthesia, mechanical ventilation, and intravenous fluids management in pregnant Merino-cross ewes have previously been published [Citation16]. Ewes in both groups were euthanized with an intravenous bolus of pentobarbitone (160 mg/kg) following fetal delivery.
Summary of APT surgical procedure and maintenance
The details for the establishment and maintenance of APT have been described previously [Citation16]. As summary, APT Group animals were surgically delivered at 95 d GA under general anesthesia. Briefly, umbilical vessels were catheterized as follows: a catheter (Nipro Corporation, Osaka, Japan) was inserted into one umbilical vein and two umbilical arteries toward the fetus after a maternal laparotomy and hysterotomy. The APT circuit including the membranous oxygenator (Nipro Corporation, Osaka, Japan) was connected. The fetuses were promptly transferred into a bag (Nipro Corporation, Osaka, Japan) filled with synthetic amniotic fluid and maintained by heaters a fluid temperature of 38.7 ± 0.3 °C (group means ± SD). Meropenem (Ranbaxy, Sydney, Australia) and fluconazole (AFT Pharmaceuticals Pty Ltd, Sydney, Australia) due to prevent infection, and total parenteral nutrition were used in the maintenance of APT [Citation16]. Age-matched in utero control animals were also surgically delivered at 100 d GA under general anesthesia and immediately euthanized for sample collection.
Necropsy and tissue sample collections
APT Group animals were delivered after being maintained for 120 h. Control Group animals were delivered by cesarean section under general anesthesia. Both groups were euthanized at 99–100 d equivalent GA with intravenous pentobarbitone (160 mg/kg, Virbac, Milperra, Australia) after cord blood collection to measure biochemical data. Blood biochemical analyses were performed by an independent clinical pathology laboratory (Vetpath, Perth, Australia) as described previously [Citation16]. Placental tissue was collected from the APT Group animals at 95d GA induction, and from the Control Group animals at the time of delivery (99–100d GA). Fetal liver tissue was collected from both groups at 99–100d GA. These tissues were fixed in 10% formaldehyde and subsequently embedded in paraffin, or were snap-frozen in liquid nitrogen and stored at −80 °C for further molecular analysis.
Histological analysis
Liver tissues embedded in paraffin blocks were sectioned at a thickness of 7 μm. The tissue was stained with hematoxylin & eosin and a terminal deoxynucleotidyl transferase dUTP Nick End Labeling (TUNEL) staining, to assess the presence of apoptosis. Using a Tunel Assay kit-HRP-DAB (Abcam, Cambridge, United Kingdom). As a positive control, DNase I (Thermo Fisher Scientific, Waltham, MA) was used according to the previously described protocol to induce DNA strand breaks. All procedures were performed according to the manufacturer’s protocol. An investigator assessed the histological outcomes in a blinded fashion. Four fields of view on non-overlapping sections at 200× total magnification were randomly selected to count the necrotic cell or apoptotic cell number in each field.
Tissue RNA isolation and quantitative PCR
DNA-free total RNA was isolated from snap-frozen fetal liver and placenta using an RNeasy Plus Mini Kit (QIAGEN, Hilden, Germany). The concentration and quality of extracted RNA were quantified with a Qubit 2.0 fluorometer (Life Technologies, Carlsbad, CA). Cycling was performed using TaqMan probes (Applied Biosystems) and primer sets (Applied Biosystems) on a ViiA7 Real-Time PCR System (Applied Biosystems). The details of the probes are shown in the supplemental file (Supplementary Appendix 1).
Messenger RNA (mRNA) transcripts for the following ten major NRs were measured: HNF4α, LRH1, LXR, ESR1, FXR, PXR, CAR, PPARα, PPARγ, and VDR [Citation3]. The following six key target transporters were also measured in the liver: NTCP, OATP1B3, BSEP, MRP2, MRP3, and MRP4. In addition, mRNA transcripts for the following hepatic proinflammatory cytokines were measured: tumor necrosis factor-alpha (TNF-α), IL-1β, and IL-6. Amplification data for each gene were normalized to 18s ribosomal protein RNA as an internal reference. Data were processed to generate fold changes over the control value using a 2-ddCq method and determine the relative expression of transcripts for statistical analyses. If the result of the target RNA was below the detection sensitivity, the Cq values were set at 40.
Western blotting
Protein concentrations were measured by Pierce Rapid Gold BCA Protein Assay Kit (Thermo Fisher Scientific, Waltham, MA). An X-Cell SureLock Mini-Cell Electrophoresis System (Life Sciences) was used for electrophoresis and transfers following the manufacturer’s instructions. Membranes were incubated with No-Stain Protein Labeling Reagent (Invitrogen, Waltham, MA) to normalize total protein and incubated primary antibodies (PXR, CAR, and PPARα) overnight at 4 °C. Washed membranes with 0.05% Tween20 (Sigma) were incubated with Goat anti-Rabbit IgG (H + L) Highly Cross-Adsorbed Secondary Antibody, Alexa Fluor Plus 800 (Invitrogen, Waltham, MA). Membranes were imaged with iBright FL1000 Imaging System (Invitrogen, Waltham, MA), and relative densitometric band intensities per lane were the total of all bands in each lane and were used to normalize each NR band intensity. In addition, the same control samples were measured for each membrane due to normalizing differences between membranes. The details of antibodies are shown in the supplemental file (Supplementary Appendix 1). Five near-term (144d GA), null-treatment fetal livers previously collected as part of our ovine databank were assessed as positive control to confirm the identity of the NRs protein and the activity of the antibody.
Statistical analysis
Statistical analyses were performed using IBM SPSS Statistics for Windows (version 25.0; IBM Corp, Armonk, NY). A χ2 test was used to test the differences in nominal values between the two groups. All numerical data were tested for normality with Shapiro–Wilk tests. In the comparison of the two groups, t test was used for parametric data, whereas Mann–Whitney U-test was used for nonparametric data. Significance was accepted as p < .05.
Results
General characteristics of fetuses
All six APT Group animals completed the 120 h experimental period with equivalent growth to in utero Control Group animals (determined by fetal body weight; APT 1079 ± 112 g vs. Control 1063 ± 49.4 g) but without evidence of any circulatory failure, infection, and other severe complications. There were no significant differences in gestational age, sex ratio, and fetal body weight, at the conclusion of the 120 h study between the APT Group and the Control group ().
Table 1. Comparison of fetal general data at necropsy.
Biochemical data in fetal plasma
Aspartate aminotransferase (AST), alanine aminotransferase (ALT), gamma-glutamyl transpeptidase (GGTP), total bilirubin, albumin, and glutamate dehydrogenase (GLDH) exhibited statistically significant increases in the APT Group animals when compared to the Control Group animals. However, there was no notable difference in the levels of total bile acid (TBA) between the two groups ().
Table 2. Compared blood hepatic biochemical data in the APT Group with the Control Group.
Gene expression of nuclear receptors in the placenta
Alterations in the expression of nuclear receptor mRNA in the placenta were compared over the 95d GA and 100d GA period. There were no significant differences in the relative mRNA expression of hepatocyte nuclear factor 4 alpha (HNF4α), liver receptor homolog 1(LRH1), liver X receptor (LXR), estrogen receptor 1 (ESR1), peroxisome proliferator-activated receptor alpha and gamma (PPARα, PPARγ), farnesoid X receptor (FXR) and vitamin D receptor (VDR) (). Transcripts for constitutive androstane receptor (CAR) and pregnane X receptor (PXR) mRNA were below the detection limit of the PCR assay and not included in .
Figure 1. Changes in mRNA expression of NRs in the placenta from 95d to 100d GA. Box plots indicate relative fold changes of mRNA expression of NRs in 95d GA with 100d GA in the placenta. The yellow box indicates 95d GA and the green box indicates the 100d GA. *p < .05 vs. control (t test). NRs: nuclear receptors; GA: gestational age; HNF4α: hepatocyte nuclear factor-4 alpha; LRH1: liver receptor homolog-1; LXR: liver X receptor; ESR1: estrogen receptor-1; FXR: farnesoid X receptor; PPAR: peroxisome proliferator-activated receptor; VDR: vitamin D receptor.
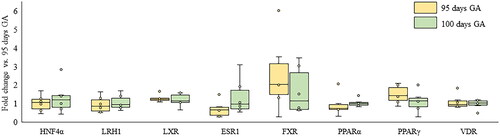
Gene expression of nuclear receptors in the fetal liver
The mRNA expression of hepatic NRs that regulate hepatobiliary metabolism and transport, represented by HNF4α, LRH1, LXR, ESR1, PXR, CAR, PPARα and PPARγ, were significantly elevated in the APT Group compared to the Control Group (). There were no significant differences in the relative expression of FXR and VDR between groups ().
Figure 2. Changes in mRNA expression of NRs in the fetal liver between APT Group and Control Group. Box plots indicated a relative fold change of mRNA expression of NRs in the APT Group with the Control Group in the fetal liver. (A-J) *p < .05 vs. control (t test). NRs: nuclear receptors; APT: artificial placenta therapy; HNF4α: hepatocyte nuclear factor-4 alpha; LRH1: liver receptor homolog-1; LXR: liver X receptor; ESR1: estrogen receptor-1; FXR: farnesoid X receptor; PXR: pregnane X receptor; CAR: constitutive androstane receptor; PPAR: peroxisome proliferator-activated receptor; VDR: vitamin D receptor.
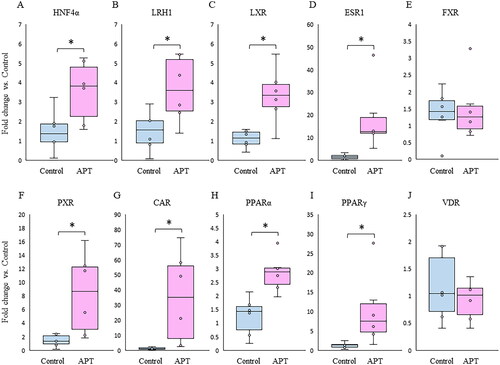
Gene expression of hepatobiliary transporters
Expression of the sinusoidal uptake transporter sodium taurocholate co-transporting polypeptide (NTCP), the organic anion transporting polypeptide 1B3 (OATP1B3), the canalicular efflux transporter bile salt export pump (BSEP) and multidrug resistance protein and the basolateral efflux transporter MRP4 were significantly elevated in the APT Group (), while there was no difference in the expression of multidrug resistance protein 2 (MRP2), and the basolateral efflux transporter MRP3 ().
Figure 3. Changes in mRNA expression of hepatobiliary transporters between APT Group and Control Group. Box plots indicated a relative fold change of mRNA expression of hepatobiliary transporters in the APT Group with the Control Group in the fetal liver. (A–C) BAs transporters are shown: uptake (NTCP) and efflux (BSEP and MRP4). (D–F) OA compounds transporters are shown: uptake (OATP1B3) and efflux (MRP2 and MRP3). *p < .05 vs. control (t test). **p < 0.05 vs. control (Mann-Whitney U-test). APT: artificial placenta therapy; BAs: bile acids; OA: organic anion; NTCP: sodium taurocholate co-transporting polypeptide; OATP1B3: organic anion transporting polypeptide-1B3; BSEP: bile salt export pump; MRP: multidrug resistance protein.
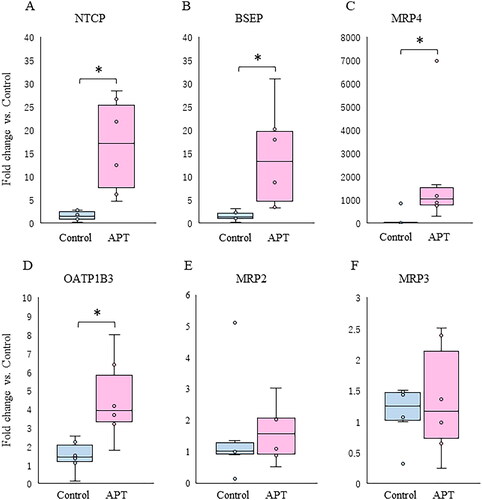
Inflammatory cytokines and histological assessment of liver tissues
TNF-α was significantly elevated in the APT Group. There was no significant difference in IL-6 or IL-1β between groups (). Furthermore, there were no inflammatory changes, mononuclear cell infiltrates, or necrotic hepatocytes in the sinusoids in the APT Group. The morphology of the liver parenchyma was normal, and hepatocytes were arranged orderly around the central vein (). Likewise, hepatocyte apoptosis was not observed in the APT Group when probed using TUNEL staining (). Due to the absence of necrotic cells or apoptotic cells with no differences detected between the groups, cell count data were not shown.
Figure 4. Inflammatory cytokines and histological assessment in the fetal liver. The expression of inflammatory cytokines TNF-α (A), IL-6 (B), and IL-1β (C) mRNA in the APT Group compared to the Control Group. Representative HE staining (magnification 200×) and TUNEL staining (magnification 200×) sections from the Control Group (D,F), the APT Group (E,G), and positive control treated DNase I(H) were shown. Red arrows indicate apoptotic changes by treated DNase I. Scale bar = 100 μm. *p < .05 vs. control (t test). TNF-α: tumor necrosis factor-alpha; APT: artificial placenta therapy; HE: Hematoxylin and Eosin; TUNEL: terminal deoxynucleotidyl transferase dUTP Nick End Labeling.
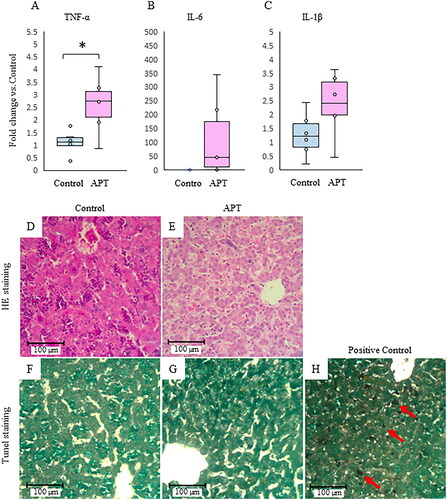
Discussion
The principal findings of this study are a significant increase in mRNA expression of NRs in the APT Group, suggesting that it may be altered at the level of transcription factors to compensate for the loss of placental-maternal axis, despite ovine fetuses being extremely preterm. This upregulation was associated with upregulated expression of bile acids (BAs) transporters; however, organic anion (OA) transporters were not changed during the experimental period. This might suggest that the excretion of OA compounds including bilirubin and drugs was not well-compensated compared to the excretion of BAs in the early period of life. Such a scenario could result in increased plasma total bilirubin and hepatic enzymes, and induce an inflammatory response in the fetal liver.
Nuclear receptors express in extremely preterm liver
It is known that the expression of NRs is low in the fetal liver [Citation3], although hepatic NRs are activated by ligands such as BAs, lipids, intermediate metabolites, and medications, and play critically important roles in regulating the transcription of enzymes and transporters involved in various metabolism and detoxification [Citation19–21]. In the present study, we demonstrated that mRNA expression of LXR, CAR, ESR1, LRH1, HNF4α, PXR, and PPARα/γ are significantly increased in the APT Group livers compared with the Control Group (). Since there were no notable disparities in the mRNA expression of NRs in the placenta between 95 d and 100 d at the period of APT experiment (), we hypothesized that the disruption of the interactive excretion system for hepatobiliary metabolites following the removal of the placental-maternal axis, and the drug loading for fetal maintenance could be the main factor underlying these elevated hepatic NRs at the transcriptional level ( and ).
On the contrary, there was no significant change in the mRNA expression of FXR between the Groups ( and ). Since BAs ligands are signaling molecules that directly activate FXR to regulate biliary metabolism in the liver, FXR is usually activated by an imbalance of biliary circulation such as cholestasis or significant liver injury, then suppresses BA uptake transporters and synthesis as negative feedback [Citation20]. In this experimental platform, there is the absence of enteral feeding, which could impact the bile load associated with BAs synthesis and enterohepatic circulation. Therefore, presumably, as the systemic pooled level of BAs was maintained within an acceptable range constantly, FXR was not upregulated In the APT Group ().
Figure 5. Overview of changes in fetal hepatic NRs and hepatobiliary transporters. Activated NRs by ligands (BAs, bilirubin, and drugs) promote (pink) or suppress (blue) expression of other NRs or target transporters. Increased mRNA expressions were highlighted in red. NRs: nuclear receptors; APT: artificial placenta therapy; BAs: bile acids; HNF4α: hepatocyte nuclear factor-4 alpha; LRH1: liver receptor homolog-1; LXR: liver X receptor; ESR1: estrogen receptor-1; FXR: farnesoid X receptor; PXR: pregnane X receptor; CAR: constitutive androstane receptor; PPAR: peroxisome proliferator-activated receptor; VDR: vitamin D receptor; NTCP: sodium taurocholate co-transporting polypeptide; OATP1B3: organic anion transporting polypeptide-1B3; BSEP: bile salt export pump; MRP: multidrug resistance protein.
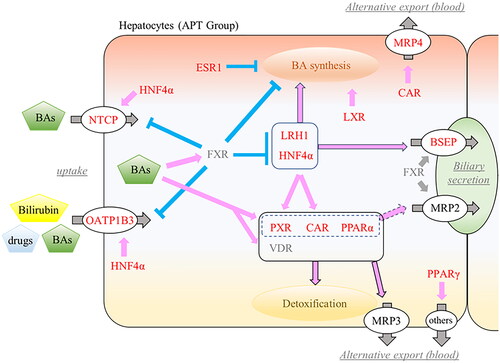
Nuclear receptors effects on target genes transporters
Hepatobiliary transporters are recognized as important components of detoxification and excretion of endogenous and xenobiotics, which mainly transport them composed of BAs and OA compounds including bilirubin and a lot of medications [Citation22]. With upregulated NRs, the mRNA expression of NTCP, OATP1B3, BSEP, and MRP4 was significantly elevated in the fetal liver of the APT Group (). On the contrary, the expression of MRP2 and MRP3, which are known OA compounds selective transporters, as targeted genes by activated PXR, CAR, and PPARα [Citation23,Citation24], was unchanged between the APT Group and the Control Group (). Interestingly, protein expressions of PXR, CAR, and PPARα in the APT Group were quite low (Supplementary Appendix 2), suggesting that there were temporary delays between mRNA and protein expressions.
In fetal rat liver, mRNA expression of Mrp2 and Mrp3 can exist at the canalicular membrane of hepatocytes from early gestation [Citation25], but mRNA and protein expression can require several weeks after birth to reach full adult expression [Citation26]. These results are consistent with a temporary delay between the onset of extrauterine management and the expression of hepatobiliary OA transporters. In other words, the delayed expression of hepatobiliary OA transporters could potentially lead to excretion disorders of OA compounds after birth, even if they are upregulated at the transcriptional factor level.
It is worth noting that blood biochemical tests after 120 h APT showed a significant increase in total bilirubin, as well as in liver transaminases (higher AST than ALT) (, ). In addition, hepatic TNFα was also increased although we have previously reported normal levels of TNFα in plasma and lung tissues taken from these experimental animals [Citation16]. Thus, increasing hepatic TNF α is assumed to indicate a liver-specific inflammatory process accompanied by elevated liver transaminases. Hepatic TNFα is an early pro-inflammatory cytokine mediator that plays a significant role in inducing hepatocytes apoptosis [Citation27,Citation28], suggesting mild acute hepatitis had developed in the APT Group, although histologically there was no evidence of hepatocyte apoptosis or necrotic changes (). Clinically, postnatal increase in liver transaminases often presents as a reversible liver injury in EPIs, however, the etiology of these changes is not well understood [Citation29]. Given that all APT Group animals were free from ischemic change and infection, insufficient compensation for extrahepatic excretion of OAs on EPIs’ liver, in particular, hyperbilirubinemia and excessive drug administration in the first week after birth could develop into a liver injury.
Limitations and challenges
There are a number of limitations to assess when interpreting the data presented in this study. We did not include time course groups with a longer survival under APT management, along with age-matched in utero controls. Based on the increased NR expression, it is important to note the time-course change in the expression of each target gene and the histological maturation of hepatocytes at several time points – especially at longer maintenance intervals.
As this study was the first trial assessing fetal liver functions using the APT ovine model, the scope of evaluated genes was limited. It would be ideal for future experiments to assess a time course change of comprehensive gene expressions including downstream signaling analysis of NRs using an unbiased (e.g. bulk RNA sequencing) technique. An additional experimental limitation of importance is the absence of a Control Group employing ventilated extremely preterm ovine fetuses. With these results in mind, ventilated control animals would be the next key in additionally evaluating liver injury in EPIs as current neonatal care based on ventilation and enteral nutrition is also absent from maternal-placental support.
Conclusions
We report the expression of NRs in the extremely preterm ovine liver and placenta. The placental expression of NRs did not change over the duration of the experiment. In contrast, the expression of NRs in the fetal liver was significantly increased after early removal of the placental-maternal axis. However, the expression of a number of target OA transporters was not elevated over the 120 h experimental period. These molecular changes were observed in conjunction with elevated markers of liver injury and bilirubin. This pattern suggests that, despite increased NRs expression, there was insufficient fetal detoxification and excretion of endogenous and xenobiotics, potentially resulting in observed acute inflammatory changes in the fetal liver. In addition to providing a better understanding of the interplay between the fetus and the placental-maternal detoxification axis, future comprehensive investigations of the expression of whole NRs and metabolic pathways in the extremely preterm liver may assist in the development of new neonatal management techniques to improve EPI outcomes. Despite the limitations, these experiments also illustrate the crucial role of the placenta in maintaining fetal homeostasis and the necessity of taking this role into account when planning experiments involving artificial placentas. From a clinical point of view, the significance of hepatic damage when caring for extremely premature infants owing to their underdeveloped liver function cannot be overstated. These results add to the view that premature infants, born well before their physiological systems are fully matured, are particularly vulnerable to a range of health challenges, including those related to hepatic function.
Presentation
This work has previously been presented, in part, at the 2023 SRI annual meeting in Brisbane, Australia and features in the conference abstract book.
Supplemental Material
Download Zip (266.9 KB)Acknowledgements
We appreciate the support of Nipro Corporation, Osaka, Japan (donation of Artificial Placenta circuit consumables) and Siemens Australia (generous RP500 consumables).
Disclosure statement
Author Shinichi KAWAMURA is employed by Nipro Corporation. The remaining authors declare that the research was conducted in the absence of any commercial or financial relationships that could be construed as a potential conflict of interest.
Data availability statement
The data that support the findings of this study are openly available in Figshare.
Appendix 1: http://doi.org/10.6084/m9.figshare.24550780.
Appendix 2: http://doi.org/10.6084/m9.figshare.24180687.
Additional information
Funding
References
- Kusuda S, Hirano S, Nakamura T. Creating experiences from active treatment towards extremely preterm infants born at less than 25 weeks in Japan. Semin Perinatol. 2022;46(1):1. doi: 10.1016/j.semperi.2021.151537.
- Bell EF, Hintz SR, Hansen NI, et al. Mortality, in-Hospital morbidity, care practices, and 2-Year outcomes for extremely preterm infants in the US, 2013-2018. JAMA. 2022;327(3):248–12 doi: 10.1001/jama.2021.23580.
- Marin JJG, Macias RIR, Briz O, et al. Molecular bases of the fetal liver–placenta–maternal liver excretory pathway for cholephilic compounds. Liver International. 2008;28(4):435–454. doi: 10.1111/j.1478-3231.2008.01680.x.
- Macias RI, Marin JJ, Serrano MA. Excretion of biliary compounds during intrauterine life. World J Gastroenterol. 2009;15(7):817–828. doi: 10.3748/wjg.15.817.
- Blazquez AG, Briz O, Gonzalez-Sanchez E, et al. The effect of acetaminophen on the expression of BCRP in trophoblast cells impairs the placental barrier to bile acids during maternal cholestasis. Toxicol Appl Pharmacol. 2014;277(1):77–85. doi: 10.1016/j.taap.2014.02.019.
- Nishiura H, Kimura A, Yamato Y, et al. Developmental pattern of urinary bile acid profile in preterm infants. Pediatr Int. 2010;52(1):44–50. doi: 10.1111/j.1442-200X.2009.02887.x.
- Morioka I. Hyperbilirubinemia in preterm infants in Japan: new treatment criteria. Pediatr Int. 2018;60(8):684–690. doi: 10.1111/ped.13635.
- Potter C. The role of a NICU hepatology consult service in assessing liver dysfunction in the premature infant. JPGN Rep. 2021;2(1):e031. doi: 10.1097/PG9.0000000000000031.
- Romagnolo DF, Zempleni J, Selmin OI. Nuclear receptors and epigenetic regulation: opportunities for nutritional targeting and disease prevention. Adv Nutr. 2014;5(4):373–385. doi: 10.3945/an.114.005868.
- Lee JS, Ward WO, Knapp G, et al. Transcriptional ontogeny of the developing liver. BMC Genomics. 2012;13(1):33. doi: 10.1186/1471-2164-13-33.
- Ladumor MK, Bhatt DK, Gaedigk A, et al. Ontogeny of hepatic sulfotransferases and prediction of age-Dependent fractional contribution of sulfation in acetaminophen metabolism. Drug metabolism and Drug Metab Dispos. 2019;47(8):818–831. doi: 10.1124/dmd.119.086462.
- Grimmer I, Moller R, Gmyrek D, et al. [Bilirubin UDP-glucuronyltransferase activity in human fetal liver homogenates]. Acta Biol Med Ger. 1978;37(1):131–135.
- Tomer G, Ananthanarayanan M, Weymann A, et al. Differential developmental regulation of rat liver canalicular membrane transporters bsep and Mrp2. Pediatr Res. 2003;53(2):288–294. doi: 10.1203/01.PDR.0000047509.54253.01.
- Zinchuk VS, Okada T, Akimaru K, et al. Asynchronous expression and colocalization of bsep and Mrp2 during development of rat liver. Am J Physiol Gastrointest Liver Physiol. 2002;282(3):G540–8. doi: 10.1152/ajpgi.00405.2001.
- Usuda H, Ikeda H, Watanabe S, et al. Artificial placenta support of extremely preterm ovine fetuses at the border of viability for up to 336 hours with maintenance of systemic circulation but reduced somatic and organ growth. Front Physiol. 2023;14:1219185. doi: 10.3389/fphys.2023.1219185.
- Usuda H, Watanabe S, Saito M, et al. Successful use of an artificial placenta to support extremely preterm ovine fetuses at the border of viability. Am J Obstet Gynecol. 2019;221(1):69.e1–69.e17. doi: 10.1016/J.AJOG.2019.03.001.
- Usuda H, Watanabe S, Saito M, et al. Successful use of an artificial placenta–based life support system to treat extremely preterm ovine fetuses compromised by intrauterine inflammation. Am J Obstet Gynecol. 2020;223(5):755.e1-755–e20. doi: 10.1016/J.AJOG.2020.04.036.
- Hankins GDV, Koen S, Gei AF, et al. Neonatal organ system injury in acute birth asphyxia sufficient to result in neonatal encephalopathy. Obstet Gynecol. 2002;99(5 Pt 1):688–691. doi: 10.1016/S0029-7844(02)01959-2.
- Chiang JYL. Hepatocyte nuclear factor 4α regulation of bile acid and drug metabolism. Expert Opin Drug Metab Toxicol. 2009;5(2):137–147. doi: 10.1517/17425250802707342.
- Chiang JYL. Bile acid regulation of gene expression: roles of nuclear hormone receptors. Endocr Rev. 2002;23(4):443–463. doi: 10.1210/ER.2000-0035.
- Edwards PA, Kennedy MA, Mak PA. LXRs;: oxysterol-activated nuclear receptors that regulate genes controlling lipid homeostasis. Vascul Pharmacol. 2002;38(4):249–256. doi: 10.1016/S1537-1891(02)00175-1.
- Trauner M, Boyer JL. Bile salt transporters: molecular characterization, function, and regulation. Physiol Rev. 2003;83(2):633–671. doi: 10.1152/physrev.00027.2002.
- Kast HR, Goodwin B, Tarr PT, et al. Regulation of multidrug resistance-associated protein 2 (ABCC2) by the nuclear receptors pregnane X receptor, farnesoid X-activated receptor, and constitutive androstane receptor. J Biol Chem. 2002;277(4):2908–2915. doi: 10.1074/jbc.M109326200.
- Zollner G, Trauner M. Nuclear receptors as therapeutic targets in cholestatic liver diseases. Br J Pharmacol. 2009;156(1):7–27. doi: 10.1111/j.1476-5381.2008.00030.x.
- St-Pierre MV, Stallmach T, Freimoser Grundschober A, et al. Temporal expression profiles of organic anion transport proteins in placenta and fetal liver of the rat. Am J Physiol Regul Integr Comp Physiol. 2004;287(6):R1505–R1516. doi: 10.1152/AJPREGU.00279.2003/ASSET/IMAGES/LARGE/ZH60120424870006.JPEG.
- Gao B, St Pierre MV, Stieger B, et al. Differential expression of bile salt and organic anion transporters in developing rat liver. J Hepatol. 2004;41(2):201–208. doi: 10.1016/J.JHEP.2004.04.029.
- Horn TL, O'Brien TD, Schook LB, et al. Acute hepatotoxicant exposure induces TNFR-Mediated hepatic injury and cytokine/apoptotic gene expression. Toxicol Sci. 2000;54(1):262–273. doi: 10.1093/toxsci/54.1.262.
- Shimizu S, Yamada Y, Okuno M, et al. Liver injury induced by lipopolysaccharide is mediated by TNFR-1 but not by TNFR-2 or fas in mice. Hepatol Res. 2005;31(3):136–142. doi: 10.1016/j.hepres.2004.11.012.
- Victor S, Dickinson H, Turner MA. Plasma aminotransferase concentrations in preterm infants. Arch Dis Child Fetal Neonatal Ed. 2011;96(2):F144–5. doi: 10.1136/adc.2008.152454.