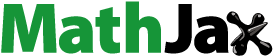
ABSTRACT
In this article, an idea has been presented that utilizes the heat produced from the petroleum gas burning accompanying the process of oil extraction to enhance the solar chimney performance. The simulation software tool ANSYS Fluent 2020R1 was used to know the possibility of exploiting this waste energy of gas and combining it with a solar chimney. Seven gas channel configurations were tested on the solar chimney to determine which can produce the most electricity. The simulation results showed that this idea could be exploited to produce higher electric energy, and the efficiency of the solar chimney was increased so that the electric energy production process continues for 24 hours instead of production during the sunshine period only. Different designs were considered for the channels inside the solar collector. Case1 and Case2 were meant for validation purposes only. Good agreement was achieved with the previous research with a maximum error of 2%. The results showed that the circular design of the two-ring burner was the best choice which gave the optimum electric power from the system of about 121.1 kW. It was noted that the circular design of the two-ring burner achieved the highest thermal efficiency, which amounted to 0.523%.
1. Introduction
The problem of energy availability is one of the most important problems that humanity faced in ancient and modern times. Since the industrial revolution, reliance on fossil fuels (coal, oil and natural gas) has increased to operate factories and provide the requirements of daily life for people (Guo et al. Citation2019; Ibrahim, Algburi, and Ahmed Citation2023). This increasing demand for fossil fuels has led to a significant decrease in reserves of conventional fuel and an increase in environmental pollution, which has led to a rise in the global temperature today (Mahmood Ibrahim, Ibraheem, and Bakr Weli Citation2023). The problem of the depletion of fossil fuels and the environmental pollution associated with it prompted scientists and researchers to search for new sources of energy, and solar energy topped the priorities of scientists in this regard (Habibollahzade et al. Citation2021). Solar energy is characterized by its availability in most countries of the world and its need for complex technology, and its ease of use for various purposes. However, what is wrong with this solar energy is that it is not available at night times and on cloudy days, which requires the presence of systems to store this type of energy, which increases its cost (Abbas and Aziz Citation2022). The use of solar energy to generate electricity is the most important application at present, and the most important of these applications are solar cells (Gomaa, Ahmed, and Rezk Citation2022), solar chimneys (Hussein and Ahmed Citation2018), solar ponds (Aweid, Ahmed, and Algburi Citation2022), and other applications.
A passive, non-mechanical technique of producing energy is a solar chimney principle, often referred to as a thermal chimney. Hot air naturally rises, and a solar chimney uses the principles of convection cooling to pull cooler air to the bottom while releasing hot air out the top. There is evidence that suggests the Romans utilized solar chimneys in Europe and the Middle East centuries ago. A solar chimney is a method of converting solar energy into electricity based on the greenhouse effect. The solar chimney consists of three main parts (): the solar collector, the chimney, and the wind turbine. Solar chimneys are characterized by low efficiency, and for this reason, engineers and scientists are constantly trying various means to increase this efficiency. Improving efficiency is done by adding external sources of heat from other systems, such as power stations (Zou and He Citation2015), solar cells (Ahmed et al. Citation2022), a solar pond (Akbarzadeh, Johnson, and Singh Citation2009), or underground heat sources (Cao et al. Citation2014). In Manzanares, Spain, the first solar chimney prototype was put into service in 1982. It had a maximum production capacity of 50 kW and ran continually for roughly seven years (Kiwan, Al-Nimr, and Salim Citation2020). The solar chimney had a tower radius of 5.08 meters and a height of 194.6 meters. The collector had a 122-meter diameter, and its inlet was 1.85 meters high. Scientists and engineers are currently trying to improve the performance of solar chimneys by using several design and operational variables.
Figure 1 . The main parts of the solar chimney (Cuce et al. Citation2022).

Flare gas and oilfield gas are other names for associated petroleum gas. ‘Associated gas’ is a common name for natural gas that originates from oil wells. This gas may be present in the formation alone (as free gas), or it may dissolve in crude oil. Regardless of its origin, natural gas commonly exists in combinations with other hydrocarbons, including ethane, propane, butane, and pentanes, after being extracted from crude oil. Additionally, nitrogen, water vapor, carbon dioxide, hydrogen sulphide, and some other substances are present in raw natural gas. Since it is recovered during the oil production process, associated gas carrying these pollutants cannot be used untreated and is difficult to transport (US Energy Information Adminsitration (EIA) Citation2017). Recent studies have shown a minor drop in recent years; nonetheless, it is still estimated that 140 billion cubic metres of gas are flared annually throughout the world. That amounts to $20 billion in dollars, but the penalty for wasting petrol extends beyond that because burning it releases as much carbon dioxide into the sky each year as 200 million cars do. Gas flaring and venting have existed alongside crude oil extraction ever since it started in the nineteenth century. If crude oil output keeps rising, related gas will need to be flared and vented, according to businesses and some governments. However, since the usefulness of gas as an energy source and its advantages for the environment have become increasingly apparent, some governments have passed legislation to minimize gas flaring. The World Bank predicts that without investment, gas flaring reached a level of roughly 140 billion cubic meters in 2017. Nevertheless, the issue persists with making significant efforts to utilize associated gas and leverage the savings in power generation and raw materials for the production of fertilizers and petrochemicals that will follow from doing so. Nearly 24 million barrels of crude oil were produced daily in Arab nations, while 21.8 billion cubic meters of gas were flared. Iraq accounted for the majority of the rise in gas flaring, as oil output climbed from 3.0 to 4.5 million barrels per day, while gas flaring increased from 13.3 to 17.8 billion cubic meters. According to reports, Iraq wastes almost 62% of the gas it produces, or 196,000 barrels of crude oil each day (US Energy Information Adminsitration (EIA) Citation2017). The resource, valued at around $45 billion at $70 per barrel, would have been sufficient to launch a brand-new gas industry (BP Citation2022).
Many researches and studies have been conducted to enhance the performance of solar chimneys. By taking into account the reverse fan model, Rabehi et al. (Rabehi et al. Citation2017) used the ANSYS Fluent program to construct and simulate the solar chimney. The Spanish model was used to illustrate the usage of numerical simulation in conjunction with a turbine model. The results showed that the parameters of flow and heat transmission were directly impacted by variations in solar radiation. The turbine’s pressure decreases significantly reduced energy production but had only a little effect on collector efficiency. The solar chimney was quantitatively analyzed by Bouabidi et al. (Bouabidi et al. Citation2019) under Tunisian meteorological conditions. To examine the impact of chimney diameter, they ran numerous test simulations. They looked at the features of airflow at different diameters. The simulation found that as the chimney’s diameter grew, so did the velocity. As a result, when the diameter expanded, the solar chimney’s efficiency rose. Consequently, it was believed that the solar chimney power plant was a practical approach to using solar energy in Tunisia. Through the use of a computational fluid dynamics (CFD)-based real turbine and three-dimensional modelling of the technical requirements for the model of Manzanares, a numerically based model of a solar chimney was made by Gholamalizadeh and Chung (Gholamalizadeh and Chung Citation2017). The results showed that the backwards fan model performed nearly as well as the real turbine while having a pressure drop that was 43.7% lower. Ismail et al. (Ismail et al. Citation2019) computational research looked at potential modifications to the heat transfer and airflow of a conventional chimney model. This study discovered that the surge in failed controllers caused an increase of about 7% in the velocity at the chimney opening. Hassan et al. (Hassan, Ali, and Waqas Citation2018) used ANSYS Fluent to run a numerical simulation that measured how varying the solar collector slope and chimney angle in their model of a solar chimney affected the amount of electricity generated. The results showed that the air velocity increased steadily due to the collector’s higher gradient. However, results showed that the flow was not stable when the tilt of the collector kept more than 6° and that air recycling beneath the collector was caused by density gradients brought on by the uneven temperature distribution, which might block air from entering the chimney. Djaouida et al. (Djaouida et al. Citation2020) conducted a mathematical study of the solar chimney. The power plant’s flow characteristics from the solar chimneys in Manzanares were simulated. The electricity output of the factory was regulated using safe and adaptive technology. To do this, they modified the reference station design by adding a new roof to the existing structure. This research established that the structure of the secondary collector roof increased the station’s power output from the primary load. A three-dimensional numerical model of a solar chimney that incorporates solar radiation, solar load, and a turbine was proposed by Guo et al. (hua Guo, yin Li, and Wang Citation2014). The system’s output was then extensively analyzed in light of variables like solar input, turbine pressure, and ambient temperature. The outcomes demonstrated that radiation heat transfer must be taken into account in the numerical models. The results also showed that the high temperature of the air was not significantly affected by the variation in atmospheric temperature but that it was significantly affected by the variation in air velocity. The power production of the plant was found to be relatively unaffected by ambient temperature within the normal range for the day. Zuo et al. (Zuo et al. Citation2021) created a cost–benefit analysis model for both onshore (WSCPPDW) and offshore (SCPPDW) solar-fired combined-cycle power and desalination-waste-heat facilities. The economic performance of the two systems and the WSCPPDW were evaluated using the net present value (NPV) method after optimization. Net income (NET) and net present value (NPV) both went up by 102.5% when a wind surcharge scheme was implemented. While both systems’ annual NET expanded with time, their yearly NPV fluctuated between increases and decreases. To calculate and assess flow and efficiency parameters for a solar chimney station, Das and Chandramohan (Das and Chandramohan Citation2018) conducted a 3D numerical simulation model. The effects of geometric factors like chimney height and solar collector roof angle were researched. The governing equations were solved using discrete ordinates (DO) and the radiation disturbance model (RNG). The findings demonstrated that altering the roof angle of the solar collector improves air velocity while lowering the air temperature. Increasing the chimney’s length from 3 to 8 meters resulted in a 31% increase in velocity. The efficiency of the solar collector was calculated to be 81.4%, the chimney efficiency to be 0.0465%, and the power output to be 0.255w. Setareh (Setareh Citation2021) investigated the geometric parameters effects like the wind speed, chimney divergence angle, and roof collector angle on the solar chimney performance. According to the results, the power output of the SCPP grew by for a constant a. It was also suggested that, for optimal SCPP output, the ratio of turbine pressure drop to total pressure potential should be between 0.7 and 0.85. This investigation also uncovered a noteworthy increase in wind velocity.
Due to the low efficiency of solar chimneys, scientists and engineers have tried to enhance the efficiency of the solar chimneys systems by mixing them with other energy systems, whether renewable or conventional systems, which led to the emergence of the so-called hybrid solar chimneys (Ahmed et al. Citation2022). A proposal was made by Habibollahzade et al. (Habibollahzade et al. Citation2018) to take advantage of waste energy in Tehran and use it as an auxiliary heat source for the solar chimney system. The novel system was assessed through standard analysis of energy, energy, exercise economic and atmospheric viewpoints. Obtainability efficiency, total energy production, and total system cost were monitored and correlated throughout the day and night. Fathy et al. (Fathi et al. Citation2018) used waste heat from a nuclear power plant to improve the performance of a solar chimney. Computational fluid mechanics technology was used to analyze the performance of the innovative system. This increased the efficiency of the thermal nuclear power plant by 42%. Habibollahzade et al. (Fathi et al. Citation2018) suggested a new biomass energy system integrated with the solar chimney. The design is achieved using the warm air of the condenser’s outlet Tehran thermal power plant into the solar chimney. The total efficiency of the system increased by 0.12%. According to Al-Kayiem et al. (Al-Kayiem et al. Citation2019), the hot air was supplied to the chimney’s solar collector through the flue-gas channel. An experimentation setup was developed for this. The ANSYS-Fluent software has also been used to simulate the proposed system. To reduce the enormous amount of energy lost in gas flaring processes and to enhance SCPP performance, Esmaili et al. (Esmaili et al. Citation2022) make an effort to numerically model a combination of solar chimneys and flare gas. A three-dimensional model was created. The findings of this study showed that this combination effectively increases power generation from 50 to 788 kW while reducing the plant’s chimney height by half. It also prevents power generation from declining and increases it by four times, from 50 to 215 kW. Ahmed and Hussein (Ahmed and Hussein Citation2018) presented designs to improve the efficiency of solar chimneys using solar PV panels. Two prototypes of a hybrid solar chimney were constructed and considered. With a chimney that was 2 metres high, the first system had a collector glass roof cover, a PV panel served as the absorber, and the second system used a PV panel as the collector roof cover. The goal of the researchers is to improve the efficiency of solar chimneys. By reviewing previous research, it has been found that integrating the solar chimney system with flare gas has received little attention despite the high thermal energy wasted in oil extraction operations. The purpose of this paper is to investigate the potential of coupling solar chimneys with waste energy from burning gas associated with crude oil to increase the efficiency and electric power production of solar chimney power plants. The specific goals of this research are to:
Understand the principles of solar chimney power plants and how they can be coupled with waste energy sources.
Assess the potential benefits of coupling solar chimneys with waste energy sources in terms of efficiency and power production.
Identify the challenges and limitations of this approach.
This study suggests that integrating solar chimneys with waste energy sources could boost power production and efficiency. This could make solar chimney power plants a better electricity generator, especially in waste energy-rich areas. This discovery may lead to more efficient and powerful solar chimney power plants. This might cut fossil fuel use and create a cleaner, more sustainable energy future.
2. Proposed model
Facilities for related gas power generation are typically found in outlying locations. If there is any power supply at all, it is frequently insufficient in these places. Therefore, using diesel generator sets was a typical method in the past. This strategy is becoming less and less viable given the sharp increase in the price of oil and, consequently, diesel fuel. Additionally, the cost of providing diesel fuel and the requisite storage is rising. When associated petroleum gas is formed, it is frequently suitable for use with other technologies and combustion in other applications. The mechanism of the solar chimney works depends on increasing the global warming process inside the solar collector as a result of the solar radiation quantities falling on the solar collector, which allows the passage of solar radiation quantities into it. As well as the process of getting rid of the gas associated with oil extraction by burning and disposing of it, it is possible to take advantage of the burning process of associated gas in the open air and make the burning process take place inside the solar collector by extending pipes carrying associated gas inside the solar collector with specific designs that ensure the distribution of the combustion process inside the solar collector evenly to ensure the process of pressure permeation distribution within the solar collector to ensure the movement of air currents evenly on the turbine blades to ensure smooth generation inside the solar chimney, as shown in . Seven designs were implemented on the solar chimney by designing different configurations for the gas channel inside the solar collector to check for the optimum case to produce maximum electric power. shows the dimensions of the models proposed in the current study. The first and second designs are two small chimneys, one containing a channel to pass the burning gas, and the second is a traditional chimney with a collector diameter of 6 m, and the distance between the glass cover and the chimney floor is 0.3 m. The height of the chimney is 6 m, and its diameter is 0.15 m. The purpose of these two designs was to demonstrate the validation of the numerical mathematical model with the results of Al-Kayiem et al. (Al-Kayiem et al. Citation2019), and the results of this model gave acceptable and close values. The other five designs were the diameter of the collector, the chimney and the height of the chimney were enlarged, and then the shape of the gas channel was manipulated. The designs are as follows:
Figure 2 . Different gas channels configurations of the solar chimney. (a) Model 1: Without gas channels; (b) Model 2: With gas channels; (c). Model 3: Long gas channels (gas outlet is out of collector); (E) Model 4: Long gas channels (gas outlet is in the collector); (F) Model 5: Short gas channels (gas outlet is in the collector); (G) Model 6: One circular gas channel; (H) Model 7: Two circular gas channels.

Table 1 . Solar chimney configurations and dimensions.
3. Methodology of the present work
3.1. Numerical procedure
The Navier-Stokes equations and the conservation laws of mass and energy were solved in Ansys Fluent, a commercial product developed by Ansys, Inc. in Pennsylvania, USA. Additionally, the body force weighted technique was used as a tool for determining the pressure limit, the COUPLE algorithm was used to characterise the relationship between pressure and velocity, and the RNG turbulence model k-e was chosen to describe the flow (Ahmed Citation2018). Upwind techniques of the second order were used to factor in the remaining equations. The double precision option was used for all numerical calculations, which produces more precise results. For all equations, including the energy equation, the maximum iteration error was set to 10−8 (Ahmed Citation2018). To perform the simulation of the system and extract accurate results, it is necessary to rely on several hypotheses that will facilitate the solution process and be an approach to the experimental work. As a result, the following theories were used as the foundation for the solution:
1- The system is in a steady state, meaning that heat transfer and flow have stabilized.
2- Since air cannot be compressed, the Boussinesq method is used to calculate its density as:
(1)
(1)
3- Convection and radiation losses account for the majority of the heat lost via the collector cover.
4- The flow is turbulent, as the Rayleigh number is greater than 108.
5- The air properties are considered constant as the system is not at a high altitude.
6- The ambient temperature is equal to the inlet temperature of the solar collector.
3.2. Mathematical model governing equations
The buoyancy force, commonly represented by the Rayleigh number, is responsible for the flow in solar chimneys (Pratap Singh, Akshayveer, and Singh Citation2020). It is commonly asserted in the literature that, similar to the Spanish prototype, solar chimney power plants must have a turbulent interior airflow since the Rayleigh number is typically greater than 1010. That’s why has been used the RNG kappa-epsilon turbulence model. Here are the software-solved governing equations (Malalasekera Citation2007):
Continuity equation:
(2)
(2)
Energy equation:
(3)
(3)
Momentum equation:
(4)
(4)
K-epsilon equations:
(5)
(5)
(6)
(6)
where the values of the constants in the common k- epsilon model are:
The flow velocity at the chimney base varied by the results of the numerical simulations for different grid sizes. The test was run with six distinct element counts: 80,000, 300,000, 700,000, 1,300,000, 2,000,000, and 2,750,000. Each try was numerically simulated using the same boundary conditions. The flow velocity fluctuation at the chimney base for different densities of cells is depicted in .
Thermal efficiency is the most important variable that is relied upon to compare the performance of the different designs presented in the current article. The thermal efficiency of the solar chimney is calculated from the following equation:
(7)
(7)
: Density of air (kg/m3).
The cross-sectional area of the chimney at the base (m2).
: Air velocity at the base of the chimney (m/s).
: Solar radiation (W/m2).
: Solar collector area (m2).
3.3. System boundary conditions
The boundary conditions of the system are shown below in , where the ambient environment temperature was set for each month depending on the values of the station location. The temperature of the ground floor was set in the arithmetic field to be equal to the ambient temperature. Atmospheric pressure was specified as the maximum allowable pressure at both the solar collector’s inlet and the solar chimney’s flue outlet. Using the mixed limit condition, the heat transfer coefficient is determined, and the limit value of the external collector surface is set to be forced heat convection in addition to long-wave radiation (ANSYS Citation2013).
Table 2 . Current simulation boundary conditions.
3.4. Validation of the numerical simulation
For the traditional SC model, both Case 1 and 2 were considered for the validation, the two cases were designed as same as the experimental work of Al-Kayiem et al. (Al-Kayiem et al. Citation2019). All the dimensions and working parameters of the researcher were applied to our cases 1 and 2 for the best results. The computational simulation’s predicted temperature and velocity values at the chimney base correspond well with their observations and don’t exceed 2%, indicating a rational and tolerable error ratio. -a shows the velocity comparison findings and the results of the temperature comparison are displayed in -b. The experimental observations and modelling prediction findings show that air temperature rise becomes effective after 400 W/m2 of solar radiation, which is consistent with our present results.
Figure 4 . Validation of the present model with the work measurements of Al-Kayiem et al. (Al-Kayiem et al. Citation2019). (a) Present work velocity validation with (Al-Kayiem et al. Citation2019); (b) Present work Temperature rise validation with (Al-Kayiem et al. Citation2019).

4. Results and discussions
To increase the efficiency of the solar energy system, an investigation in which gas passages within a solar collector were combined with a solar chimney system. The results are shown and explained in this section. The findings will be shown by outlining the planned cases, including the temperatures and velocity within the system for each case, contrasting them with one another, and finally displaying the electrical energy produced for all cases. Regarding Case 1 and Case 2, they were previously displayed and explained as validation cases. So, the results will be shown starting from Case 3 until Case 7.
4.1. Results of case 3
The diameter of the solar collector and the height of the chimney were both set at 80 m when the virtual model of a solar chimney was created. The diameter of the chimney was kept at 5 m with the extension of gas pipes associated with burning it inside the solar collector, and this represents case 3. According to the default values of the design, the temperature distribution inside the collector, as shown in -a, ranged from 310 to 329 K. The velocity of the air entering the solar collector and then to the solar chimney was gradual in value until it reached the highest value at the entrance of the solar chimney, as shown in -b. It is also noted that the distribution of velocity will be a parabolic curve so that the highest velocity will be in the middle of the diameter of the chimney and was (5 m/s). This velocity is suitable for generating electricity using a wind turbine (Al-Jibouri Citation2014). Depending on the velocity that was achieved in this case, the electric power was calculated to be about 63.5 kW.
4.2. Results of case 4
The previous configuration was re-designed, but by considering the outlet of the gas channels to be inside the collector and this was represented as Case 4. The temperature distribution inside the collector, as shown in -a, fell between 307 and 339 K based on the default values of the design. As seen in -b, the air velocity as it entered the solar collector and proceeded to the solar chimney increased gradually until it reached its peak value at the chimney exit. The air moved at a velocity ranging between 0 to 8.7 m/s. The electric power was estimated to be approximately 97.98 kW based on the velocity that was attained in this case. It is worth noting that the highest speed in case 4 was at the exit of the chimney, while in case 3 it was at the base of the chimney, and this determines the location of the wind turbine installation, which was at the highest speed along the chimney.
4.3. Results of case 5
This case was designed the same as Case 4, however, increasing the distance between the associated gas burners to see its effect on the efficiency of the chimney and increasing the air velocity. In this design, only the length of the gas channel has been shortened, and the exit of the gas channel is inside the solar collector. The change in the temperature distribution of the solar chimney is noted for the design that spacing between the associated gas flares, as shown in -a, and this shows the effect of the location of the flares and their distribution inside the solar chimney collector, where the temperature ranged between 310 and 349 K. -b shows a change in the air velocity inside the solar collector, caused by a change in the location of the gas flares that go along with it. This supports the idea that air velocity that enters the solar collector and its climb to the chimney depends on the position of the related gas flares. The air moved at a velocity between 0 and 9.361 m/s. It is also noted that the highest speed was at the base of the chimney, which necessitates installing the wind turbine in this area. Based on the velocity reached in this instance, the electric power of the system was calculated to be around 116.13 kW.
4.4. Results of case 6
To study the effect of the shape of the associated gas flares on the effect of the temperature distribution inside the solar collector, the flares were re-designed in the form of a circular ring, and this case represented Case 6. With the change of the shape of the flares, it is noticed that the temperature changes inside the solar collector, as shown in -a, where the temperatures ranged between 307 and 330 K. And according to the change in temperature and the design of the circular burner, the airflow velocity inside the solar collector will change as shown in -b, where the air velocity ranged between 0 and 8.5 m/s. It is also noted that the rate of wind speed is low at the base of the chimney, as it did not exceed 6.7 m/s. It is also noted that the shape of the rate of speed inside the chimney is deformed. Based on the velocity that was achieved in this scenario, the electric power was calculated to be around 115.71 kW.
4.5. Results of case 7
To study the impact of the flares design on the solar chimney performance, another design was conducted, through the design of a gas flare in the form of two circular rings. The new design showed an effect that differs in the performance of the solar chimney from the effect of the previous design, including the effect of temperature distribution in the solar collector, as shown in -a, and the heat distribution ranged between 307 and 339.5 K. Likewise, a change in the effect of the air velocity inside the solar collector, as shown in -b, and the velocity of the airflow ranged between 0 and 10.2 m / sec. The velocity rates were highest at the base of the chimney, and this design also recorded the highest velocity compared to other designs. -c illustrates the shape of the airflow for the circular design with two rings, and it appears more streamlined in the airflow than the circular design with one ring at the entrance of the solar chimney. The estimated electric power in this scenario was 121.1 kW based on the velocity that was achieved.
Figure 9 . Temperature distribution, velocity distribution, and streamlines inside the solar chimney for Case 7. Temperature distribution; (b) Velocity distribution; (c) Streamlines of flow.

A comparison of the solar system’s electrical power values for each of the suggested design instances is shown in . The Figure makes it evident that the system’s maximum productivity was for Case 7, which was achieved by creating two rings to distribute the gas heat more evenly inside the solar collector. This is what made this system the optimum one among the other suggested configurations, with a maximum output power of 121 kW.
4.6. Thermal efficiency
Thermal efficiency is one of the most important criteria for determining the best design among the five designs presented in this study and for comparison with other designs mentioned in previously published research. . It was noted that design No. 7 achieved the highest thermal efficiency, which amounted to 0.523%, followed by design No. 4, which achieved 0.415%. To achieve the desired goal of the proposed designs, the thermal efficiency of case 7 was compared to the thermal efficiencies of the solar chimney in many previous published studies, as shown in , taking into account the difference in dimensions for the different cases. It is evident from this table that the efficiency of the proposed system (case 7) is very close to the highest efficiencies previously recorded by Alkasrawi et al. (Alkasrawi et al. Citation2021) and Guo et al. (Guo et al. Citation2021) which was 0.6018 and 0.564 respectively in comparison with the present study (case 7) which was 0.564.
Figure 11 . Different thermal efficiency of the cases at noon under the weather condition of the study location.

Table 3 . Thermal efficiencies of the solar chimney in previous published studies.
5. Conclusions
One of the unexplored potential options in the realm of utilizing renewable solar energy is the solar chimney. It has been demonstrated that using CFD is a useful method for increasing decision confidence while building and using a solar chimney. This study used numerical analysis to examine how well a solar chimney performed. The solar chimney power plant was modelled in three dimensions using ANSYS Fluent 2020R1 software. The solar chimney was tested with seven different gas channel layouts to find the most efficient one. By comparing the findings to other studies, a consensus has been reached. The simulation results demonstrated that this concept could be utilized to generate more electrical power, and the efficiency of the solar chimney was improved to allow for continuous electrical power generation around the clock, rather than just during daylight hours. The channels inside the solar collector could take several different forms. Cases 1 and 2 were created solely for validation purposes. Good agreement was achieved with the previous research with a maximum error of 2%. Maximum power outputs and air velocity were as follows: 63.5 kW and 5 m/s for case3, 97.98 kW and 8.69 m/s for case 4, 116.13 kW and 9.36 m/s for case 5, 115.71 kW and 8.48 m/s for case 6, and 121.1 kW and 10.21 m/s for case 7. According to the findings, a two-ring burner with a circular layout (Case 7) produced the most efficient output of electric power (about 121.1 kW) from the system.. Also, it was noted that design No. 7 achieved the highest thermal efficiency, which amounted to 0.523%, followed by design No. 4, which achieved 0.415%. Through the default design of this technology, it was shown that it is possible to combine these two technologies to produce electric power with capacities that depend on the size of the station, and thus it is a high-quality investment in the subject of investment in the associated petroleum gas, which is depleted in a way that affects the economy and the environment. Also, it is necessary to study the economic feasibility, financial cost, and environmental impact of new designs of solar chimneys.
Disclosure statement
No potential conflict of interest was reported by the author(s).
References
- Abbas, E. F., and S. A. Aziz. 2022. “The Impact of Air Gap Width on the Free Thermal Load in the Trombe Wall Contains a Phase Change Material.” Al-Kitab Journal for Pure Sciences 2 (2): 264–275. https://doi.org/10.32441/kjps.02.02.p18.
- Ahmed, O. K. 2018. “Assessment of the Performance for a New Design of Storage Solar Collector.” International Journal of Renewable Energy Research 8 (1): 250–257.
- Ahmed, O. K. 2018. “A Numerical and Experimental Investigation for a Triangular Storage Collector.” Solar Energy 171 (June): 884–892. https://doi.org/10.1016/j.solener.2018.06.097.
- Ahmed, O. K., S. Algburi, Z. H. Ali, A. K. Ahmed, and H. N. Shubat. 2022a. “Hybrid Solar Chimneys: A Comprehensive Review.” Energy Reports 8: 438–460. https://doi.org/10.1016/j.egyr.2021.12.007.
- Ahmed, O. K., A. A. Hassan, E. F. Abbas, and R. W. Doud. 2022b. “Numerical and Experimental Assessment of PV / Solar Chimney.” NTU Journal of Renewable Energy 2 (1).
- Ahmed, O. K., and A. S. Hussein. 2018. “New Design of Solar Chimney (Case Study).” Case Studies in Thermal Engineering 11 (December 2017): 105–112. https://doi.org/10.1016/j.csite.2017.12.008.
- Akbarzadeh, A., P. Johnson, and R. Singh. 2009. “Examining Potential Benefits of Combining a Chimney with a Salinity Gradient Solar Pond for Production of Power in Salt Affected Areas.” Solar Energy 83 (8): 1345–1359. https://doi.org/10.1016/j.solener.2009.02.010.
- Al-Jibouri, D. O. K. A. 2014. “Feasibility of Using Wind Energy for Irrigation in Iraq.” International Journal of Mechanical Engineering and Technology 5 (5): 62–72.
- Al-Kayiem, H. H., M. A. Aurybi, S. I. U. Gilani, A. A. Ismaeel, and S. T. Mohammad. 2019. “Performance Evaluation of Hybrid Solar Chimney for Uninterrupted Power Generation.” Energy 166: 490–505. https://doi.org/10.1016/j.energy.2018.10.115.
- Alkasrawi, M., E. Abdelsalam, H. Alnawafah, F. Almomani, M. Tawalbeh, and A. Mousa. 2021. “Integration of Solar Chimney Power Plant with Photovoltaic for co-Cooling, Power Production, and Water Desalination.” Processes 9 (12): 2155–2117. https://doi.org/10.3390/pr9122155.
- ANSYS, I. 2013. ANSYS Fluent Tutorial Guide Releas 15 (1. Canonsburg): 130–140.
- Aweid, R. S., O. K. Ahmed, and S. Algburi. 2022. “Performance of Floating Photovoltaic/Thermal System: Experimental Assessment.” International Journal of Energy Research (April): 24229–24242. https://doi.org/10.1002/er.8729.
- Bouabidi, A., H. Nasraoui, A. Ayadi, Z. Driss, and M. S. Abid. 2019. “Numerical Analysis of Chimney Diameter Effect on the Fluid Flow and the Heat Transfer Characteristics Within the Solar Tower.” Energy Sources, Part A: Recovery, Utilization, and Environmental Effects 41 (20): 2494–2506. https://doi.org/10.1080/15567036.2019.1568631.
- BP. 2022. “BP Statistical Review of World Energy 2022, (71st ed.),” [Online]. Available: https://www.bp.com/content/dam/bp/business-sites/en/global/corporate/pdfs/energy-economics/statistical-review/bp-stats-review-2022-full-report.pdf.
- Cao, F., H. Li, Q. Ma, and L. Zhao. 2014. “Design and Simulation of a Geothermal-Solar Combined Chimney Power Plant.” Energy Conversion and Management 84: 186–195. https://doi.org/10.1016/j.enconman.2014.04.015.
- Cuce, E., et al. 2022. “Solar Chimney Power Plants: A Review of the Concepts, Designs and Performances.” Sustainability 14 (3), https://doi.org/10.3390/su14031450.
- Das, P., and V. P. Chandramohan. 2018. “CFD Analysis on Flow and Performance Parameters Estimation of Solar Updraft Tower (SUT) Plant Varying its Geometrical Configurations.” Energy Sources, Part A: Recovery, Utilization, and Environmental Effects 40 (12): 1532–1546. https://doi.org/10.1080/15567036.2018.1477881.
- Djaouida, B., Z. Aouachria, A. H. Benmachiche, and S. Ali. 2020. “Controlling Power Output of Solar Chimney Power Plant According to Demand.” International Journal of Ambient Energy 41 (13): 1467–1481. https://doi.org/10.1080/01430750.2018.1517677.
- Esmaili, M. M., S. H. Fallah, M. Izanlu, and M. S. Valipour. 2022. “Investigation on the Performance of a Solar Chimney-Flare gas Hybrid System.” Sustainable Energy Technologies and Assessments 52 (PC): 102279. https://doi.org/10.1016/j.seta.2022.102279.
- Fathi, N., et al. 2018. “Efficiency Enhancement of Solar Chimney Power Plant by use of Waste Heat from Nuclear Power Plant.” Journal of Cleaner Production 180: 407–416. https://doi.org/10.1016/j.jclepro.2018.01.132.
- Ganguli, A. A., and S. Deshpande. 2020. “Three Dimensional CFD Studies of a Solar Chimney: Effect of Geometrical Parameters and Diurnal Variations on Power Generated.” Frontiers in Chemical Engineering 2 (April): 1–15. https://doi.org/10.3389/fceng.2020.00002.
- Gholamalizadeh, E., and J. D. Chung. 2017. “Analysis of Fluid Flow and Heat Transfer on a Solar Updraft Tower Power Plant Coupled with a Wind Turbine Using Computational Fluid Dynamics.” Applied Thermal Engineering 126: 548–558. https://doi.org/10.1016/j.applthermaleng.2017.07.192.
- Gomaa, M. R., M. Ahmed, and H. Rezk. 2022. “Temperature Distribution Modeling of PV and Cooling Water PV/T Collectors Through Thin and Thick Cooling Cross-Fined Channel box.” Energy Reports 8: 1144–1153. https://doi.org/10.1016/j.egyr.2021.11.061.
- Guo, P., T. Li, B. Xu, X. Xu, and J. Li. 2019. “Questions and Current Understanding About Solar Chimney Power Plant: A Review.” Energy Conversion and Management 182 (October 2018): 21–33. https://doi.org/10.1016/j.enconman.2018.12.063.
- Guo, P., Y. Zhou, D. Zhang, B. Xu, and J. Li. 2021. “Numerical Investigation and Multi-Objective Thermo-Economic Optimization of a Solar Chimney Power Plant.” International Journal of Energy Research 45 (7): 10317–10331. https://doi.org/10.1002/er.6521.
- Habibollahzade, A., E. Houshfar, P. Ahmadi, and A. Behzadi. 2018. “Exergoeconomic Assessment and Multi-Objective Optimization of a Solar Chimney Integrated with Waste-to-Energy.” Solar Energy 176 (April): 30–41. https://doi.org/10.1016/j.solener.2018.10.016.
- Habibollahzade, A., E. Houshfar, M. Ashjaee, and K. Ekradi. 2021. “Continuous Power Generation Through a Novel Solar/Geothermal Chimney System: Technical/Cost Analyses and Multi-Objective Particle Swarm Optimization.” Journal of Cleaner Production 283: 124666. https://doi.org/10.1016/j.jclepro.2020.124666.
- Hassan, A., M. Ali, and A. Waqas. 2018. “Numerical Investigation on Performance of Solar Chimney Power Plant by Varying Collector Slope and Chimney Diverging Angle.” Energy 142: 411–425. https://doi.org/10.1016/j.energy.2017.10.047.
- hua Guo, P., J. yin Li, and Y. Wang. 2014. “Numerical Simulations of Solar Chimney Power Plant with Radiation Model.” Renewable Energy 62 (January 2014): 24–30. https://doi.org/10.1016/j.renene.2013.06.039.
- Hussein, A. S., and O. K. Ahmed. 2018. “Assessment of the Performance for a Hybrid PV / Solar Chimney.” International Journal of Engineering & Technology 7 (4.37): 114–120. https://doi.org/10.14419/ijet.v7i4.37.24085
- Ibrahim, A. K., S. Algburi, and O. K. Ahmed. 2023. “Enhancement of the Performance of the PV Trombe Wall: A Short Review.” Cleaner Engineering and Technology 14 (June): 100652. https://doi.org/10.1016/j.clet.2023.100652.
- Ismail, A., A.-A. El-Marhoumy, A. Hamed, and A. T. A. Eldein Hussin. 2019. “Numerical Modeling for a Solar Chimney.” Journal of Al-Azhar University Engineering Sector 14 (50): 87–98. https://doi.org/10.21608/auej.2019.28512.
- Kiwan, S., M. Al-Nimr, and I. Salim. 2020. “A Hybrid Solar Chimney/Photovoltaic Thermal System for Direct Electric Power Production and Water Distillation.” Sustainable Energy Technologies and Assessments 38 (November 2019): 100680. https://doi.org/10.1016/j.seta.2020.100680.
- Lipnicki, Z., M. Gortych, A. Staszczuk, T. Kuczyński, and P. Grabas. 2019. “Analytical and Experimental Investigation of the Solar Chimney System.” Energies 12 (11), https://doi.org/10.3390/en12112060.
- Mahmood Ibrahim, A., R. R. Ibraheem, and R. Bakr Weli. 2023. “Energy Saving in Batteries Using the Photovoltaic System.” Al-Kitab Journal for Pure Sciences 4 (1): 78–94. https://doi.org/10.32441/kjps.04.01.p7.
- H. K. V., and W. Malalasekera. 2007. Introduction to Computational Fluid Dynamics. 2nd ed. Glasgow: Harlow.
- Mullett, L. B. 1987. “The Solar Chimney—Overall Efficiency, Design and Performance.” International Journal of Ambient Energy 8 (1): 35–40. https://doi.org/10.1080/01430750.1987.9675512
- Pratap Singh, A., A. Kumar Akshayveer, and O. P. Singh. 2020. “Performance Enhancement Strategies of a Hybrid Solar Chimney Power Plant Integrated with Photovoltaic Panel.” Energy Conversion and Management 218 (May): 113020. https://doi.org/10.1016/j.enconman.2020.113020.
- Rabehi, R., A. Chaker, Z. Aouachria, and M. Tingzhen. 2017. “CFD Analysis on the Performance of a Solar Chimney Power Plant System: Case Study in Algeria.” International Journal of Green Energy 14 (12): 971–982. https://doi.org/10.1080/15435075.2017.1339043.
- Setareh, M. 2021. “Comprehensive Mathematical Study on Solar Chimney Powerplant.” Renewable Energy 175: 470–485. https://doi.org/10.1016/j.renene.2021.05.017.
- Torabi, M. R., et al. 2021. “Investigation the Performance of Solar Chimney Power Plant for Improving the Efficiency and Increasing the Outlet Power of Turbines Using Computational Fluid Dynamics.” Energy Reports 7: 4555–4565. https://doi.org/10.1016/j.egyr.2021.07.044.
- US Energy Information Adminsitration (EIA). 2017. “Country Analysis Executive Summary: Iraq.” U.S. Energy Information Administration 2022 (January): 1–8.
- Zou, Z., and S. He. 2015. “Modeling and Characteristics Analysis of Hybrid Cooling-Tower-Solar-Chimney System.” Energy Conversion and Management 95: 59–68. https://doi.org/10.1016/j.enconman.2015.01.085.
- Zuo, L., et al. 2021. “Economic Performance Evaluation of the Wind Supercharging Solar Chimney Power Plant Combining Desalination and Waste Heat After Parameter Optimization.” Energy 227: 120496. https://doi.org/10.1016/j.energy.2021.120496.