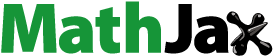
ABSTRACT
Smash-ridge tillage is a novel cultivation technique that significantly influences the quality of arable land and crop yield. In this study, we employed high-throughput 16S rRNA sequencing and Biolog-ECO methods to systematically investigate the impact of smash-ridge tillage on soil microbial community structure and functional diversity. The results demonstrate that both ST30 and ST50 treatments significantly enhance the average plant height, average plant diameter, average fresh root weight, stem fresh weight, and leaf area of tobacco plants, with the ST50 treatment exhibiting superior performance. Furthermore, both ST30 and ST50 treatments exhibit significantly higher soil enzyme activity and microbial community diversity compared to the CK treatment. They also improve the soil microbial utilization of carbon sources. Additionally, the ST50-treated soil samples demonstrate 15 microbial functional pathways that exceed those of the CK and ST30 treatments. In conclusion, the Smash-ridge tillage treatment at a depth of 50 cm yields more favorable results. This study provides a theoretical foundation for enhancing soil quality in Smash-ridge tillage by elucidating the mechanisms through which it impacts soil microbial ecology.
1. Introduction
In China, tobacco is a significant cash crop that provides substantial economic benefits, prompting farmers to engage in continuous tobacco cultivation on the same land to maximize income. Due to the labor-intensive and technologically demanding nature of tobacco cultivation, farmers often adhere to fixed plots in order to minimize costs, improve efficiency, and exhibit reluctance to change locations or diversify cropsCitation1. Continuous tobacco cropping can have adverse effects on soil quality, microbial communities, and the yield and quality of tobacco. Continuous cropping leads to soil acidification, resulting in increased phenolic acid content and decreased bacterial diversity, which can disrupt the structure of bacterial communitiesCitation2. Therefore, the application of modern and improved scientific farming methods is particularly important.
Smash-ridge tillage is a deep tillage technique that combines the advantages of deep loosening, deep tillage, rotary tillage, and vertical tillageCitation3, which can break compacted plowshares and loosen the soil layer without disturbing it and producing new hard plows. Smash-ridging cultivation is a novel farming method that significantly enhances soil quality, water and nutrient retention, root development, and crop yieldCitation4. This method increases soil porosity, organic matter, and available nutrients while reducing bulk density and pHCitation5. Furthermore, studies show that this cultivation method can improve soil structure, water retention, nutrient availability, root growth, forage yield, and qualityCitation6. It has been applied to various crops across different provinces in China, Studies have shown that the fine ridge tillage mode has stronger beneficial effects on soil quality, water storage capacity, crop yield, root vitality, soil microbial biomass and microbial diversity in farmlandCitation7,Citation8. In recent years, the flour ridge tillage technique has achieved great success in agricultural production. However, the effects of silty tillage on soil microbial communities and soil ecosystem functions in cultivated land are currently unknown.
In this study, we employed high-throughput 16S rRNA sequencing and Biolog-ECO methods to systematically investigate the impact of smash-ridge tillage on soil microbial community structure and functional diversity. The objectives of this study were as follows: (1) to assess the variations in soil enzyme activity, microbial diversity, and community structure in cultivated land under different farming methods; (2) to analyze the functional diversity and groups of soil microbial communities under various tillage methods; and (3) to examine how smash-ridge tillage influences the relationship between soil microbial structure, enzyme activity, and microbial functional diversity. The anticipated outcomes of this research aim to provide a theoretical foundation and technical support for addressing the challenges associated with continuous tobacco cropping and enhancing tobacco production.
2 Methods
2.1 Soil sampling
The study site was located in Zhaohua County, Guangyuan City, Sichuan Province (32° 0’ 54.92” N, 105° 26’ 39.09” E). The area of flue-cured tobacco in Zhaohua County was 1946.6 HM2, making it one of the important producing areas in northern Sichuan Province. The region has a humid subtropical climate, with uneven rainfall distribution resulting in earlier drought and later flooding periods. The average annual temperature, rainfall, and sunshine length are 15.4°C, 1003.9 mm, and 1328.3 hours, respectively. The soil type is calcareous purple soil, and the basic physical and chemical indexes of the sample soil are shown in .
Table 1. Basic chemical character of the sample soil.
The tested variety was “Zhongchuan 208.” Three treatments were established, namely, traditional cultivation with a depth of 20 cm (CK), powdered ridge cultivation with a depth of 30 cm (ST1), and powdered ridge cultivation with a depth of 50 cm (ST2). In this experiment, a suspended powdered ridge machine was used to fully loosen and vertically grind the soil, followed by compaction of the powdered ridges. The control group used traditional cultivation with tractor plowing and harrowing. The land was prepared in March 2021, tobacco seedlings were transplanted on April 5, and the harvest took place on September 27.
Soil samples were collected on September 28. The soil samples were taken using a five-point sampling method at a depth of 20 cm in the plowed layer, with five replicate samples for each soil. The soil samples were placed in sterile bags, sieved with a 2 mm sieve after removing stones, and stored at 4°C for soil enzyme activity and Biology-ECO analysis. Fresh soil samples of 50 g were sieved with a 1 mm sieve and stored at −80°C in an ultra-low temperature freezer for the determination of soil microbial community structure.
2.2 Measurement of agronomic traits of tobacco plants
Measurement of plant growth indicators was conducted on selected 15 tobacco plants from each continuous cropping year plot after 110 days of plant growth.
Plant height: The distance from the soil surface to the topmost point of the stem.
Leaf area: A leaf was selected at the waist level, and its length and width were measured. The length was measured from the leaf base to the leaf tip, and the width was measured at the widest point. The leaf area was calculated using a conversion coefficient of 0.63.
Leaf area = Length × Width × Conversion coefficient
Stem circumference: The lowermost point of the aboveground section of the tobacco plant was established as the initial reference point. Using a flexible measuring tape positioned at a point located one-third of the plant’s overall height from the starting position, the stem circumference of the tobacco plant was measured.
Effective leaf number: During the actual cultivation process, tobacco plants underwent leaf removal during the seedling stage and topping treatment during the field period. The lower foot leaves were also removed. The remaining number of leaves was considered the effective leaf number.
Fresh root weight: After the tobacco roots were dug out from the soil and washed, the surface moisture was absorbed with absorbent paper or filter paper, and the fresh root weight was measured using an electronic balance.
Fresh stem weight: After removing the underground part and leaves of the tobacco plant, the fresh weight of the stem per plant was measured using an electronic balance.
2.3 Determination of soil enzyme activity
Urease activity: For each sample, 2–5 g of air-dried soil was weighed into a 50 mL centrifuge tube. Then, 1 mL of toluene was added to ensure the complete wetting of the soil sample. After 15 minutes, 10 mL of 10% urea solution and 20 mL of citric acid buffer solution (pH = 6.7) were added, followed by thorough shaking. The conical flask was placed in a constant temperature chamber at 37°C and incubated for 24 hours. After incubation, the solution was diluted to the mark with water at 38°C, thoroughly shaken, centrifuged, and filtered. A portion (1–3 mL) of the filtrate was drawn into a 50 mL colorimetric tube, diluted to 20 mL with distilled water, vigorously shaken, followed by the addition of 4 mL of phenol-sodium solution and thorough mixing. Then, 3 mL of sodium hypochlorite was added, shaken well, and left to stand for 20 minutes. The solution was further diluted to the mark with water, resulting in an indophenol blue color (maintained stable for up to 1 hour). Colorimetric measurement was performed at 578 nmCitation9.
m1: the milligrams of NH3-N derived from the sample absorbance value obtained from the standard curve; m2: the milligrams of NH3-N obtained from the no soil control absorbance value from the standard curve; m3: the milligrams of NH3-N obtained from the no substrate control absorbance value from the standard curve; V: the volume of the color solution; N: the ratio of the leaching liquid volume to the filtrate volume; M: the weight of dried soil.
Nitrite reductase activity: Taking 1 g of fresh soil sample in a 100 ml plastic bottle, 20 mg of CaCO3 and 1 ml of KNO3 solution were added. After thorough mixing, 1 ml of glucose solution was added, while in another variant, an equal amount of distilled water was added as a substitute for the substrate. The bottle was securely capped, lightly shaken, and placed in a constant temperature incubator at 30°C for 24 hours. Simultaneously, a reagent blank control was set up. After incubation, 50 ml of deionized water and 1 ml of aluminum potassium sulfate solution were added, followed by a 20-minute settling period. After thorough mixing, the solution was filtered. Subsequently, 20 ml of the filtrate was transferred to a porcelain evaporating dish (using a conical flask) and evaporated in a water bath. Then, 2 ml of phenol-sulfuric acid solution was added for dissolution, followed by a 10-minute treatment. Afterward, 15 ml of deionized water was added, and the solution was adjusted to a yellow using 10% NaOH. Finally, the solution was transferred to a 50 ml volumetric flask, brought to volume, and colorimetrically measured at 400 ~ 500 nmCitation9.
C1: The absorbance value of the no-soil blank, representing the calculated NO3-N content from the standard curve; C2: The absorbance value of the sample, representing the calculated NO3-N content from the standard curve; C3: The absorbance value of the soil without substrate (potassium nitrate), representing the calculated NO3-N content from the standard curve (optional at this stage); V: Volume of the test solution (50 ml); f: Fractional factor (50 ml/20 ml);dwt: Dry weight of the soil.
Alkaline phosphatase activity: Take an appropriate amount of air-dried soil and place it into a 200 ml Erlenmeyer flask. Treat with 5 drops of toluene for 15 minutes, then add 20 ml of 0.5% sodium phenylphosphonate. Place the reaction mixture in a 37°C constant temperature incubator and incubate for 24 hours. After incubation, filter the mixture through a dense filter paper using 100 ml of 0.3% aluminum sulfate solution. No-soil control: Exclude soil samples and follow the same procedures as the sample experiments. This control is established to verify the purity of reagents throughout the experiment. No-substrate control: For each soil sample, replace the substrate with an equal volume of water, following the same procedures as the sample experiments. Colorimetric procedure: Transfer 3 ml of the filtrate to a 50 ml volumetric flask and follow the coloration method described for constructing the standard curve. When using borate buffer solution, the color appears blue, and colorimetric measurement is performed at 510 nm using a spectrophotometer. Construction of the standard curve: Take 0, 1, 3, 5, 7, 9, 11, and 13 ml of the phenol working solution (b), place them in 50 ml volumetric flasks, add 5 ml of buffer solution and 4 drops of chlorodibromobenzene quinone reagent. After coloration, dilute to the mark, and perform colorimetric measurement after 30 minutes. Construct a standard curve with optical density on the y-axis and concentration on the x-axisCitation9.
a1: the milligrams of phenol derived from the sample absorbance value obtained from the standard curve; a2: the milligrams of phenol obtained from the no-soil control absorbance value from the standard curve; a3: the milligrams of phenol obtained from the no-substrate control absorbance value from the standard curve; V: the volume of the color solution; n: the ratio of the leaching liquid volume to the filtrate volume; m: the weight of dried soil.
Aryl sulfatase activity: Place 1.00 g of fresh soil sample (<2 mm) into a 50 mL Erlenmeyer flask, add 0.2 mL of toluene, 4 mL of buffer solution, and 1 mL of potassium picrate solution. To eliminate the color influence of soil leachate, a control should be performed by adding 0.2 mL of toluene and 4 mL of buffer solution to 1.00 g of soil sample. Gently shake to mix, close with a stopper, and incubate at 37°C for 1 hour. After incubation, remove the lid, add 1 mL of CaCl2 solution and 4 mL of NaOH solution. For the control, add 1 mL of sodium picrate solution and shake briefly for a few seconds, then immediately filter through filter paper.
Simultaneously, prepare the solutions for constructing the working curve. Dilute the prepared standard solution by 100 times with water, then draw 1 mL, 2 mL, 3 mL, 4 mL, and 5 mL of the diluted standard solution into separate 50 mL Erlenmeyer flasks (containing 0 mg, 0.01 mg, 0.02 mg, 0.03 mg, 0.04 mg, and 0.05 mg of picrate). Adjust each to 5 mL with water, add 1 mL of CaCl2 and 4 mL of NaOH solution, shake briefly, and then filter through filter paper.
Perform colorimetric analysis under the same conditions. Use a spectrophotometer to measure the absorbance of the solution at 400 nm − 420 nmCitation9.
w: The amount of picrate produced per unit of time; m1: The mass of picrate in the test solution; m2: The mass of the sample; K: The moisture coefficient.
β-glucosidase activity: Place 1 g of fresh soil sample (<2 mm) in a 100 ml Erlenmeyer flask, add toluene, allow ventilation in a fume hood for 10 minutes, then add 4 ml of pH-adjusted MUB solution and 1 mm of PNPG solution. Close the flask tightly, thoroughly mix the contents, and incubate at 37°C for 1 hour. Add 1 ml of CaCl2 solution and 4 ml of pH-adjusted Tris buffer, shake well, rapidly filter through fast filter paper, and perform colorimetric measurement of the filtrate at 400 nm. For the blank control, after incubation, before adding CaCl2 and Tris buffer, introduce 1 ml of buffer solution (substituting for 1 ml of substrate solution). Each sample requires a blank control and three replicates. Standard curve: draw 0, 1, 2, 3, 4, 5, and 6 ml into a 100 ml volumetric flask, dilute to 5 ml with deionized water, then add 1 ml of calcium chloride solution and 4 ml of Tris solution. Thoroughly mix, adjust to 50 ml, filter, and perform colorimetric measurement at 400 nmCitation9.
C: the content of picric acid in the sample; V: the volume of the soil solution; dwt: the dry soil mass.
Determination of soil microbial functional diversity
The microbial carbon source utilization characteristics in soil were determined using the Biolog method. A total of 5.0 g of soil sample was weighed and added to a triangular flask containing 45 mL of 0.85% sterile physiological saline. The flask was shaken at 150 rpm for 30 minutes at 25°C, followed by a 1-minute ice bath and 30 minutes of settling to obtain a microbial suspension of the soil sample. The soil suspension underwent a substantial 1000-fold dilution. Employing a micropipette within aseptic conditions provided by a laminar flow cabinet, the meticulously prepared soil suspension was systematically introduced into the individual wells of the Biolog microplate, each accommodating precisely 150 μL of the suspension. After securely sealing the plates, they were meticulously positioned within a 25°C incubation chamber, ensuring an uninterrupted period of 7 days for incubation under darkness. Periodic measurements were recorded at a wavelength of 590 nm, conducted at 24-hour intervals. The average well color development (AWCD) of microbial metabolic activity was calculated using the formula:
Where Ci is the absorbance value of each well at 590 nm, R is the absorbance value of the control well at 590 nm, and n represents the number of carbon sources in the EcoPlate.
The ability of soil microbes to utilize each category of carbon sources was determined by calculating the AWCD values for each category of carbon sources in the EcoPlate, using the formula:
Where Cm is the absorbance value of each category of carbon source at 590 nm, R is the absorbance value of the control well at 590 nm, and n represents the number of that particular category of carbon sources in the EcoPlate.
2.4 Methods for studying soil microbial community structure and functional composition
DNA extraction and PCR amplification
Genomic DNA of soil samples was extracted using the CTAB method, and the purity and concentration of DNA were detected by 1% agarose gel electrophoresis. A suitable amount of sample was taken in a centrifuge tube and diluted with sterile water to a concentration of 1 ng·µL-1. For bacterial sequencing, the V3 and V4 variable regions were amplified by PCR using primers 341F (5’-CCTAYGGGRBGCASCAG-3’) and 806 R (5’-GGACTACNNGGGTATCTAAT-3’). For fungal community sequencing, the ITS3 and ITS4 regions were amplified by PCR using primers 2024F (5’-GCATCGATGAAGAACGCAGC-3’) and 2409 R (5’-TCCTCCGCTTATTGATATGC-3’). All PCR products were analyzed by 2% agarose gel electrophoresis.
Illumina novaseq sequencing
PCR products with equal concentrations were thoroughly mixed. The mixed products were then purified using a 2% agarose gel with 1×TAE buffer. The target bands were excised and recovered using the Qifollowagen Gel Extraction Kit. The prepared libraries were constructed using the Illumina TruSeq® DNA PCR-Free Sample Preparation Kit (Illumina, USA). The quantification and quality control of the libraries were performed using Qubit and library detection. After passing the quality control, the libraries were sequenced using the NovaSeq 6000 PE250 platform.
Bioinformatics Analysis
The analysis process mainly followed the “Atacama soil microbiome tutorial” in the QIIME2 documentation. The raw sequence fastq files were imported into a format suitable for subsequent processing in QIIME2 using the qiime tools import plugin. Subsequently, the QIIME2 dada2 plugin was utilized for quality control, trimming, denoising, merging, and removing chimeras, resulting in the final feature sequence table. The QIIME2 feature-classifier plugin was employed to align the representative sequences of the Amplicon Sequence Variants (ASVs) against the pre-trained 13_8 version of the GREENGENES database with 99% similarity (trimmed to the V3-V4 region based on the 341F/806 R primers), obtaining the taxonomic classification information of the speciesCitation10. Next, the QIIME2 feature-table plugin was used to remove all contaminating mitochondrial and chloroplast sequences. The LEfSe method was then applied to identify differentially abundant bacteria among groups and samples. Subsequently, the QIIME2 core-diversity plugin was employed to calculate the diversity matrix, including alpha diversity indices at the feature sequence level (Chao1, Ace, Shannon, and Simpson indices) to assess the biodiversity within samples. Beta diversity indices of samples were visualized using NMDS plots. The FAPROTAX software was used to predict the potential functional composition of the microbial community.
2.5 Data analysis
Statistical significance tests were conducted using the R girmess package, multcompView package, and agricolae package. PCA analysis was performed using the R FactoMineR package. Random forest regression analysis was conducted using the R RandomForest package. Data visualization was carried out using the R ggplot2 package.
3. Results
3.1 Effects of smash-ridge tillage on agronomic traits and soil enzyme activity of tobacco plants
(1) Performance of agronomic traits of tobacco plants with different smash-ridge tillage treatment
(2) Performance of soil enzyme activity of tobacco plants with different smash-ridge tillage treatment
depicts the performance of six agronomic traits throughout the growth process of tobacco plants under various cultivation conditions. Among the samples, the treatment with a smash-ridge tillage depth of 30 cm (ST30) exhibited a significantly greater leaf area (1080.87 cm2) compared to the control group (CK), whereas the other five indicators showed no significant differences in comparison to the control group (CK). Conversely, the treatment with a smash-ridge tillage depth of 50 cm (ST50) demonstrated significantly higher average plant height (119.33 cm), average stem circumference (10.25 cm), average fresh root weight (93.67 g), stem fresh weight (93.67 g), and leaf area (1276.61 cm2) compared to the control group (CK) (p < 0.05).
Figure 1. Performance of agronomic traits in tobacco plants under different cultivation methods. (a) average plant height of tobacco plants(b) average stem circumference of tobacco plants(c) average leaf area of tobacco plants(d) average number of leaves on tobacco plants(e) average fresh root weight of tobacco plants(f) average fresh stem weight of tobacco plants.

presents the impact of different cultivation methods on soil enzyme activity. The results indicate that soil samples obtained from smash-ridge tillage cultivation exhibited significantly higher activities of urease, nitrite reductase, alkaline phosphatase, and aryl sulfatase enzymes compared to soil samples from traditional plow-tilling cultivation (CK). Moreover, when comparing different smash-ridge tillage depths, the soil samples with a smash-ridge tillage depth of 50 cm displayed significantly greater activities of these four enzymes in comparison to soil samples with a smash-ridge tillage depth of 30 cm. Only β-glucosidase activity exhibited higher levels in the CK samples compared to the samples from the smash-ridge tillage at a depth of 30 cm (p < 0.05).
Table 2. Influence of different cultivation methods on soil enzyme activity.
3.2 Impact of smash-ridge tillage on soil microbial community composition and function in tobacco plantation
(1) Performance of soil microbial community composition with different smash-ridge tillage treatment
In this study, the bacterial community composition of all soil samples was examined using 16S rDNA sequencing technology, resulting in the identification of 151 operational taxonomic units (OTUs) at the phylum level. As shown in , the dominant bacterial phyla in soil samples from different cultivation treatments were relatively consistent, including Proteobacteria, Acidobacteria, Bacteroidetes, Actinobacteria, Gemmatimonadetes, Verrucomicrobia, Chloroflexi, Nitrospirae, Firmicutes and Cyanobacteria with cumulative relative abundances exceeding 95%. Moreover, the relative abundance of Proteobacteria was significantly higher in the soil samples from smash-ridge tillage cultivation (30 cm and 50 cm) compared to the traditional cultivation method, while the relative abundance of Acidobacteria showed a significant decrease. illustrates the distribution of unique bacterial OTUs in soil samples from different cultivation methods. As depicted in the figure, both the CK sample and the 30 cm smash-ridge tillage soil sample had one unique bacterial OTU, while the 50 cm smash-ridge tillage soil sample had six unique OTUs.
Figure 2. Bacterial community composition (phylum level) of soil samples under different cultivation conditions (a) bacterial phylum composition (b) distribution of specific OTUs in different treatments.

Based on the annotation of OTUs, species richness and diversity indices of bacterial communities in different soil samples were further analyzed. As shown in , the species richness indices (Ace index and Chao1 index) of soil samples from both f smash-ridge tillage heights (30 cm/50 cm) were significantly higher than those of the control soil samples. However, there was no significant difference in species richness indices between soil samples with different smash-ridge tillage depths. Similarly, the Shannon Index and Simpson Index of bacterial community structures in smash-ridge tillage soil samples at different depths were significantly higher than those in the control samples. However, there was no significant difference observed between the 30 cm and 50 cm depths.
Table 3. α biodiversity indices of soil bacterial communities under different cultivation conditions.
β biodiversity is often used to describe the compositional differences of species among different habitats. In this study, the differences in soil bacterial community structure under different cultivation methods were analyzed using NMDS (), with a stress value of 0.1043 based on Bray-Curtis dissimilarity. The figure clearly illustrates that the soil samples from different cultivation treatments were categorized into three major groups based on their microbial community characteristics.
Figure 3. β biodiversity of soil bacterial communities under different cultivation conditions (NMDS).

(2) Performance of carbon source utilization characteristics of bacterial communities with different smash-ridge tillage treatment
Figure 4. Carbon source utilization characteristics of bacterial communities in soil samples under different tillage conditions (a)percentage of explained variance in principal components(b) contribution of different carbon sources to the first two principal components(c) relative position of soil samples in the first two principal components.

An analysis was conducted to examine the carbon source utilization of soil microbial communities across different soil layers, resulting in the identification of six principal components. The first two principal components exhibited variance contribution rates of 85.1% and 7.1% respectively (). Given that the cumulative variance contribution rate of the first two principal components exceeds 90%, subsequent analysis focused on these two components.
presents the contribution analysis of the six major carbon sources to principal components 1 and 2. The results indicate that all carbon sources are situated in the first and fourth quadrants, implying a positive influence on principal component 1. The ranking of principal component contribution degrees, in descending order, are as follows: C2 > C5 > C3 > C1 > C6 > C4.(C1: carbonhydrate; C2: carboxylic acid; C3: amine;C4: amino acid;C5: phenolic acid;C6: polymer)
(3) Performance of microbial functions prediction with different smash-ridge tillage treatment
Significant differences in carbon source utilization were observed among soil samples subjected to various tillage practices. illustrates the relative positions of different tillage soil samples within the two-dimensional map defined by the first two principal components. The CK soil samples are primarily distributed in the second and third quadrants, exhibiting proximity to the distribution of fine ridge tillage samples at a depth of 30 cm. Furthermore, there is an overlap with the 95% confidence ellipse, suggesting similarities in carbon source utilization between the two sample groups. In contrast, the fine ridge tillage samples at a depth of 50 cm are predominantly located in the first and fourth quadrants, with no intersection with the 95% confidence ellipse of the preceding two treatment soil samples. This observation implies that their carbon source utilization differs from the aforementioned groups.
Figure 5. Prediction of FAPROTAX microbial functions in soil samples under different tillage conditions.

The FAPROTAX database utilizes published scientific literature on relevant bacterial strains to annotate and map OTUs (Operational Taxonomic Units) to established metabolic or other ecologically relevant functions. It is particularly suitable for functional annotation and prediction of biogeochemical cycling processes in environmental samples. In this study, a total of 688 OTUs were identified at the species level and were used for predicting the main functions of soil microorganisms using the FAPROTAX database. displays the top 20 ranked functional pathways based on their relative abundances.
The analysis reveals that among the top twenty functional pathways, twelve are closely associated with nitrogen utilization and the biogeochemical cycling of nitrogen. Furthermore, a comparison of functional pathways in different tillage samples indicates that the expression levels of 15 pathways in the 50 cm fine ridge soil sample are significantly higher than those in the control sample.
This study employed random forest regression analysis to identify the top 10 OTUs at the genus level that exert the most significant influence on tobacco plant leaf area (). Among these, the three most influential OTUs were Granulicella, Ilumatobacter, and Modestobacter, with importance values of 2.37, 2.20, and 2.12, respectively. Following closely were OTUs ranked 4th to 7th: Caldilinea, Bacillus, Nitrobacteria, Kaistobacter and Sphingomonas, each exhibiting importance values exceeding 2.
4. Discussion
Numerous research results have demonstrated that the composition and activity of soil microbial communities are influenced by agricultural tillage practicesCitation11. Relevant studies have found that fine ridge tillage provides favorable conditions for the survival of deep soil microorganisms, leading to a significant increase in the total bacterial count in the 0-40 cm soil layer compared to conventional tillage. Fine ridge tillage effectively enhances the abundance of soil microorganisms and increases the quantities of ammonia-oxidizing bacteria, nitrogen-fixing bacteria, inorganic phosphate bacteria, and potassium bacteria in the soil compared to rotary tillage and deep plowing treatmentsCitation12. The results of this study indicate that fine ridge tillage significantly improves both the abundance and biodiversity of bacterial communities compared to traditional rotary tillage, which is consistent with previous research findings. The reason behind this result may be attributed to the ability of fine ridge tillage to effectively reduce soil compaction and promote the formation of soil macroaggregatesCitation13,Citation14. The dynamic changes in soil aggregate structure feedback on the control of microbial activity and the differentiation of soil organic matter composition, thereby establishing a coupling effect between soil organic matter composition-soil aggregate structure-microbial community structure dynamicsCitation15, ultimately altering bacterial community structure and diversity.
In this study, following various heights of smash-ridge tillage treatment on tobacco planting soil samples, both bacterial richness (Ace index and Chao1 index) and diversity (Shannon Index and Simpson Index) were significantly enhanced compared to the control samples. This enhancement indicates that smash-ridge tillage substantially influences the structural characteristics of soil bacterial communities. The alterations in soil bacterial community structure characteristics ultimately have implications for the functionality of the entire soil microbial ecosystemCitation16, which is consistent with the fundamental outcomes of this research.
The diversity of soil microbial bacterial and fungal communities directly influences microbial functional diversity (). Existing research has shown a close correlation between soil microbial community structure and carbon source metabolismCitation17. The interactions between copiotrophs (R-strategists) and oligotrophs (K-strategists), which possess functional genes related to metabolism, genetic information processing, and organic systems, in the soil promote the absorption of nutrients and the dissolution of iron and small molecules from the soil, accelerating their own growth, and enhancing bacterial metabolic activity, thereby increasing bacterial functional community diversityCitation17,Citation18.
This study also found that tillage practices affect soil enzyme activity (). The transformation of nitrogen, phosphorus, and the metabolism and transport of carbohydrates in the soil are closely related to urease, transformation enzymes, and alkaline phosphataseCitation19,Citation20. Fine ridge tillage improves the crop-soil environment through physical means, giving vitality to crop roots and promoting downward growthCitation21. This leads to the release of more enzymes to enhance the exchange of nutrients with the soil microenvironmentCitation2, resulting in higher soil enzyme activity in fine ridge tillage compared to traditional tillage. Enzymes in the soil originate not only from crop roots but also from the metabolic activities of soil microorganismsCitation22. Heterotrophic bacteria release enzymes with corresponding functions to participate in soil nutrient cycling for their own developmentCitation23.
Compared to fine smash tillage, traditional tillage hinders the development of crop surface roots and soil microbial activityCitation24, resulting in low soil enzyme activity. Fine ridge tillage improves the physical structure of cultivated soil, promotes the growth of crop roots and the development of soil microorganisms, and releases corresponding enzymes, accelerating soil nutrient cycling. As a result, soil enzyme activity in fine ridge tillage is higher than that in traditional tillage. Other studies have also shown that soil enzyme activity not only reflects the degree of organic matter hydrolysis but also indicates the ability to utilize carbon sourcesCitation25. This study also found that soil enzyme activity significantly influences microbial functional diversity, indicating that fine ridge tillage can indirectly enhance microbial functional diversity by increasing enzyme activity. This strengthens the nutrient utilization ability of soil microbial communities, improves community functional structure, enhances soil ecosystem stability, and enhances the performance of tobacco plants in field agronomic traits.
In this study, a total of 677 genus-level OTUs were annotated through comparison. Using random forest regression analysis, the top 10 microbial OTUs with the highest correlation to the total leaf area of individual tobacco plants (number of leaves × leaf area) were selected. Granulicella (genus of granule-forming bacteria) and Ilumatobacter (genus of acidophilic bacteria) both belong to the Acidobacteria phylum, and are typically associated with carbon source utilization in the soilCitation26. Previous research suggests that Granulicella can serve as an indicator organism for evaluating specific soil types and the succession patterns of soil microbial communities in typical black soil areas under different land use practicesCitation11,Citation27. The latter is sensitive to nitrogen content in the soil environment and plays a role in enhancing the stability of soil microbial bacterial community structure, promoting bacterial synergistic interactions, and increasing resistance to interference. Furthermore, related studies have found significant negative correlations between the relative abundance of these two microbial genera and the distribution and quantity of some soil-borne pathogenic microorganisms in the soil environmentCitation28. This indicates their significant potential for application in inhibiting soil-borne pathogenic microbial agents.
The genus Modestobacter belongs to the Actinobacteria phylum. Studies have found that this microbial genus is closely associated with the cycling of phosphorus (P) in soil due to the presence of functional genes such as alkaline phosphatase genes (phoA/phoD) and phosphorus transport-related genes (phoU, phnC, phnD, phnE, phoB, phoH, phoP, phoR, pitH, ppk, pstA, pstB, pstC, and pstS). These genes contribute to the efficient utilization of P in the soil and help plants adapt to adverse soil environmentsCitation29,Citation30.
The genus Bacillus comprises aerobic or facultative anaerobic bacteria, many of which possess significant agricultural importance. Most species within this genus are saprophytic bacteria capable of producing diverse beneficial metabolites. As a result, they play a crucial role in promoting crop growth and facilitating carbon sequestrationCitation31,Citation32 This bacterial genus is also a key species involved in the formation of biofilms in soil. It assists plants in resisting environmental stresses, adapting to extreme conditions, promoting soil aggregation, and enhancing soil structure stability. Nitrobacteria are involved in the biogeochemical cycle of nitrogen, improving the efficiency of plant nitrogen utilizationCitation33. Research has shown a close relationship between the abundance of Nitrobacteria in soil and the disease resistance of tobacco plantsCitation34. Kaistobacter has been considered a signature microorganism for soil improvement in some studies, and its presence significantly increases in intercropping agricultural systems.
Sphingomonas, as a common beneficial microorganism in soil, can produce plant growth hormones, promote root growth, increase plant biomass, and enhance plant stress toleranceCitation35.For example, the genus Sphingomonas is significantly enriched in disease-resistant rice and can interfere with the biosynthetic pathway of virulence factors in rice bacterial pathogens by secreting small-molecule signals such as salicylic acid, thereby improving rice disease resistanceCitation36,Citation37.It can also act as an antagonist of plant pathogenic fungi and secrete valuable exopolysaccharidesCitation38. Research has also shown that continuous cropping reduces the abundance of Sphingomonas in soilCitation39. The above results indicate that smash-ridge tillage not only enhances the microbial community diversity in cultivated soil but also increases the abundance of functional microorganisms involved in soil nutrient transformation, thereby playing an important role in maintaining the stability of the soil microecological environment.
Previous studies have shown that root growth can release enzymes and carbon sourcesCitation38, thereby influencing the microbial decomposition of carbon sources and promoting an increase in soil microbial diversityCitation40. There are differences in the microbial community structure between smash-ridge tillage and traditional farming, and the reason for this could be that smash-ridge tillage promotes root growth, leading to the release of more carbon sources by the roots and consequently enhancing microbial functional diversity. Research has demonstrated that diseased roots increase the release of carboxylic acids and amine carbon sources, thereby enhancing the utilization of these carbon sources by soil microorganismsCitation41–43This indicates that smash-ridge tillage can reduce the probability of crop root disease, as diseased roots secrete fewer carboxylic acids and amine carbon sources into the surrounding soil, altering the microbial capacity to utilize carbon sources.
Among the top twenty functional pathways identified in this study, twelve of them are closely related to nitrogen utilization and the biogeochemical cycle of nitrogen. Additionally, when comparing the functional pathways of different farming samples, it was found that the expression levels of 15 functional pathways were significantly higher in the raised bed soil samples at a depth of 50 cm compared to the control samples. This clearly indicates that smash-ridge tillage can effectively activate the ecological functions of bacterial communities, enhance the functional activity of soil microorganisms, and assist plants in better absorbing and transporting nutrients from the soil environment.
5. Conclusions
This study investigates the effects of different farming practices on tobacco agronomic traits, soil enzyme activity, microbial community structure, and functional metabolism in tobacco-growing soils. The results demonstrate that smash-ridge tillage, compared to the conventional tillage method, significantly improves the bacterial community structure, increases functional groups involved in nutrient metabolism, and enhances soil microbial diversity. These improvements ultimately manifest in the agronomic status of tobacco plants when utilizing smash-ridge tillage. Smash-ridge tillage enhances the activities of urease, nitrite reductase, alkaline phosphatase, and aryl sulfatase, which are enzymes involved in nutrient cycling. The smash-ridge tillage technique promotes the capacity of soil microorganisms for nutrient metabolism, thereby enhancing the overall functional diversity of soil microorganisms. The diversity of soil microbial communities, functional diversity, enzyme activity, and agronomic traits of tobacco plants all indicate that the smash-ridge tillage treatment at a depth of 50 cm outperforms the treatment at a depth of 30 cm. In summary, from the perspective of soil microbial ecology, it can be concluded that smash-ridge tillage technology promotes nutrient cycling, improves soil quality, and enhances the agronomic performance of tobacco plants. This study provides a scientific basis for the application and development of smash-ridge tillage technology in the southwestern tobacco-growing regions of China. The impact of farming practices on soil quality is a long-term process. Therefore, with the long-term use of smash-ridge tillage, changes in soil physical structure and soil microbial ecology will also occur. This study only investigates the effects of smash-ridge tillage at different depths on soil enzyme activity, microbial community structure, and functional diversity for one year. In the future, long-term monitoring and tracking studies will be conducted to further investigate the changes in soil microbial ecology, as well as to explore research on soil aggregates, soil carbon content, and soil diseases.
Author contributions
Methodology, He Jixian,Lai Yu; software, Chen Qi.; formal analysis, Liu Weijie; investigation, Yu Jian, Li Fucheng.; resources, Yu Shikang; writing – original draft preparation, Zhu Bo; writing – review and editing, Zhu Bo.; visualization, Zhu Bo, Gu Huizhan.; supervision, Li Fucheng. All authors have read and agreed to the published version of the manuscript.
Disclosure statement
No potential conflict of interest was reported by the author(s).
Data availability statement
The data will be available from the corresponding author following a reasonable request
Additional information
Funding
References
- Chen Y, Yang L, Zhang L, Li J, Zheng Y, Yang W, Deng L, Gao Q, Mi Q, Li X, et al. Autotoxins in continuous tobacco cropping Soils and their management. Front Plant Sci. 2023;14:14. doi:10.3389/fpls.2023.1106033.
- Bai Y, Wang G, Cheng Y, Shi P, Yang C, Yang H, Xu Z. Soil acidification in continuously cropped tobacco alters bacterial community structure and diversity via the accumulation of phenolic acids. Sci Rep. 2019;9(1):12499. doi:10.1038/s41598-019-48611-5.
- Wu F, Zhai L, Xu P, Zhang Z, Baillo EH, Tolosa LN, Kimotho RN, Jia X, Guo H. Effects of deep vertical rotary tillage on the grain yield and resource use efficiency of Winter wheat in the Huang-Huai-Hai Plain of China. J Integr Agr. 2021;20(2):593–12. doi:10.1016/S2095-3119(20)63405-0.
- Wang Z, Li Y, Li T, Zhao D, Liao Y. Tillage practices with different soil disturbance shape the rhizosphere bacterial community throughout crop growth. Soil And Tillage Res. 2020;197:104501. doi:10.1016/j.still.2019.104501.
- Wenhao Y, Zhang X, Wu L, Rensing C, Xing S. Short-term application of magnesium fertilizer affected soil microbial biomass, activity, and community structure. J Soil Sci Plant Nutr. 2021;21(1):675–689. doi:10.1007/s42729-020-00392-x.
- Ding J, Li F, Le T, Xu D, Zhu M, Li C, Zhu X, Guo W. Tillage and seeding strategies for wheat optimizing production in harvested rice fields with high soil moisture. Sci Rep. 2021;11(1):119. doi:10.1038/s41598-020-80256-7.
- Li X, Wei B, Xu X, Zhou J. Effect of deep vertical rotary tillage on soil properties and Sugarcane biomass in rainfed dry-land regions of Southern China. Sustainability. 2020;12(23):10199. doi:10.3390/su122310199.
- Wei B, Han S, He G. Smash-ridging cultivation improves crop production. Outlook Agric. 2022;51(2):173–177. doi:10.1177/00307270211001881.
- Burns RG. Enzymes in the environment III - Preface. Soil Biol Biochem. 2008;40:2067–2067. doi:10.1016/S0038-0717(08)00262-9.
- Bokulich NA, Kaehler BD, Rideout JR, Dillon M, Bolyen E, Knight R, Huttley GA, Gregory Caporaso J. Optimizing taxonomic classification of marker-gene amplicon sequences with QIIME 2’s Q2-feature-classifier Plugin. Microbiome. 2018;6:90. doi:10.1186/s40168-018-0470-z.
- Griffiths BS, Philippot L. Insights into the resistance and resilience of the soil microbial community. FEMS Microbiol Rev. 2013;37(2):112–129. doi:10.1111/j.1574-6976.2012.00343.x.
- Bardgett RD, Jones AC, Jones DL, Kemmitt SJ, Cook R, Hobbs PJ. Soil microbial community patterns related to the history and intensity of grazing in sub-montane ecosystems. Soil Biol Biochem. 2001;33(12–13):1653–1664. doi:10.1016/S0038-0717(01)00086-4.
- Nannipieri P, Ascher J, Ceccherini MT, Landi L, Pietramellara G, Renella G. Microbial diversity and soil functions. Eur J Soil Sci. 2017;68:12–26. doi:10.1111/ejss.4_12398.
- Weidhuner A, Hanauer A, Krausz R, Crittenden SJ, Gage K, Sadeghpour A. Tillage impacts on soil aggregation and aggregate-associated carbon and nitrogen after 49 years. Soil And Tillage Res. 2021;208:104878. doi:10.1016/j.still.2020.104878.
- Zhou M, Liu C, Wang J, Meng Q, Yuan Y, Ma X, Liu X, Zhu Y, Ding G, Zhang J, et al. Soil aggregates stability and storage of soil organic carbon respond to cropping systems on black Soils of Northeast China. Sci Rep. 2020;10(1):265. doi:10.1038/s41598-019-57193-1.
- Jia S, Zhang X, Chen X, McLaughlin NB, Zhang S, Wei S, Sun B, Liang A. Long-term conservation tillage influences the soil microbial community and its contribution to soil CO2 emissions in a mollisol in Northeast China. J Soils Sediments. 2016;16(1):1–12. doi:10.1007/s11368-015-1158-7.
- Kaiser K, Wemheuer B, Korolkow V, Wemheuer F, Nacke H, Schöning I, Schrumpf M, Daniel R. Driving forces of soil bacterial community structure, diversity, and function in temperate grasslands and forests. Sci Rep. 2016;6(1):33696. doi:10.1038/srep33696.
- Wu Z, Hao Z, Sun Y, Guo L, Huang L, Zeng Y, Wang Y, Yang L, Chen B. Comparison on the structure and function of the rhizosphere microbial community between healthy and Root-Rot Panax Notoginseng. Appl Soil Ecol. 2016;107:99–107. doi:10.1016/j.apsoil.2016.05.017.
- Wang Y, Liu L, Tian Y, Wu X, Yang J, Luo Y, Li H, Awasthi MK, Zhao Z. Temporal and spatial variation of soil microorganisms and nutrient under white clover cover. Soil And Tillage Res. 2020;202:104666. doi:10.1016/j.still.2020.104666.
- Qin X, Liu Y, Huang Q, Zhao L, Xu Y. Effects of sepiolite and biochar on enzyme activity of soil Contaminated by cd and atrazine. Bull Environ Contam Toxicol. 2020;104(5):642–648. doi:10.1007/s00128-020-02833-w.
- Singh DK, Kumar S. Nitrate reductase, arginine deaminase, urease and dehydrogenase activities in natural soil (ridges with forest) and in cotton soil after acetamiprid treatments. Chemosphere. 2008;71(3):412–418. doi:10.1016/j.chemosphere.2007.11.005.
- Hou D, Bi J, Ma L, Zhang K, Li D, Rehmani MIA, Tan J, Bi Q, Wei Y, Liu G, et al. Effects of soil moisture content on germination and physiological characteristics of rice seeds with different specific gravity. Agronomy. 2022;12(2):500. doi:10.3390/agronomy12020500.
- Zhang J, Li F, Liao P, Khan A, Hussain I, Iqbal A, Ali I, Wei B, Jiang L. Smash ridge tillage strongly influence soil functionality, physiology and rice yield. Saudi J Biol Sci. 2021;28(2):1297–1307. doi:10.1016/j.sjbs.2020.11.054.
- Jin K, Sleutel S, Buchan D, De Neve S, Cai DX, Gabriels D, Jin JY. Changes of soil enzyme activities under different tillage practices in the Chinese loess plateau. Soil And Tillage Res. 2009;104(1):115–120. doi:10.1016/j.still.2009.02.004.
- Wang Z, Liu L, Chen Q, Wen X, Liao Y. Conservation tillage increases soil bacterial diversity in the dryland of Northern China. Agron Sustain Dev. 2016;36:28. doi:10.1007/s13593-016-0366-x.
- Zheng B, Jing Y, Zou Y, Hu R, Liu Y, Xiao Z, He F, Zhou Q, Tian X, Gong J, et al. Responses of tobacco growth and development, nitrogen use efficiency, crop yield and economic benefits to smash ridge tillage and nitrogen reduction. Agronomy. 2022;12(9):2097. doi:10.3390/agronomy12092097.
- Hartmann M, Six J. Soil structure and Microbiome functions in agroecosystems. Nat Rev Earth Environ. 2023;4(1):4–18. doi:10.1038/s43017-022-00366-w.
- Costa OYA, De Hollander M, Pijl A, Liu B, Kuramae EE. Cultivation-independent and cultivation-dependent metagenomes reveal genetic and enzymatic potential of microbial community involved in the degradation of a complex microbial polymer. Microbiome. 2020;8(1):76. doi:10.1186/s40168-020-00836-7.
- Li H, Xu Z, Yang S, Li X, Top EM, Wang R, Zhang Y, Cai J, Yao F, Han X, et al. Responses of soil bacterial communities to nitrogen deposition and precipitation increment are closely linked with aboveground community variation. Microb Ecol. 2016;71(4):974–989. doi:10.1007/s00248-016-0730-z.
- Zhao F, Zhang Y, Dong W, Zhang Y, Zhang G, Sun Z, Yang L. Vermicompost can suppress Fusarium Oxysporum f. Sp. Lycopersici via generation of beneficial bacteria in a long-term tomato monoculture soil. Plant Soil. 2019;440(1–2):491–505. doi:10.1007/s11104-019-04104-y.
- Jiang Z-M, Zhang B-H, Sun H-M, Zhang T, Yu L-Y, Zhang Y-Q. Properties of Modestobacter Deserti Sp. Nov., a kind of novel phosphate-solubilizing Actinobacteria inhabited in the desert biological soil crusts. Front Microbiol. 2021;12:742798. doi:10.3389/fmicb.2021.742798.
- Luo G, Ling N, Nannipieri P, Chen H, Raza W, Wang M, Guo S, Shen Q. Long-term fertilisation regimes affect the composition of the alkaline phosphomonoesterase encoding microbial community of a vertisol and its derivative soil fractions. Biol Fertil Soils. 2017;53(4):375–388. doi:10.1007/s00374-017-1183-3.
- Wang J, Chapman SJ, Yao H. The effect of storage on microbial activity and bacterial community structure of drained and flooded paddy soil. J Soils Sediments. 2015;15(4):880–889. doi:10.1007/s11368-014-1053-7.
- Patel SKS, Kumar P, Singh M, Lee J-K, Kalia VC. Integrative approach to produce hydrogen and polyhydroxybutyrate from biowaste using defined bacterial cultures. Bioresour Technol. 2015;176:136–141. doi:10.1016/j.biortech.2014.11.029.
- Wu Y, He J, Liu W, Cheng W, Shaaban M, Jiang Y. The effects of continuous straw returning strategies on SOC balance upon fresh straw incorporation. Environ Res. 2023;232:116225. doi:10.1016/j.envres.2023.116225.
- Bian F, Zhang X, Zhong Z, Wen X, Xiu C, Li Q, Huang Z. Introducing sedum affects root-soil interface phytoremediation of heavy metals in lei bamboo forest and potential risks from edible bamboo shoots. Land Degrad Dev. 2022;34(6):1820–1829. doi:10.1002/ldr.4571.
- Luo Y, Wang F, Huang Y, Zhou M, Gao J, Yan T, Sheng H, An LSS. Sphingomonas sp. Cra20 increases plant growth rate and alters rhizosphere microbial community structure of Arabidopsis thaliana under drought stress. Front Microbiol. 2019;10. doi:10.3389/fmicb.2019.01221.
- Asaf S, Numan M, Khan AL, Al-Harrasi A. Sphingomonas: from diversity and genomics to functional role in environmental remediation and plant growth. Crit Rev Biotechnol. 2020;40:138–152. doi:10.1080/07388551.2019.1709793.
- Matsumoto H, Fan X, Wang Y, Kusstatscher P, Duan J, Wu S, Chen S, Qiao K, Wang Y, Ma B, et al. Bacterial seed endophyte shapes disease resistance in rice. Nat Plants 2021. 2021;7(1):60–72. doi:10.1038/s41477-020-00826-5.
- Lorenz K, Preston CM, Krumrei S, Feger K-H. Decomposition of needle/leaf litter from scots pine, black cherry, common oak and European beech at a conurbation forest site. Eur J Forest Res. 2004;123(3):177–188. doi:10.1007/s10342-004-0025-7.
- Pengthamkeerati P, Motavalli PP, Kremer RJ. Soil microbial activity and functional diversity changed by compaction, poultry litter and cropping in a claypan soil. Appl Soil Ecol. 2011;48(1):71–80. doi:10.1016/j.apsoil.2011.01.005.
- Wang X, Xia K, Yang X, Tang C. Growth strategy of microbes on mixed carbon sources. Nat Commun. 2019;10(1):1279. doi:10.1038/s41467-019-09261-3.
- Li Z, Wang K-F, Zhao X, Ti H, Liu X-G, Wang H. Manganese-mediated reductive functionalization of activated aliphatic acids and primary amines. Nat Commun. 2020;11(1):5036. doi:10.1038/s41467-020-18834-6.