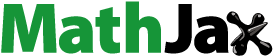
ABSTRACT
The synthesis of glycopolymers by copolymerising an allyl glucosamine (AG) monomer with co-monomers methyl methacrylate (MMA), acrylonitrile (AN) and 2-hydroxyethyl methacrylate (HEMA) was investigated via free-radical polymerisation of 2,2-azobisisobutyronitrile (AIBN) in dimethylformamide (DMF). Three new copolymers, poly(AG-co-MMA), poly(AG-co-AN) and poly(AG-co-HEMA), were obtained. The chemical structures of the glycopolymers were analysed using 1H-NMR, 13C-NMR and FTIR. The thermal properties and degradation kinetics of the three glycopolymers were examined by thermogravimetric (TG) analysis at different heating rates. The effects of different co-monomers on the copolymerisation yield, thermal properties and biological activities of the resulting glycopolymers were investigated. The activation energies of the decomposition stages were calculated using the Flynn–Wall–Ozawa (FWO) and Kissinger methods. Furthermore, the biological activity of AG monomers and glycopolymers was studied and compared to chitosan. Poly(AG-co-HEMA) had the most significant effect on MCF-7 cell viability, and all glycopolymers have a low toxic effect profile on MCF-7 cell lines.
1. Introduction
Synthetic polymers containing carbohydrates are commonly referred to as glycopolymers. They have attracted the attention because of their potential significance in biological applications. Modifying synthetic polymers using carbohydrate derivatives enables tuning the properties of the resulting polymers, including their biodegradability and biocompatibility, [Citation1] resulting in more environmentally friendly alternative polymer products. Carbohydrate-modified polymers have applications in clinical diagnostics for targeted gene therapies and prosthetic uses [Citation2,Citation3], drug delivery systems, tissue engineering, dental applications, contact lens materials and bioimplants [Citation4].
Natural biomass-derived carbohydrates are a significant precursor for the synthesis of biodegradable polymers [Citation5–7] and provide alternatives to materials with fossil fuel-derived chemical structures [Citation8–10]. Thus, they are also an important way to reduce dependency on fossil fuel-based resources [Citation11]. The N-deacetylated derivative of chitin, chitosan, a naturally occurring polysaccharide in insects and crustaceans, has attracted interest for such approaches owing to its availability, advantageous structural properties and biodegradability [Citation12,Citation13].
Glucosamine, the monomer unit of chitosan, is produced on a large scale and is a relatively cheap source of carbohydrates [Citation14]. Glucosamine derivatives have applications and interests in antitumor drugs [Citation15], coagulated drugs [Citation16], gelators [Citation17], and adjuvant drugs [Citation18]. However, glucosamine derivatives are scarce owing to relatively long synthetic routes using protecting groups [Citation19,Citation20].
Vinyl sugar monomers, synthesised via a reaction of a vinyl-containing acid chloride [Citation21] or anhydride [Citation22] with protected sugars, produce many synthetic polymers with different structures and properties [Citation23]. Polyacrylonitriles (PAN) are an important class of polymers with excellent thermal and mechanical stability. They are useful membrane materials in pervaporation, water treatment, gas separation, enzyme immobilisation, biomedical applications and purification of biochemical products [Citation24–26]. Copolymerisation with acrylonitrile (AN) has been used to broaden the applications of PAN-based membranes [Citation27,Citation28].
Methacrylic monomers with pendant saccharide residues [Citation29], has been deployed for synthesising methacrylic glycopolymers [Citation30],such as 3-O-methacryloyl-1,2:3,4-di-O-isopropylidene-d-galactopyranose [Citation31],3-O-methacryloyl-1.2,5.6-diisopropylidene-d-gluco-furanose [Citation31], and monomethacroyl-sucrose [Citation32]. They were homopolymerised or copolymerised via atom-transfer radical polymerization [Citation33] or free-radical methods initiated with 2,2-azobisisobutyronitrile (AIBN) [Citation25]. AIBN was used as the initiator in this study because it has the advantage of not producing oxygenated by-products and undesirable color changes. A methacrylate benzodioxinone monomer was copolymerised with methyl methacrylate (MMA) and 2-hydroxyethyl methacrylate (HEMA) to create a photochemically sensitive random copolymer [Citation34]. Thermoresponsive glycopolymers of poly(N-isopropylacrylamide)-co-(2-[β-manno[oligo]syloxy] ethyl methacrylate)s have also been synthesised from oligo-β-mannosyl ethyl methacrylates (obtained via biocatalysts) [Citation35].
A library of glucomonomers was developed using N-allyl glucosamine (AG) for synthesis of various glycopolymers. These AG glucomonomers have a polymerisable group connected via glycosidic bonds. Thanks to these properties, they could be combined with other substances and be used to synthesize biofunctional materials for applications in tissue engineering, drug-delivery systems and biosensing [Citation36]. Further, hydrophobic methacrylate monomers were used to minimise weak solvent interactions with the glycopolymer [Citation24,Citation35]. Hence, there is a large potential for future research to advance the opportunities provided by sugar-based copolymers and investigate the properties derived from the high densities of functional groups that classical linear polymer analogues possess [Citation37,Citation38].
The low toxicity, biodegradability, stability and renewability of glycopolymers make them very promising for biomedical applications [Citation39–41]. Human breast cancer cell lines are commonly used as cell culture models to study the in vitro biological and toxic effects of biopolymers [Citation42–44]. Poly(AG-co-MMA) is a type of amorphous polymer in the acrylate family [Citation45]. It is a biocompatible material with a low toxicity profile that does not induce any immune response in the organism to which it is administered. Consequently, it has attracted interest in biomedical applications for several years [Citation46]. Poly(AG-co-HEMA) is a non-toxic, hydrophilic polymer with excellent biocompatibility that has been described as a promising biomaterial for clinical use [Citation47].
In this study, an N-alloc glucosamine monomer (AG) () is used in the radical homopolymerisation of the novel sugar-carrying glycopolymers with comonomers MMA, AN and HEMA via free radical copolymerization using AIBN as initiator in DMF at 72 °C for 24 h (). The thermal degradation kinetics of these glycopolymers are investigated. The apparent activation energies (Ea) for thermal degradation of these glycopolymers are calculated using the Kissinger and Flynn–Wall–Ozawa (FWO) methods. These findings (vide infra) indicate that the new glycopolymers are promising candidates for preparing biodegradable polymers for biological applications. These new allyl glucosamine copolymers are good candidates for the biomedical applications such as targeted and controlled drug delivery, improvement i aqueous solubility and stability of drugs, tissue engineering, and antimicrobial coatings in further studies.
2. Experimental
2.1. Materials
All the following chemicals were obtained from Sigma-Aldrich and Merck A.G: glucosamine hydrochloride (U.S.A, stated purity≥98.0%), allylchloroformate (Germany, stated purity≥99.0%), ethyl acetate (EtOAc) (Germany, stated purity 99.0%), diethyl ether (Germany, stated purity≥99.0%), n-hexane (Germany, stated purity 99.0%), toluene (Germany, stated purity 99.0%), sodium hydrogen carbonate (NaHCO3) (Germany, stated purity 98.0%), sodium sulphate (Na2SO4) (Germany, stated purity 98.0%), MMA (U.S.A, stated purity≥99.0%), HEMA (U.S.A, stated purity≥99.0%), AN (U.S.A, stated purity≥99.0%) and AIBN (Germany, stated purity 98.0%).
2.2. Instrumentation
Nuclear magnetic resonance (NMR) spectra [Citation48] H and 13C) were recorded in dimethyl sulfoxide-d6 (DMSO-d6) at ambient temperature using a Varian AS 400+ Mercury FT NMR spectrometer (400 MHz). Attenuated total reflectance-Fourier-transform infrared (ATR – FTIR) spectroscopy (Perkin Elmer 100 FTIR with PIKE Gladi ATR accessory) was performed at room temperature. The test wavenumber range of FTIR spectra was 400–4000 cm−1. Column chromatography and thin-layer chromatography were performed on silica gel G-60 (Merck 7734) and precoated aluminium plates (Merck 5554), respectively.
Thermogravimetric analysis (TGA) and derivative TG (DTG) analysis were performed on a Perkin Elmer Diamond TA/TGA with heating rates of 5°C/min, 10°C/min, 15°C/min and 20°C/min under N2 atmosphere. The temperature ranged from 30°C and 600°C. The weight loss (TG curve) and its first derivative (DTG curve) were recorded simultaneously as a function of temperature.
Molecular weights were determined using a gel permeation chromatography (GPC) instrument equipped with a Waters Styragel column (HR series 2, 3, 5E) using tetrahydrofuran as the eluent at a flow rate of 1 mL/min and a Waters 410 differential refractometer detector.
2.3. Synthesis of the 2-N-allyloxycarbonyl-2-amino-2-deoxy-α-d-glucopyranose (1)
Glucosamine hydrochloride (7.0 g, 32 mmol) was dissolved in water (150 mL), followed by a solution of NaHCO3 (6.35 g, 75 mmol) in water (150 mL) was added. The reaction mixture was cooled in an ice bath. Allyl chloroformate (4 mL, 32 mmol, 1 equiv.) was added to the flask dropwise. The mixture was stirred at 0°C for 3 h (). The reaction mixture was concentrated under reduced pressure and dried overnight under high vacuum to yield a white solid compound 1 (9.00 g, 98%). [HRMS (ES−) for C10H17NO7Na required 286.0897, found 286.0891] [Citation48]. H NMR (400 MHz, DMSO-d6) δ = 6.16 (1 H, d, J = 3.6 Hz, H-1), 5.81 (1 H, m, –CH=CH2), 5.22 (2 H, m, –CH=CH2), 4.95 (1 H, dd, J = 3.6 Hz, H-2), 4.55 (2 H, m, –CH2), 4.45 (1 H, m, H-4), 4.35 (1 H, dd, J = 10.4, 2.3 Hz, H6a), 4.25 (1 H, dd, J = 10.4, 4.9 Hz, H6b), 4.36–4.15 (2 H, m, –CH2), 4.05 (1 H, s, H-3), 4.00 (1 H, m, H-5) and 3.64 (4 H, s, OH) [Citation49].
2.4. Synthesis of 1,3,4,6-tetra-O-acetyl-2-N-allyloxycarbonyl-2-amino-2-deoxy-α-D-glucopyranose (N-alloc glucosamine, AG)
Dried compound 1 (2.6 g, 1 mmol) was dissolved in dry pyridine (2.5 mL volume based on 4 OH groups) under N2, yielding a yellow solution. The reaction mixture was stirred for 24 hours after adding 5 mmol of acetic anhydride (5 equiv. 1.25 equiv. for each of the 4 hydroxyl groups). Further, the reaction mixture was extracted using EtOAc (3 × 25 mL) and 3% aqueous hydrochloric acid (3 × 20 mL). The organic extracts were combined and washed sequentially with water (3 × 10 mL) and a saturated aqueous solution of NaHCO3 (2 × 20 mL). The combined organic extracts were dried over Na2SO4, filtered and concentrated under vacuum to yield the acetylated product 1 as light brown oil (3.53 g, 83%). The material was crystallised by titrating with diethyl ether with an Rf of 0.53 (in v/v EtOAc/hexane 1:1) and m.p. of 104ºC–106ºC (Recrystallization of compound AG (N-alloc glucosamine) from diethyl ether produced a white crystalline solid.) mp 119–121 ºC. [α]D [Citation23] + 58.9 (c 0.6, CHCl3), lit., 115 [α]D [Citation22] +27.6 (c 2.36, CHCl3). 1H NMR (400 MHz, CDCl3) ∂ ppm; 6.26 (d, J = 3.6 Hz, 1 H, H-1), 5.86 (m, 1 H, -CH=CH2), 5.23–5.03 (m, 3 H, H-3, -CH=CH2), 4.91 (d, J = 8.8 Hz, 1 H), NHC=O (-assigned by D2O exchange-), 4.60–4.50 (m, 2 H, -CH2), 4.35 (dd, J = 8.4, 9.6 Hz, 1 H, H-4), 4.21 (ddd, J = 3.6, 9.8, 10.2 Hz, 1 H, H-2), 4.15 (dd, J = 5.7, 10.3 Hz, 1 H, H-6a), 4.04–3.99 (m, 1 H, H-6b), 3.92–3.89 (m, 1 H, H-5), 2.16/2.06/2.02/1.98 (4 × 3 H, 3 × s, COCH3). 13C NMR (300 MHz, CDCl3) ∂ ppm; 171.2/170.7/169.2/168.7 (H3CC=O), 155.4 (NHC=O), 132.2 (-CH=CH2), 118.1 (-CH=CH2), 91.8 (C-1), 70.6 (C-3), 69.7 (C-5), 67.6 (C-4), 66.1 (-CH2), 61.5 (C-6), 52.8 (C-2), 20.9/20.7 (x2)/20.6 (O=CCH3). m/z (C I + ve) for C18H25NO11Na required 454.1325 found 454.1319. IR cm−1 (KBr thin film); 3266 (NHC=O), 1754 (O – C=O), 1529 (C=C), 1371, 1229 (C – C(O) –C), 1028, 927.
2.5. Copolymerisation of N-alloc glucosamine monomer (AG (N-alloc glucosamine)) with methyl methacrylate (MMA) to synthesise poly(AG-co-MMA)
The new glycopolymer poly(AG-co-MMA) from AG (N-allyl glucosamine) monomer (0.25 g, 0.58 mmol) and MMA (0.31 mL, 2.9 mmol), AN (0.19 mL, 2.9 mmol) and HEMA (0.35 mL, 2.9 mmol) at a 1:5 mol monomer rate. A conventional free-radical co-polymerisation was conducted using AIBN (2.0%, based on the total weight of the monomer) as an initiator in 2 mL of dimethylformamide (DMF) at 72°C for 24 h, forming glycopolymers poly(AG-co-MMA), poly(AG-co-AN) and poly(AG-co-HEMA) with approximately 3%, 10% and 7% conversion, respectively, in an all-glass Schlenk flask under inert atmosphere. The synthesised glycopolymer was precipitated in methanol and dried under vacuum at 40°C for 24 h and analysed using FTIR and NMR spectra. 1H NMR (d6-DMSO, 400 MHz): δ 5.98 (d, J = 3.6 Hz, H-1), 5.20 (m, H-3), 5.10 (dd, 1 H, J = 3.6, 10.2 Hz, H-2), 4.60–4.44 (m, -CH2, H-7,8), 4.15 (dd, J = 8.4, 9.6 Hz, H-4), 4.07 (m, H-5), 3.98 (m, H-6), 3.92 (m, H-9), 3.54 (s, metoxyl protons), 2.80–2.20 (methyl protons). 13C NMR: 178.0–162.2 (carbonyl carbons), 90.2 (C-1), 70.8, 69.5. 68.7, 65.4, 61.8 (C-2, C-3, C-4, C-5, C-6), 52.6, 52.0 (-OCH3, -O-CH2-) 37.1–21.0 (aliphatic carbons).
2.6. Copolymerization of N-alloc glucosamine monomer (AG) with acyrolonitrile (AN) (poly(ag-co-AN)
The new copolymer (Poly(AG-co-AN)) was obtained from reaction of N-alloc glucosamine monomer (AG) (0.25 g, 0.58 mmol) and acrylonitrile (AN) (0.19 mL, 2.9 mmol) (1:5 mol monomer rate) via free radical copolymerization using AIBN (2,2-azobisisobutyronitrile) (2.0%, based on the total weight of the monomer) as initiator. Reaction in 2 mL of DMF at 72 °C for 24 h (Schlenk) afforded ca.10% conversion to the copolymer (Poly(AG-co-AN)). The copolymer was precipitated in methanol and dried under vacuum at 40 °C for 24 h and then was characterised using FTIR and NMR spectra. 1H NMR (d6-DMSO, 400 MHz): δ 5.99 (d, J = 3.6 Hz, H-1), 5.16 (m, H-3), 4.95 (dd, 1 H, J = 3.6, 10.2 Hz, H-2), 4.15–4.07 (m, -CH2, H-7,8), 3.98 (dd, J = 8.4, 9.8 Hz, H-4), 3.15 (m, H-5, H-6), 2.90–2.48 (methyl protons). 13C NMR: 176.0–161.0 (carbonyl carbons), 132.0 (−CN), 90.0 (C-1), 70.4, 69.0. 68.4, 65.2, 62.0 (C-2, C-3, C-4, C-5, C-6), 52.6 (-O-CH2-) 36.9–21.7 (aliphatic carbons).
2.7. Copolymerization of N-alloc glucosamine monomer (AG) with 2-hydroxyethyl methacrylate(hema) (poly(ag-co-HEMA))
The new copolymer (Poly(AG-co-HEMA)) was obtained from N-alloc glucosamine monomer (AG (N-allyl glucosamine)) (0.25 g, 0.58 mmol) and 2-hydroxethyl methacrylate (HEMA) (0.35 mL, 2.9 mmol) (1:5 mol monomer rate) via free radical copolymerization using AIBN (2,2-azobisisobutyronitrile) (2.0%, based on the total weight of the monomer) as initiator. Carrying out this polymerisation in 2 mL of DMF at 72 °C for 24 h (in a Schlenk) afforded (Poly(AG-co-HEMA)) in ca. 7% conversion. The resulting polymer was precipitated in methanol and dried under vacuum at 40 °C for 24 h and characterized using FTIR and NMR spectra. 1H NMR (d6-DMSO, 400 MHz): δ 6.12 (d, J = 3.6 Hz, H-1), 5.19 (m, H-2), 5.02 (m, H-3, H-4), 4.18–3.90 (m, -H-5, H-6, H-7), 3.62 (br s, H-11, H-12), 3.38 (br s, -OH), 2.70–1.90 (methyl protons). 13C NMR: 179.1–162.0 (carbonyl carbons), 90.1 (C-1), 70.7, 69.3. 68.6, 65.4, 61.6, 61.2 (C-2, C-3, C-4, C-5, C-6, C-8), 58.0, 57.8 (C-14, C-15) 36.7–21.1 (aliphatic carbons).
2.8. Kinetic analysis
In the literature, the thermal behaviour of many polymers has been examined using the thermogravimetric (TG) method and some physicochemical data have been determined using the obtained thermograms [Citation50–52]. Calculation of the kinetic parameters A, E and n can be made using several different methods based on integral or differential methods [Citation53–55]. Kinetic calculations using TG analysis (TGA) data are made using mass-percentage – temperature curves obtained at different heating rates. Methods for kinetic analyses include FWO, Kissenger, Coats – Redfern, Friedman, Freeman Carroll Metodu and van Krevelen Yöntemi methods. In our study, activation energies were calculated using differential (Kissenger method) and integral methods (FWO), using Equations (1) and (3), respectively.
2.8.1. Differential method
2.5.1.1. Kissenger method
The activation energy can be calculated using Equation (1) using the Kissenger method without knowing the solid-state degradation reaction mechanism.
β is heating rate, Tmax is temperature related to maximum reaction rate, A is pre-exponential factor, αmax is maximum degradation fraction, n is reaction order. Plotting ln (β/T [Citation1]max) versus (1000/Tmax) gives Ea activation energy from slope [Citation53].
2.8.2. Integral methods
2.8.2.1. Flynn–Wall–Ozawa (FWO) method
The FWO method is an integral method which can be used to determine the activation energy without knowledge of the reaction mechanism. The pre-exponential factor (A) and activation energy (Ea) do not depend on the degradation fraction but rather on the temperature. This method uses Equation (2) [Citation54,Citation55].
The Doyle approximation is then used and Equation (3) can be obtained.
The plot of log β versus 1000/T should be linear with the slope Ea/R, from which Ea can be obtained [Citation54,Citation55].
2.9. Cell culture
MCF-7 human breast cancer cell lines were purchased from American Type Culture Collection (ATCC, Rockville, Md., U.S.A). Cells were cultured as previously described [Citation56] in Eagle’s minimum essential medium containing 10% fetal bovine serum, 2 mM L-Glutamine, 100 U/mL penicillin, 0.1 mg/mL streptomycin, at 37°C in a humidified incubator with 5% CO2. The culture medium was changed every 2–3 days. Cells were passaged approximately every 5 days.
2.10. Cell viability test
Cell viability was determined using 3-(4,5-dimethylthiazol-2-yl)-2,5-diphenyltetrazolium bromide (MTT) assay [Citation57]. MCF-7 cell lines were seeded into 96-well plates at 2500 cells/well. After 24 h, the medium of the cells was replaced with a fresh medium. The synthesised glycopolymers were dissolved in DMSO at doses of 0, 1.2, 2.5, 5, 10, 20, 40 and 80 μg/mL. The cells were then treated with glycopolymer solutions for 24, 48 and 72 h. After the treatment period, 15 μL of the MTT solution at a concentration of 5 mg/mL was added to each well. Cells were incubated at 37 °C for 4 h. At the end of the incubation, the medium containing MTT was removed and 100 μL of DMSO was added to each well. Absorbance values were read using a microplate reader at a wavelength of 570 nm. The cell viability was calculated according to the formula as follows:
3. Results and discussion
Herein, radical copolymerisation of AG with MMA, AN and HEMA was carried out via reactions at 70 °C in DMF. Copolymer poly(AG-co_AN) was obtained in 10% yield, poly(AG-co-HEMA) in 7% yield and poly(AG-co-MMA) in 3% yield (yields as a ratio of the weight of the product to the total weight of the monomers) ().
Table 1. Copolymerisation of allyl glucosamine (AG) monomer with methyl methacrylate (MMA), acrylonitrile (AN) and 2-hydroxyethyl methacrylate (HEMA) in dimethyl formamide (DMF) under a nitrogen atmosphere.
There are several studies of MMA, AN and HEMA co-monomers synthesised by copolymerisation with different functionalised monomers [Citation58–60]. The conversions reported here for poly(AG-co-MMA), poly(AG-co-AN) and poly(AG-co-HEMA) are lower than those of copolymers reported using MMA, AN and HEMA comonomers with other N-allyl group monomer [Citation52]. The novel strategy proposed herein for preparing the N-allyl derivative comonomer at a mild temperature. Depending on the molecular structure of the comonomer synthesized, curing kinetics and final material properties can be customized.
3.1 Characterisation studies
3.1.1 FTIR spectra of the allyl glucosamine monomer and the synthesised glycopolymers
The FTIR spectra of the AG monomer and the synthesised copolymers are shown in . The FTIR spectrum of the AG monomer shows C – H in CH3 and C – H in CH2 at 2925 cm−1; vinyl C=CH2 at 1529 cm−1 and C – O–C peak in the sugar ring at 1028 cm−1. Lastly, the absorption bands at 1754 and 3266 cm−1 correspond to the NHC=O group (C=O and N – H, respectively).
Figure 1. TIR spectra of allyl glucosamine (AG) monomer and glycopolymers (poly(ag-co-MMA), poly(ag-co-AN) and poly(ag-co-HEMA)).

The FTIR spectra of the glycopolymers poly(AG-co-AN), poly(AG-co-MMA) and poly(AG-co-HEMA) () showed key absorptions as follows: C – H in CH3 and C – H in CH2 at 2800 - 3000 cm−1; C – O–C peak in the sugar ring at 1110 cm−1. Peaks observed at approximately 1710 and 1730 cm−1 are characteristic carbonyl vibrations of methacrylate groups. The intensity of the vinyl carbonyl peaks at ca.1640 cm−1 for the sugar methacrylate monomer was significantly reduced and shifted to a longer wavenumber after polymerisation, attributed to the absence of conjugation between C=O and C=CH2 linkages. The C=C peaks at 1452 cm−1 and characteristic absorption for the vinyl structure disappeared after polymerisation. The N-alloc group exhibited the characteristic C – H in CH3 and C – H in CH2 absorption bands at 2900–3100 cm−1. Furthermore, the expected hydroxyl peak of poly(AG-co-HEMA) is at approximately 3450 cm−1 in .
3.1.2 The 1H and 13C NMR spectra of the allyl glucosamine monomer and the synthesised glycopolymers
In the 1H NMR spectrum of the AG monomer, two doublets are observed at 6.26 ppm and 4.21 ppm for H-1 and H-2, respectively. For an ⍺-glucose derivative, H-1 and H-2 have a typical coupling constant of 3.6 Hz. The H-5 protons yield a multiplet centred at 3.92 ppm. One of the H-6 signals (H-6a) obtained is resolved into a doublet of doublets at 4.15 ppm with coupling constants J6a,5 of 10.3 Hz and J6a,6 of 0.8 Hz. The H-6b signal is observed at 4.21 ppm with JH6b-H5 of 5.7 Hz. Meanwhile, H-4 appears as a doublet of doublets at 4.35 ppm with J4,5 of 8.4 Hz. The H-3 signal is a multiplet at 4.07 ppm with an H-3 and H-4 coupling constant of 3.2 Hz. The NH proton appears as a broad signal singlet at 4.91 ppm. Moreover, the 12Hs of the four acetyl methyl groups are seen at 2.16 - 1.98 ppm. Finally, the alkene protons of the allylic group yield multiplet signals at 5.80 ppm and 5.21 ppm. Furthermore, the 13C NMR data of the AG monomer are also fully consistent.
In the 1H NMR spectra of the synthesised glycopolymers, the anomeric proton and other sugar protons are observed at 6.20 and 4.80 - 4.10 ppm, respectively. However, the disappearance of characteristic C=C peaks for the AG monomer can be observed in the 1H NMR spectra of the synthesised glycopolymers. These results show the successful synthesis of sugar-based monomers and their copolymers (the 1H NMR spectra of all glycopolymers are shown in ).
In the 13C NMR spectrum of AG, the acetyl group carbons (H3CC=O) are evident between 171.2 and 168.7 ppm. In addition, allylic carbon (–CH=CH2) is clear at 132.2 ppm and 118.1 ppm and amide carbonyl carbon (NHC=O) can be observed at 155.4 ppm. Meanwhile, the anomeric C-1 is observed at 91.8 ppm (). In the case of the 13C NMR spectra of the polymers and the alkyl group carbon peaks of the synthesised glycopolymers appear in the range of 40.4–21.6 ppm. The allyl carbons of the glycopolymers are shifted to higher fields because of increased electron density. The sugar moiety carbons of the glycopolymers are observed for C-1 to C-6 (the 13C NMR spectra of all the glycopolymers are shown in ).
3.2 Thermal studies
The TG of each synthesised glycopolymers and the AG monomer were measured in temperature range of 30–600°C at a rate of 10 °C/min under a nitrogen purge (100 mL/min) [Citation52,Citation53] and shown in .
Thermograms and DTGs () indicate that there is one decomposition stage for poly(AG-co-MMA), with degradation starting at 310°C and continuing up to 450°C. In contrast, poly(AG-co-AN) decomposes in two stages, beginning at 160°C and continuing at a slower rate to 280°C. The degradation percentage is approximately 25% and may be assigned to the oligomer. The second degradation started at 280°C and continued until 475°C. The second degradation may be assigned to poly(AG-co-AN) and the degradation percentage at this stage is approximately 35%. The maximum weight loss of the second product occurs at approximately 425°C. Poly(AG-co-HEMA) degradation began at 130°C and continued until 280°C, with approximately 45% mass loss. The second degradation began at 285°C and continued to approximately 370°C, resulting in an additional 7% degradation mass loss. The temperature for the maximum weight loss of the second product is approximately 320°C. The third decomposition stage mass loss is approximately 30% between 370°C and 500°C, yielding nearly 8% of residue even at 600°C.
Ea were calculated using differential (Kissinger) and integral (FWO) methods to obtain more information on the thermal stability of the synthesised glycopolymers. shows the dynamic TG plots of the glycopolymers under a nitrogen atmosphere at heating rates of 5, 10, 15 and 20 °C/min. As shown in , the thermal decompositions of the synthesised glycopolymers were not significantly affected by the heating rates.
Figure 11. TG curves of a) Poly(AG-co-MMA) b) Poly(AG-co-HEMA) c) Poly(AG-co-AN) at heating rates of 5, 10, 15, and 20 °C/min.; in nitrogen atmosphere.

summarise the Ea calculated using the Kissinger and FWO methods. The calculated decomposition Ea obtained from the Kissinger method are 166 kJ/mol for the poly(AG-co-MMA), 105 and 242 kJ/mol for the poly(AG-co-AN) and 129, 138 and 239 kJ/mol for poly(AG-co-HEMA). The mean values of Ea calculated by the FWO method were 186 kJ/mol for poly(AG-co-MMA), 110 and 212 kJ/mol for poly(AG-co-AN) and 70, 90 and 160 kJ/mol for poly(AG-co-HEMA). Ea obtained using FWO were smaller than those of the Kissinger method, most notably for poly(AG-co-HEMA), because the peaks on the DTG curves were not sharp enough to determine the peak point.
Table 2. Activation energies (Ea, kJ/mol) of poly(ag-co-MMA) obtained using the Flynn–Wall–Ozawa (FWO) and Kissinger methods.
Table 3. Activation energies (Ea, kJ/mol) of poly(ag-co-AN) obtained using the FWO and Kissinger methods.
Table 4. Activation energies (Ea, kJ/mol) of poly(ag-co-HEMA) obtained using the FWO and Kissinger methods.
3.3 Molecular weight study of the synthesised glycopolymers
The mass-average molecular weight (Mw), number-average molecular weight (Mn) and polydispersity index (PDI) for the synthesised copolymers were analysed by GPC and presented in .
Table 5. Number-average molecular weight (Mn), mass-average molecular weights (Mw) and polydispersity index (PDI) of synthesised glycopolymers determined using GPC.
These molecular weights are lower than those of homopolymers poly(MMA), poly(AN) and poly(HEMA) because of the resonance stability of the AG monomer in the double bond. This may be due to the degradative chain transfer to the monomer by forming an allylic radical, which weakens chain propagation. The molecular weights were also lower than those of typical vinyl compounds owing to the allylic radical resonance stabilisation, making the abstraction of a hydrogen atom more facile [Citation52,Citation61]. As a result, the allyl radical produced has a lower tendency to initiate a new polymer chain; hence, this chain transfer will lead to a termination reaction. This is ascribed to lower reactivity of the propagating radicals formed from these conjugated monomers [Citation61] the ester and nitrile substituents stabilize the radicals and decrease their reactivity toward transfer, and simultaneously the reactivity of the monomer toward propagation is enhanced. This resonance is called the degradative chain reaction. Therefore, the molecular weights of the obtained polymers are very low. Moreover, the PDI (Mw/Mn) of the synthesised glycopolymers are calculated as 1.678, 1.138 and 1.660, respectively (). These PDI values indicate that all the synthesised glycopolymers have homogeneous molecular weight distributions.
3.4 Biological evaluation
The cell viability effects of the new synthetic glycopolymers were evaluated using human breast cancer MCF-7 cell lines. The synthesised copolymers and the AG monomer were applied to MCF-7 cell lines in the same doses and for the same durations.
Poly(AG-co-MMA) had no significant effect on cell viability at 24, 48 and 72 h until 80 μg/mL (p > 0.05) (). Similar effects were observed with poly(AG-co-AN) (). However, poly(AG-co-HEMA) decreased cell viability after a dose of 10 μg/mL at 24 h (p < 0.05). It was determined that the highest dose at 72 h reduced cell viability 75% (). AG monomer at doses ranging 5–80 µg/mL, significantly decreased cell viability in a time- and dose-dependent manner (p < 0.05). At 80 μg/mL, the cell viability decreased by 95% and 98% at hours 48 and 72, respectively (). The new glycopolymers did not have a toxic effect of up to 72 h at all the treatment doses.
Figure 12. Effect of allylglucosamine monopolymer on cell viability in MCF-7 cell lines. Cell viability was determined by MTT analysis. Biopolymers were treated to MCF-7 cell lines at a dose of 0–80 μg/ml until 72 h. a). poly(ag-co-MMA), b). poly(ag-co-AN), c). poly(ag-co-AN) and d). monomer (AG). The results represent the average of three independent tests.Data are expressed as mean±standard deviation. (*p<0.05).

4. Conclusion
Three new carbohydrate-based glycopolymers (poly(AG-co-MMA), poly(AG-co-AN) and poly(AG-co-HEMA)) were synthesised from AG monomer via free-radical copolymerisation reactions using AIBN as an initiator in DMF. The copolymerisation yields indicated that AG leads to low conversions with the co-monomers MMA, HEMA and AN. The maximum copolymerisation yield was obtained using AG with AN, providing a glycopolymer with a 10% yield at 70 °C for 24 h. The thermal degradation of poly(AG-co-MMA), poly(AG-co-AN) and poly(AG-co-HEMA) in a nitrogen atmosphere proceeds in one-, two- and three-stage reactions, respectively. Among the AG-based glycopolymers synthesised, the HEMA-based glycopolymer (poly(AG-co-HEMA)) had the greatest effect on MCF-7 cell viability, noting that the monomeric carbohydrate itself in fact produces almost complete suppression fo cell-viability after 48 h or more. All the glycopolymers have low toxicity on MCF-7 cell lines.
Acknowledgments
This study was supported by Ege University’s Scientific Research Projects Coordination Unit with Project number FGA-2020-21474. Fatma Telli received a grant from the TUBITAK (Scientific and Technical Research Council of Turkey) BIDEB-2219 Programme for a postdoctoral fellowship.
Disclosure statement
No potential conflict of interest was reported by the authors.
References
- Varma AJ, Kennedy JF, Galgali P. Synthetic polymers functionalized by carbohydrates: a review. Carbohydr Polym. 2004;56:429–445.
- Telli FC. Synthesis, characterization and thermokinetic analysis of the novel sugar based styrene co-polymer. Polimeros. 2020;30:1–8.
- Vert M. Polymeric biomaterials: strategies of the past vs. strategies of the future. Prog Polym Sci. 2007;32:755–761.
- Pachence JM, Kohn J, In R, et al. Principles of tissue engineering. Academic Press/R G Landes Company. 1997;1:273–285.
- Louwrier A. Industrial products—the return to carbohydrate- based industries. Biotechnol Appl Biochem. 1998;27:1–8.
- Singh R, Bhattacharya B, Rhee HW, et al. Solid gellan gum polymer electrolyte for energy application. Int J Hydrog Energ. 2015;40:9365–9372.
- Methven JM. Polymeric materials from renewable resources. RAPRA Rev Rep. 1991;4:1–134.
- Borges MR, JAd S, Vieira M, et al. Polymerization of a water soluble glucose vinyl ester monomer with tensoactive properties synthesized by enzymatic catalyst. Mater Sci Eng C. 2009;29:519–523.
- Majeti NV, Kumar R. A review of chitin and chitosan applications. React Funct Polym. 2000;46:1–27.
- Dodane V, Vilivalam VD. Pharmaceutical applications of chitosan. Pharm Sci Technol. 1998;1:246–253.
- Carneiro MJ, Fernandes AC, Figueiredo CM, et al. Synthesis of carbohydrate based polymers. Carbohydr Polym. 2001;45:135–138.
- Neto CGT, Giacometti JA, Job AE, et al. Thermal analysis of chitosan based networks. Carbohydr Polym. 2005;62:97–103.
- Rinaudo M. Chitin and chitosan: properties and application. Prog Polym Sci. 2006;31:603–632.
- Zhang L, Liu W, Han B, et al. Synthesis and antitumor activity of arginine–glucosamine conjugate. Carbohydr Polym. 2007;69:644–650.
- Orgueira HA, Bartolozzi A, Schell P, et al. Modular synthesis of heparin oligosaccharides. Chemistry. 2003;9:140–169.
- Goyal N, Cheuk S, Wang G. Synthesis and characterization of d-glucosamine-derived low molecular weight gelators. Tetrahedron. 2010;66:5962–5971.
- Jiang ZH, Budzynski WA, Qiu D, et al. Monophosphoryl lipid a analogues with varying 3-O-substitution: synthesis and potent adjuvant activity. Carbohydr Res. 2007;342:784–796.
- Yamatani K, Kawatani R, Ajiro H. Synthesis of glucosamine derivative with double caffeic acid moieties at N- and 6-O-positions for developments of natural based materials. J Mol Struct. 2020;1206:127689–127695.
- Zhang J, Zhao M, Peng S. Synthesis of mimetic peptides containing glucosamine. Carbohydr Res. 2011;346:1997–2003.
- Lowe AB, Wang R. Synthesis of controlled-structure AB diblock copolymers of 3-O-methacryloyl-1,2: 3,4-di-O-isopropylidene-D-galactopyranose and 2-(dimethylamino) ethyl methacrylate. Polymer. 2007;48:2221–2230.
- Wulff G, Schmid J, Venhoff T. The synthesis of polymerizable vinyl sugars. Macromol Chem Phys. 1996;197:259–274.
- Black WAP, Dewar ET, Rutherford D. Polymerisation of unsaturated derivatives of 1,2: 5,6-di-O-isoprop ylidene-d-glucofuranose. J Chem Soc. 1963;1:4433–4439.
- Z-K X, Kou R-Q, Liu Z-M, et al. Incorporating α-allyl glucoside into polyacrylonitrile by water-phase precipitation copolymerization to reduce protein adsorption and cell adhesion. Macromolecules. 2003;36:2441–2447.
- Krasteva N, Harms U, Albrecht W, et al. Membranes for biohybrid liver support systems—investigations on hepatocyte attachment, morphology and growth. Biomaterials. 2002;23:2467–2478.
- Lin WC, Liu TY, Yang MC. Hemocompatibility of polyacrylonitrile dialysis membrane immobilized with chitosan and heparin conjugate. Biomaterials. 2004;25:1947–1957.
- Park CH, Nam SY, Lee YM, et al. Pervaporation of pyridine–water mixture through poly(acrylonitrile-co-monoacryloxyethyl phosphate) membrane. J Membr Sci. 2000;164:121–128.
- Kimura S, Hirai K. Vinyl polymerization LIX. On the syntheses of polymethacrylates of D-glucose and L-sorbose. Makromol Chem. 1962;58:232–236.
- Muthukrishnan S, Mori H, Müller AHE. Synthesis and characterization of methacrylate-type hyperbranched glycopolymers via self-condensing atom transfer radical copolymerization. Macromolecules. 2005;38: 3108:3119.
- Kimura S, Imoto M. Synthesis of polymethacryloyl-D-glucose and its copolymers with acrylonitrile. Vinyl polymerization LIV. Makromol Chem. 1961;50:155–160.
- Patil DR, Dordick JS, Rethwisch DG. Chemoenzymatic synthesis of novel sucrose-containing polymers. Macromolecules. 1991;24:3462–3463.
- Ohno K, Tsujii Y, Fukuda T. Synthesis of a well‐defined glycopolymer by atom transfer radical polymerization. J Polym Sci A Polym Chem. 1998;36:2473–2481.
- Stubbs KA, Macauley MS, Vocadlo DJ. A highly concise preparation of O-deacetylated arylthioglycosides of N-acetyl-D-glucosamine from 2-acetamido-3,4,6-tri-O-acetyl-2-deoxy-α-D-glucopyranosyl chloride and aryl thiols or disulfides. Carbohydr Res. 2006;341:1764–1769.
- Saltan F. Preparation of poly(eugenol-co-methyl methacrylate)/polypropylene blend by creative route approach: structural and thermal characterization. Iran Polym J. 2021;30:1227–1236.
- Arcos-Hernandez M, Naidjonoka P, Butler SJ, et al. Thermoresponsive glycopolymers based on enzymatically synthesized oligo-β-mannosyl ethyl methacrylates and N-Isopropylacrylamide. Biomacromolecules. 2021;22:2338–2351.
- Godjevargova T. Behavior of glucose oxidase immobilized on ultrafiltration membranes obtained by copolymerizing acrylonitrile and N-vinylimidazol. J Membr Sci. 2000;172(1–2):279–285.
- Miura Y. Design and synthesis of well-defined glycopolymers for the control of biological functionalities. Polym J. 2012;44:679–689.
- Narain R, Jhurry D, Wulff G. Synthesis and characterization of polymers containing linear sugar moieties as side groups. Eur Polym J. 2002;38:273–280.
- Slavin S, Burns J, Haddleton DM, et al. Synthesis of glycopolymers via click reactions. Eur Polym J. 2011;47:435–446.
- Yadav P, Yadav H, Shah VG, et al. Biomedical biopolymers, their origin and evolution in biomedical sciences: a systematic review. J Clin Diagn Res. 2015;9:21–25.
- Song R, Murphy M, Li C, et al. Current development of biodegradable polymeric materials for biomedical applications. Drug Des Devel Ther. 2018;12:3117–3145.
- Udenni Gunathilake TMS, Ching YC, Ching KY, et al. Biomedical and microbiological applications of bio-based porous materials: a review. Polymers. 2017;9:160–176.
- Jun S-K, Cha J-R, Knowles JC, et al. Development of Bis-GMA-free biopolymer to avoid estrogenicity. Dent Mater. 2020;36:157–166.
- Walker L, Perkins E, Kratz F, et al. Cell penetrating peptides fused to a thermally targeted biopolymer drug carrier improve the delivery and antitumor efficacy of an acid-sensitive doxorubicin derivative Int. J Pharm. 2012;436:825–832.
- Gayathri S, Ghosh OSN, Viswanath AK, et al. Synthesis of YF3: yb, Er upconverting nanofluorophores using chitosan and their cytotoxicity in MCF-7 cells. Int j biol macromol. 2015;72:1308–1312.
- Ali U, Kjba K, Na B. A review of the properties and applications of poly (methyl methacrylate) (PMMA). Polym Rev. 2015;55:678–705.
- Bettencourt A, Almeida AJ. Poly(methyl methacrylate) particulate carriers in drug delivery. J Microencapsul. 2012;29:353–367.
- Kejlová K, Labský J, Jírová D, et al. Hydrophilic polymers-biocompatibility testing in vitro. Toxicol Vitro. 2005;19:957–962.
- Pramudya I, Chung H. Recent progress of glycopolymer synthesis for biomedical applications. Biomater Sci. 2019;7:4848–4872.
- Boullanger P, Banoub J, Descotes G. N-Allyloxycarbonyl derivatives of D-glucosamine as promotors of 1,2-trans-glucosylation in Koenigs–Knorr reactions and in Lewis acid catalyzed condensations. Can J Chem. 1987;65:1343–1348.
- Wang D, Das A, Leuteritz A, et al. Thermal degradation behaviors of a novel nanocomposite based on polypropylene and Co–Al layered double hydroxide. Polym Degrad Stab. 2011;96:285–290.
- Wang H, Yang J, Long S, et al. Studies on the thermal degradation of poly(phenylene sulfide sulfone). Polym Degrad Stab. 2004;83:229–235.
- Yildirim Y, Balcan M. Comparative copolymerization of allyl glycidyl ether with styrene using radiation and chemical initiation methods. Iran Polym J. 2013;22:1–7.
- Kissinger HE. Reaction kinetics in differential thermal analysis. Anal Chem. 1957;29:1702–1706.
- Flynn JH, Wall LA. A quick direct method for the determination of activation energy from thermogravimetric data. J Polym Sci, Part B: Polym Phys. 1966;4:323–328.
- Ozawa T. A new method of analyzing thermogravimetric data. Bull Chem Soc Jpn. 1965;38:1881–1886.
- Akter R, Hossain MZ, Kleve MG, et al. Wortmannin induces MCF-7 breast cancer cell death via the apoptotic pathway, involving chromatin condensation, generation of reactive oxygen species, and membrane blebbing. Breast Cancer. 2012;4:103–113.
- Mosmann T. Rapid colorimetric assay for cellular growth and survival: application to proliferation and cytotoxicity assays. J Immunol L Methods. 1983;165:55–63.
- Erol I, Guldiken A. Synthesis of novel functionalized methacrylate copolymers and their copolymerization kinetics, thermal stability, and biocidal properties. J Appl Polym Sci. 2021;138:51334–51348.
- Szanka A, Szarka G, Ivan B. Poly(methyl methacrylate-co-2-hydroxyethyl methacrylate) four-arm star functional copolymers by Quasiliving ATRP: equivalent synthetic routes by protected and nonprotected HEMA comonomers. J Macromol Sci A. 2014;51:125–133.
- Toms RV, Balashov MS, Shaova AA, et al. Copolymers of acrylonitrile and acrylic acid: effect of composition and distribution of chain units on the thermal behavior of copolymers. J Polym Sci B Polym Phys. 2020;62:102–115.
- Matsumoto A. Polymerization of multiallyl monomers. Prog Polym Sci. 2001;26:189–257.